Abstract
This review aims to elucidate the current knowledge and future research needs regarding the hazard potential of nanocellulose to human health. Growing interest from research and industry alike has led to increasing likelihood of human contact to the material via various exposure routes. Although a number of comprehensive reviews on human health hazards of nanocellulose have been conducted, this paper brings new insights as it systematically analyzes and quantitatively assesses the results of in vivo and in vitro tests in terms of investigated endpoints, tested concentration ranges, physicochemical properties, surface modifications and source of the tested nanocellulose, exposure route, and cell lines used. The quality of the studies is further inspected based on various established criteria. Considering the rapid development of nanocellulose-based products and the novelty of the material, human health studies remain scarce. By assessing those that have been conducted, patterns and gaps were identified that will be helpful to guide future research. The results show that there are still significant uncertainties remaining, particularly regarding in vivo testing, with pulmonary exposure showing some cause for concern. Although a substantial number of in vitro studies have been undertaken, results are often conflicting. The detected effects could not be directly attributed to size of nanoparticles, cell lines, surface modifications or tested concentrations. This may also be linked to the varying quality of the studies. This review ends by identifying key gaps to help pave the way for future research and ensure the safe development and use of nanocellulose.
Introduction
With increasing use of nanocellulose (NC) in a wide range of industries and applications comes an increased likelihood of human exposure to this material during the various life stages of NC-containing products (Gottschalk and Nowack Citation2011). NC shows promise within a multitude of application fields, from healthcare to construction (Piccinno et al. Citation2016; Stoudmann, Nowack, and Som Citation2019). NC can come from many different sources, ranging from wood pulp and cotton to food waste and algae. The two main types of NC are cellulose nanofibers (CNF) and cellulose nanocrystals (CNC), which differ in terms of production process, morphology, and functions (Blanco et al. Citation2018). CNC generally display higher stiffness than CNF, and are produced through acid hydrolysis, as opposed to mechanical grinding or high-pressure homogenization in the case of CNF. The dimensions of CNC depend on the initial cellulosic material and hydrolysis process used, but are generally between 10 and 50 nm in width and 1–5 µm in length (Usov et al. Citation2015). CNF are flexible fibers ranging from 3–100 nm in width and more than 1 µm in length (Dufresne Citation2017), even reaching critical fiber lengths of more than 5 µm and often forming web-like structures. Pretreatments (e.g. 2,2,6,6-tetramethylpiperidine-1-oxyl radical (TEMPO) oxidation, carboxymethylation, enzymatic treatments…) are frequently used either before or after the extraction process of NC to enhance the final material properties (Jonoobi et al. Citation2015). Currently, CNF is by far the most produced and utilized, although there has been a shift to production of CNC at industrial scale in recent years (Future Markets Inc Citation2015).
Recommendations regarding the Occupational Exposure Limits Value (OEL) of NC via inhalation or dermal exposure are limited due to the small amount of data available regarding the hazard of this material. Following the precautionary principle, the OEL value of 0.01 fibers cm−3 (the same as for carbon nanofibres) has been suggested due to the potential biopersistence of NC when inhaled (Stockmann-Juvala, Taxell, and Santonen Citation2014).
A number of review studies on NC have shown that toxicity studies remain scarce and knowledge gaps remain. Furthermore, certain studies have shown diverging results. A screening-level life cycle risk assessment of NC (CNC and CNF) conducted by evaluating its toxicity according to various exposure scenarios pointed out that studies on long-term exposures and with realistic concentrations are currently lacking, as well as studies on certain common human health endpoints such as carcinogenicity, neurotoxicity and reproductive effects (Shatkin and Kim Citation2015). These data gaps therefore inhibit a clear conclusion regarding the human health impacts of NC to be made. A review of the toxicity of CNC found that although the small number of in vivo studies on oral and dermal toxicity showed no signs of adverse effects, the results from both pulmonary (inhalation in vivo) and cytotoxicity studies showed inconsistent results (Roman Citation2015). However, considering the differences in cell lines, cellulose source, and testing conditions, these results were not surprising. The author calls for more studies to be conducted to elicit clearer conclusions, as well as highlighting the importance of analyzing samples for contaminants. Another review also focusing on the toxicity of CNC, but specifically used as carriers in medicine, stressed the crucial role that the preparation–including the various possible chemical modifications of these versatile materials–plays in determining their toxicity (Seabra et al. Citation2018). An extensive review of the current knowledge of the impacts of NC on human and environmental health showed that realistic doses and exposure scenarios seem to suggest a low toxicity potential (Endes et al. Citation2016). However, certain specific physicochemical characteristics appear to lead to increased toxicity, although the structure-activity relationship is currently not well understood. The authors identified wide divergences between study findings, and point out the current lack of chronic, low dose, and repeated exposure studies. These variations in study findings were attributed to a wide range of factors including variation in exposure doses, test model, cellulose source, pretreatment methods, exposure scenarios, or incomplete material characterization. However, the authors stressed that heightened toxicity cannot be attributed to any single physicochemical property. One review also points out that the low number of peer-reviewed studies and of long-term exposure studies of NC seems to be a big issue (Lie, Ålander, and Lindström Citation2017). From the results of the few existing in vivo studies, inhalation of the material may be a potential threat, and the authors recommend prudence when this exposure route is a possibility. Recently, a review of the knowledge surrounding risk of NC through inhalation exposure, as well as an assessment of the quality of the studies relating to their usefulness for risk assessments showed some positive results (Ede et al. Citation2019). Using two sets of criteria developed by Krug and Wick (Citation2011) and Card and Magnuson (Citation2010) to assess the quality of nanotoxicity studies, the authors found that although the overall quality was currently inadequate for the results to be used for risk assessments, studies were improving over time. This inadequacy was due to a number of factors, including but not limited to inadequate characterization, unrealistic doses, lack of long-term testing, and lack of controls. The authors conclude that short-term exposure via inhalation of CNF has similar effects as poorly-soluble low toxicity (PSLT) particles, and that neither CNC nor CNF appear to conform to the fiber paradigm. However, long-term, low dose exposure studies were again identified as a major data gap.
Most recently, Čolić, Tomić, and Bekić (Citation2020) reviewed knowledge on the immune system’s response to nanocellulose, with particular focus on macrophages and dendritic cells. The study shows that through surface modifications, the pro-inflammatory response of NC can be reduced and even become anti-inflammatory. The authors highlight the value this may hold to medical applications of NC, such as wound healing, as well as the need for further immunological studies and 3D model systems in vitro.
The overarching conclusions of these reviews are that uncertainties are still present, preventing a straightforward judgment to be made regarding health and safety issues surrounding NC. However, from these reviews it is difficult to judge what exactly has or has not been assessed. The aim of this paper is to clarify the current state of knowledge on the potential hazard of NC. By doing so, it will be easier to target research needs of toxicological studies with adequate quality and to understand the current state of knowledge for decision-making in material and product innovation. Through the quantification of what has been done in the literature in terms of endpoints, material characteristics, and testing models, we are able to map existing knowledge gaps. To do so, data from various toxicity endpoints, cell lines, concentration ranges and sizes of tested NC was systematically gathered and quantitatively analyzed.
Literature review
Using the Web of Science database in February 2019, we identified 60 studies with the keywords: Nanocellulose OR ‘Cellulose nano*’ AND Toxicity OR Genotoxicity OR Inflammation OR ‘Oxidative stress’ OR ‘Reproduct*’ OR ‘in vivo.’ From these, 38 were selected as relevant research studies, i.e. peer reviewed studies that investigated the human health effects of NC. We collected information regarding the physicochemical characteristics of the investigated nanomaterials, cellulose source, surface modification, the employed testing methods and models, the exposure routes when applicable, and the tested dose/concentrations ranges.
In order to have an overview of the quality of the identified studies, we selected 8 criteria from the Literature Criteria Checklist developed within the Data and knowledge on Nanomaterials (DaNa) project (DaNa Citation2016), as well as crystallinity of CNC. From the Literature Criteria Checklist, only the criteria judged as most relevant for the intended purpose of this paper and the nanomaterial at hand were selected (Schmutz et al. Citation2017); our aim was to gain an overall view of the quality of the existing studies ().
Table 1. Share of studies fulfilling certain quality criteria selected or adapted from the Literature Criteria Checklist developed in the Data and knowledge on Nanomaterials (DaNa) project. Number of studies: n = 38.
Although all studies reported on concentration and most studies on particle length (90% of studies), shape (87%), test medium (90%), negative controls (90%), and positive control (64%), other criteria were far less frequently discussed. Impurities, crystallinity of CNC, and check for interferences were reported for less than half of the studies at hand. The two most extensive and comprehensive studies reported 9 of the 11 selected criteria (Ong et al. Citation2017; Ilves et al. Citation2018), and the median was 6 out of 11 criteria (see Supplementary material Table S1).
Overview of nanocellulose hazard potential
In order to provide a quantitative visual overview of the current state of knowledge regarding the hazard potential of NC and to identify trends or inconsistencies in study findings and potential gaps, the 38 studies were analyzed by investigating what endpoints have been looked at. summarizes the hazard potential of materials tested in vivo and in vitro according to various toxicity endpoints. These endpoints were selected as they are the ones needed to assess human health risks (Jesus et al. Citation2019). To be as detailed as possible, each count in represents a single material (i.e. with a specific physicochemical property). Studies can therefore find themselves in more than one box, if multiple materials or endpoints were investigated. In the Supplementary material, Figure S1 details the number of studies having looked at each of these endpoints, and the detailed list of the 38 reviewed studies can be found in Supplementary Table S2. In the next sections, we look in further detail at the hazards described in .
Figure 1. Systematic review of toxicity results of CNC and CNF. The length of the bars refers to the number of investigated materials (i.e. each with specific physicochemical properties) per endpoint, represented in terms of observed effect. As certain studies looked at multiple materials, a similar table relating to the number of studies having been conducted per endpoint can be found in the Supplementary material Figure S1.

In vivo research
While the majority of studies are in vitro, few in vivo studies have been performed. Three studies were identified that conducted in vivo acute testing of CNC via pulmonary exposure. The first, looking at the pulmonary toxicity of two CNC materials (in gel form and as a powder) derived from wood pulp and administered by pharyngeal aspiration to mice showed significant variations in oxidative stress, tissue damage, and inflammatory response depending on the material 24 h after exposure (Yanamala et al. Citation2014). Both tested materials were found to cause a more severe acute inflammatory response in lungs than asbestos (concentration range of 50–200 µg/mouse), with the CNC gel exhibiting the greatest effect on the test mice. Pulmonary damage, measured by increased LDH activity in BAL, was also observed for both materials. On the other hand, in the second study, no inflammatory response nor pulmonary damage was observed while testing both CNC and CNF, also administered by pharyngeal aspiration, at concentrations of 40 and 80 µg/mouse (Park et al. Citation2018). Although the materials persisted in the lungs 14 days post-exposure and showed signs of inducing an inflammatory response, neither of the tested materials behaved as ‘asbestos-like’ materials. The third study, using a wood-derived CNC, looked at acute inhalation toxicity in rodents and found the LD50 to be greater than 26 µg/L, as well as at genotoxicity by performing an in vivo erythrocyte micronucleus test on mice, where no adverse effects were observed (O’Connor, Berry, and Goguen Citation2014).
Eight materials from four studies were identified regarding pulmonary exposure of CNF, of which the study by Park et al. (Citation2018) mentioned above where no adverse effects were observed. The studies by Hadrup et al. (Citation2019) and Ilves et al. (Citation2018) show consistencies and share overlaps. With regard to the investigated materials, both looked at non-functionalized and carboxylated CNF from wood-based pulp. However, Hadrup et al. (Citation2019) reported greater inflammation than the study by Ilves et al. (Citation2018) for the same materials. Hadrup et al. (Citation2019) found that testing the pulmonary effects of two CNF materials (non-functionalized and carboxylated) administered by intratracheal instillation in mice elicited an inflammatory and acute phase response, leading the lowest tested dose (0.3 mg/kg by bodyweight) to be suggested as lowest observed adverse effect level (LOAEL) value for the tested materials. While no significant genotoxicity was observed for the non-functionalized CNF, the carboxylated material induced increased DNA damage in BAL. The authors further demonstrated pulmonary presence of both materials 28 days post-exposure. Ilves et al. (Citation2018) looked at four CNF materials (two non-functionalized, carboxymethylated and carboxylated) administered via oropharyngeal aspiration, and found that they persisted in the mice for at least a month. However, the effects were significantly less severe than those due to rigid multi-walled carbon nanotubes (CNT), to which the results were compared. The authors also found significant influx of neutrophils into BAL after 24 h for all materials. While Hadrup et al. (Citation2019) used intratracheal instillation, Ilves et al. (Citation2018) used aspiration, put forward as an explanation to the observed differences in inflammatory response between the studies. The viscosity of CNF means that a larger fraction of material may be blown into the lung and lower airways by intratracheal instillation than with aspiration, where the material is dosed on the back of the tongue and where the inhalation itself drags the material down the lung. Both studies observed significant toxicity at all tested concentrations (6 and 18 μg/mouse, and 10 and 40 µg/mouse, respectively), and observed that carboxylation lowered toxicity. Lastly regarding pulmonary exposure, Catalán et al. (Citation2017) found dose depended genotoxicity looking at CNF from spruce sulfite dissolving pulp administered by pharyngeal aspiration, with a significant increase in percentage of DNA tail in murine lung cells at the two lowest doses (range 10–200 µg/mouse). The authors further observed an acute inflammatory response 24 h post exposure, as well as a dose-dependent accumulation of CNF in the bronchi and alveoli.
Two studies were identified regarding repeated-dose via pulmonary exposure. Shvedova et al. (Citation2015) found that CNC administered to male and female mice by pharyngeal aspiration twice a week over three weeks led to chronic pulmonary inflammation and damage, oxidative stress, and abnormal histology of conductive and respiratory airways. The second study, by Farcas et al. (Citation2017), looked at the effect of long-term exposure of CNC on the reproductive system of mice. Three months after exposure via aspiration to a cumulative dose of 240 µg/mouse administered over three weeks (40 µg/mouse/day), results showed DNA damage to sperm, testicular and epididymal oxidative stress, pathologic abnormalities of testes and inflammatory response, and significant reproductive toxicity. No repeated-dose studies via pulmonary exposure were identified for CNF.
The materials having been tested with regard to exposure via ingestion, dermal contact, and ocular contact come from two studies. O’Connor, Berry, and Goguen (Citation2014) looked at acute and repeated dose oral toxicity as well as skin sensitization of CNC. The authors found no significant effect for any tested concentrations and endpoints. The NOEL was found to be greater than 2000 mg/kg bodyweight/day for the repeated oral dose, and as no effect was observed from the single dose ingestion, the authors hypothesize that the LD50 is also greater than 2000 mg/kg bodyweight/day. Ong et al. (Citation2017) also looked at acute oral toxicity and skin irritation, as well as eye irritation after exposure to lignin-coated CNC and CNF. The authors did not observe any significant effects for any of the endpoints and materials. Although the three endpoints from these studies did not show signs of toxicity, firm conclusions cannot be made considering this small study number. The same can be said regarding other in vivo endpoints, with very few studies having looked at dermal and ocular contact and injection.
In vitro research
In this section we look in more detail at the results of the in vitro endpoints and the reasons why: certain materials may have exhibited a significant effect while others did not for the same endpoint. This is the case for cytotoxicity, oxidative stress, inflammation of both CNC and CNF, as well as for genotoxicity of CNF (). However, this information deserves further analysis as the results are dependent on the model system, concentration ranges tested, assay duration and physicochemical properties of the materials (Jesus et al. Citation2019). Below, we present these diverging studies in more detail, specifically focusing on genotoxicity, oxidative stress and inflammation. The cytotoxicity results will be analyzed in more depth later on.
Genotoxicity
Regarding CNC, two studies looking at genotoxicity were identified, both having found no significant effects. Looking at cotton-derived CNC, Catalán et al. (Citation2015) found no significant genotoxicity in human bronchial epithelial cells using the micronucleus assay to assess chromosomal damage at doses ranging from 2.5 to 100 µg/mL. In the second study, O’Connor, Berry, and Goguen (Citation2014) observed no signs of genotoxicity using the in vitro mammalian chromosome aberration test in cultured Chinese hamster ovary cell lines (800, 2000, and 5000 µg/mL).
The ambiguous results from the genotoxicity endpoint regarding CNF warrant further investigation, as this would give information regarding long-term risks of NC. Three studies relating to in vitro genotoxicity of CNF were identified. Results from the study by Pitkänen et al. (Citation2014) showed no genotoxic effect of CNF on Salmonella typhimurium using the bacterial reversion assay at concentration ranges of 19–300 µg/mL, and controls performing as expected. However, the authors point out that follow up genotoxicity testing to this first screening is needed for the studied materials. Looking at five CNF materials from various sources, de Lima et al. (Citation2012) found two of them (brown cotton and curaua (Ananas erectifolius)) to be significantly genotoxic at all tested concentrations (0.01, 0.1 and 1%) in lymphocytes, while the other three (white, ruby, and green cotton) were not, using the Comet assay. The study also looked at plant cells using the Allium cepa assay, with results showing greater genotoxic effect to be tied to green, white and brown cotton and curaua. Ruby cotton was the only CNF source that did not induce any significant DNA breaks in either cell types. Similarly, Ventura et al. (Citation2018) assessed genotoxicity using the Comet and Micronucleus assays in a co-culture of A549 cells (adenocarcinomic human alveolar basal epithelial) and THP-1 macrophages. A genotoxic effect was observed from the Comet assay results at the highest tested concentration (range 1.5–25 µg/cm2), although DNA damage was low, as was the case for de Lima et al. (Citation2012). The micronucleus assay showed a significant increase in the frequency of micro nucleated binucleated cells at the two lowest concentrations, warranting further investigation. Parameters explaining these varying results could be for example the physicochemical properties of each of the tested materials. However, tested concentrations did not appear to be a good indicator of whether genotoxicity was or was not observed (see Supplementary material Table S2 for tested concentrations).
Oxidative stress
Diverging results are also apparent with regard to oxidative stress and inflammation. Two CNC materials tested on A549 cells, varying in terms of cellulose source (cotton and tunicates) and physicochemical properties (aspect ratio, zeta potential, stiffness, surface charge), showed no signs of oxidative stress through the assessment of GHS levels (Endes et al. Citation2014). Menas et al. (Citation2017) tested multiple CNC and CNF materials varying in terms of their synthesis and physicochemical properties (length, structure, polydispersity index), also on A549 cells. All materials except a CNC produced by acid hydrolysis exhibited signs of oxidative stress and inflammation after 72 h, through the assessment of GHS and SH levels and release of cytokines/chemokines, respectively. The study concludes that predicting NC toxicity requires a concerted approach where physicochemical properties are known and target endpoints selected to allow for correlating physico-chemical properties to biological outcomes, going beyond classical assays. Looking at CNF from spruce, Čolić et al. (Citation2015) did not observe signs of oxidative stress in murine fibroblasts at any of the six tested concentrations (31.25 µg/mL-1000µg/mL).
Inflammation
As for oxidative stress, Endes et al. (Citation2015) did not observe any signs of inflammatory response at any of the tested concentrations of the two CNC materials using a 3D multi-cellular model of the human epithelial airway barrier at the air liquid interface. Neither did Catalán et al. (Citation2015) when looking at CNC from cotton in human monocyte-derived macrophages. Menas et al. (Citation2017) however did find that CNC induced a significant inflammatory response in A549 cells, greater than the two CNF materials also investigated in the study. Also using A549 cells as test systems, Ventura et al. (Citation2018) similarly did not observe any inflammatory response tied to TEMPO-mediated CNF. In a study interested in safety surrounding friction grinding and spray drying of CNF, no inflammatory effects on human peripheral blood mononuclear cells (PBMCs) and murine macrophages (RAW 264.7) were observed (Vartiainen et al. Citation2011). CNF is also receiving a lot of interest from the medical field. A wood-derived CNF was found to be attractive for biomedical applications as it did not induce an inflammatory response in peripheral blood mononuclear cells (Čolić et al. Citation2015). Another study found TEMPO-mediated CNF to show promise as wound dressing material, with no observed increased secretion of inflammatory cytokines in human epidermal keratinocytes (Nordli et al. Citation2016).
A number of comparative studies have been conducted regarding the inflammatory effect of CNF. One study looking at the effects of CNF derived from cotton, CNT, and crocidolite asbestos fibers (CAFs) on a human 3D lung cell coculture model showed that although CNF did lead to a dose dependent toxicity and induce an inflammatory response, these were significantly lower than those seen by CNT and CAFs (Clift et al. Citation2011). The authors highlight the issues tied to the use of monocultures for hazard screening. The study by Lopes et al. (Citation2017) found that although a pro-inflammatory response was observed for an unmodified CNF on human monocytic cells, this effect was not apparent from the two modified materials (carboxymethylated, and 2,3-epoxypropyl trimethyl ammonium chloride (EPTMAC) quaternized).The authors concluded that inflammation may be driven by the surface chemistry of the materials. A similar conclusion was reached regarding the study by Ilves et al. (Citation2018) looking at four CNF materials, with the two non-surface modified materials showing greater inflammatory potential compared to the other three materials (carboxymethylated and carboxylated). An inflammatory response was observed on human monocytic cells (THP-1) for the non-functionalized materials, and milder signs for the two modified CNFs. The authors hypothesize that the difference in results between the two unmodified CNFs could be due to the neutral zeta potential of the material showing no negative effects, potentially causing less inflammatory response. Lastly, in an extensive study performing cytokine profiling of 19 engineered nanomaterials (ENMs), the four investigated CNF materials were found to be nontoxic at concentrations up to 100 µg/mL (Bhattacharya et al. Citation2017). However, all four induced an inflammatory response at sub-cytotoxic doses.
This overview highlights the complexity and ambiguity surrounding results from the literature when considering them as a whole. The small number of studies is clearly an issue, preventing any straightforward conclusions to be made at this point. In the next section, we try to further disentangle the studies to gain further insights into what has been done and still needs doing.
Fiber paradigm
In order to deepen the analysis, the lengths of the NC materials were compiled to see whether this material property could be linked to cytotoxicity (). Cytotoxicity was selected as it is the endpoint having the largest number of data points.
Figure 2. Observed cytotoxicity effect as a function of length of 42 tested CNC materials and 18 CNF. The dashed line at 5 µm in the CNF plot refers to the lower length threshold for materials to fit the fiber paradigm (Donaldson et al. Citation2010).

Due to the high aspect ratio of many NC materials, there have been discussions about the possibility of the fiber paradigm coming into play. Length, rigidity, dose and biopersistence determine a fiber’s pathogenicity. Phagocytosis or encapsulation of fibers by macrophages is not possible when fibers are longer than about 10 µm and show relatively high stiffness (Donaldson et al. Citation2010). From we see that length alone is not an accurate predictor of toxicity, as certain long CNFs showed no signs of toxicity whereas other longer ones did, and vice versa. While CNFs are flexible and often intertwined, CNCs are more rigid and rod-like, with a Young’s modulus ranging between 50 and 143 GPa (Hoeng, Denneulin, and Bras Citation2016, Voisin et al. Citation2017). In comparison, the Young’s modulus of single walled carbon nanotubes (SWCNTs) range between 320 and 1470 GPa, and multi-walled carbon nanotubes (MWCNTs) between 270 and 950 GPa (Voisin et al. Citation2017). The biopersistence of NC is still unclear, but it is likely that these particles reside for long periods of time, similarly to bulk cellulose (Shatkin and Kim Citation2015). Ilves et al. (Citation2018) and Hadrup et al. (Citation2019) both found that CNFs were still present in the lungs of mice one month post-exposure via oropharyngeal aspiration and intratracheal instillation, respectively. Park et al. (Citation2018) also found that both CNC and CNF were still present in the lungs 14 days post-exposure, also via oropharyngeal aspiration.
In the collated studies of this paper, no CNC materials were above the fiber paradigm's length threshold of >5 µm (Donaldson et al. Citation2010). Nevertheless, the possibility remains that certain fibers do meet this criterion. Considering the rigid nature of these materials, there is therefore a need for further studies looking into the possibility of CNC and the fiber paradigm (Camarero-Espinosa et al. Citation2016). Based on , in vivo studies via pulmonary exposure should be a focus, as certain studies observed significant effects. Thorough analysis of material properties in correlation to specific lung injuries is also advisable.
Ranges of tested concentrations and their toxicity effect
In and , we sorted the collated studies looking at cytotoxicity and inflammation in vitro (the two most reported in vitro endpoints) based on maximum tested concentrations. We did not do so for the six CNC materials under in vitro inflammation (), as the material and methods sections of the respective papers did not allow for comparability due to the units used (e.g. µg/cm2, µg/mL). It should be noted that all tested cell lines and assays were pooled together in creating these graphs. Certain cell lines are likely more sensitive than others, as well as there being differences in the physicochemical properties of the materials under consideration. These graphs should therefore be taken as an overview of what has been done, and not be overly interpreted.
Figure 3. Number of materials studied for cytotoxicity with effect/no effect as affected by maximum tested concentrations. For example, four CNC materials having shown an effect have been tested in concentrations ranging from 0 to 249 µg/mL, the highest tested concentration for these studies. For comparative reasons, studies reporting concentrations in µg/cm2 were not included.
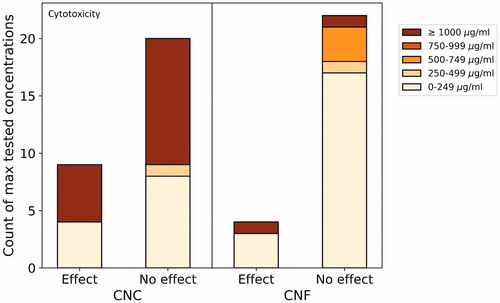
Figure 4. Number of CNF materials studied for inflammatory response in vitro with effect/no effect as affected by maximum tested concentrations. For comparative reasons, studies reporting concentrations in µg/cm2 were not included.
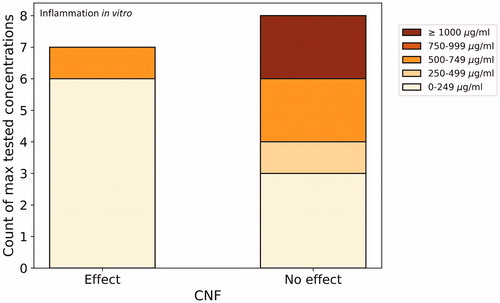
Four CNC materials having caused significant cytotoxic effects () were from a study looking at the effect of surface coatings of CNC on mammalian cell lines (mouse macrophages (J774A.1) and human breast adenocarcinoma cells (MCF-7) (Jimenez et al. Citation2017). Five materials were tested by MTT and Lactate Dehydrogenase (LDH) assays, including four CNCs with coatings (amino functionalized, β-cyclodextrin coating, poly(N-isopropylacrylamide) (NIPAAm) coating, and poly(N-3-aminopropyl) methacrylamide (APMA) coating). At 24 h only the two highest concentrations induced a marginal effect, but only in J774A.1 cells and only three of the materials (poly(NIPAAm), amino functionalized, and poly(APMA)). In MCF-7 cells, two of the materials induced an increase in cell viability at higher concentrations. However, these effects did not seem to be dose-dependent. After 48 h, all materials induced a marginal reduction in cell viability in J774A.1 cells, not dose-dependent. However, only amino functionalized CNCs induced cytotoxicity in MCF-7 cells, although also not dose-dependent. All materials induced a marginal dose-dependent LDH release in J774A.1 cells. There did not seem to be a correlation between these observed time-dependent effects and anionic or cationic surface coatings. The authors conclude that there is a need for long-term studies looking at the effect of functionalization on immunogenicity and cytotoxicity. Hanif et al. (Citation2014) did not observe significant cytotoxic effect from four CNC materials at concentrations below 250 µg/mL, with a maximum tested concentration of 1000 µg/mL. Many CNC materials were tested at high concentrations without an effect being observed, while fewer studies tested very high concentrations (≥1000 µg/mL) for CNF. Cytotoxic effects were nevertheless observed in certain studies looking at CNF materials. In one, CNF showed a slight cytotoxic effect on human cervical cancer cells (HeLa229) at 24 h at the highest concentration tested (240 µg/mL) but no genotoxic effects (Pitkänen et al. Citation2014). Cytotoxicity was assessed by highest tolerated dose (HTD) and total protein content (TPC), both at 24 h and 72 h. In another study having found a toxic effect (Clift et al. Citation2011), the authors used the LDH assay and a 3D human lung cell coculture and a concentration range of 5–30 µg/mL. Cytotoxic effects were also elicited by CNF in a study at concentrations between 200 and 5000 µg/mL (total range: 0.02–5000 µg/mL), observed through flow cytometer assay on bovine fibroblasts (Pereira et al. Citation2013). It should be noted that this dose range is very high and unlikely to occur in an occupational setting, as low-level, long-term exposure are the most likely conditions (Shatkin and Kim Citation2015). Furthermore, the authors do not seem to have tested for impurities or interferences. Čolić et al. (Citation2015) also tested a high concentration (1000 μg/mL) that may have led to interferences with the assay system, however the authors did not observes any cytotoxic effects to L929 cells nor inflammatory response to human peripheral blood mononuclear cells (PBMNCs). Ilves et al. (Citation2018) found one of the non-modified CNF materials to reduce cell viability, while no cytotoxicity was observed from the three other materials tested on human monocytic cells (THP-1) at a maximum concentration of 100 µg/mL. The authors further looked at inflammation in vitro on this same cell line, and also found only this same non-modified material to induce an inflammatory response.
Most of the CNF materials that induced an inflammatory effect in vitro were in the lowest concentration range (): Also testing on human monocytic cells, Bhattacharya et al. (Citation2017) found that three of the four tested CNF materials at a maximum concentration of 25 µg/mL induced a pro-inflammatory response, but no significant cytotoxicity. The authors hypothesize that this may be due to the ‘deactivation of nuclear receptor pathways.’ Testing at a maximum of 30 µg/mL, Clift et al. (Citation2011) also observed a pro-inflammatory response in a 3D human lung cell coculture, although far less than the responses observed from multi-walled carbon nanotubes or crocidolite asbestos fibers. Unmodified CNF was also found to be the only one out of three tested materials to induce a significant inflammatory effect on human monocytic cell lines (Lopes et al. Citation2017). The tested range was the highest of the reviewed studies that showed an effect, being between 50 and 500 μg/mL.
Regarding studies looking at inflammation in vivo, shows the fold increase of neutrophils in BAL as a function of concentration. The three CNC materials experienced relatively low fold change, with the maximum being a 3.2 fold increase compared to the control at 200 µg/mouse (Yanamala et al. Citation2014). Neutrophils increased far more with CNF materials. The maximum fold increase can be seen in the linear dose-dependent increase resulting in the 75 fold increase at 200 µg/mouse observed by Catalán et al. (Citation2017), using a biocide control (Busan 1009 diluted in phosphate buffered saline). The other CNF materials testing less high concentrations all also induced a dose-dependent increases of neutrophils in BAL.
Figure 5. Fold increase of neutrophils in BAL as a function of tested concentrations of five in vivo studies looking at the inflammatory response of eight CNF materials (green) and three CNC (orange). Shapes of markers refer to specific materials.
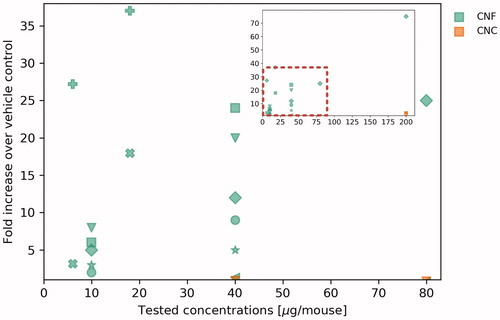
In , the various surface functionalizations used in the compiled studies looking at cytotoxicity and inflammation (in vitro and in vivo) are listed. Not many studies were identified, and the results from those that were highlight the need for further investigation into the effect of these surface coatings on toxicity and pro-inflammatory responses. In the investigation of the cytotoxicity of four CNF materials on human monocytic cells (THP-1), with concentrations ranging from 1 to 100 µg mL−1, only an unmodified material led to significant dose-dependent toxicity (Ilves et al. Citation2018), in contrast to the study on CNC discussed above, where only the modified CNC caused effects (Jimenez et al. Citation2017). Coating of CNC with sulfate groups and phenyl sulfonates was found to be a good way to control the antiviral activity of CNC (Zoppe et al. Citation2014). Also regarding the use of CNC for medical purposes, no toxicity to human brain microvascular endothelial cells (HBMECs) from aminated CNC has been found, making it a good candidate for the targeted delivery of therapeutics (Roman et al. Citation2009). Neither the aldehyde-modified CNC nor the Rhodamine B isothiocyanate-modified CNC showed significant cytotoxicity (Mahmoud et al. Citation2010, Yang et al. Citation2013).
Table 2. Types of Functionalization used in various studies looking at the cytotoxicity and inflammation of NC. The color and shade of the cells represent whether or not a significant effect was observed and the maximum tested concentration, respectively. For ease of comparison, studies reporting concentrations in µg/cm2 were not included.
Certain studies did however see a cytotoxic effect from functionalization: one study looked at three types of CNFs, namely without surface modification, carboxymethylated, and 2,3-epoxypropyltrimethylammonium chloride (EPTMAC) quaternized and found a pro-inflammatory effect on THP-1 cells only from the unmodified CNF (Lopes et al. Citation2017). Similarly, two unmodified CNFs led to greater pro-inflammatory response than the carboxymethylated and carboxylated CNFs, also tested on THP-1 cells (Ilves et al. Citation2018).
Regarding surface modification and inflammation in vivo, only the studies by Ilves et al. (Citation2018) and Hadrup et al. (Citation2019) investigated a same functionalization, namely carboxylation. Although Hadrup et al. (Citation2019) observed a significant pro-inflammatory response from this material, this response was lower than for the other investigated materials. Ilves et al. (Citation2018) found that the carboxylated material was the only one not to elevate the expression of cytokine IL-13. Overall, both studies found that carboxylation lowered toxicity. Looking at inflammation in vitro, Lopes et al. (Citation2017) found that carboxymethylation and EPTMAC quaternization lowered the inflammatory response compared to non-functionalized CNF.
Another factor playing a role in the observed effects of NC is the source of cellulose. Different sources will yield NC with various physicochemical properties, as well as impurities from previous processing (Roman Citation2015). The majority of studies used NC extracted from wood pulp, where pulping may result in certain traces of chemicals remaining. Cotton is also often used, and the same issue applies. The variety of cellulose sources and the observed effects regarding cytotoxicity and inflammation are presented in Table S3 in the Supplementary material.
The link between concentration and toxicity or inflammatory response is not straightforward. Since we summarized results from very different materials (in terms of NC source, physicochemical properties, etc.), assessed in different cell types/model systems and exposed for different time periods, such a ‘putative discrepancy’ can be explained. Furthermore, inflammation also largely depends on endotoxin (Dobrovolskaia, Shurin, and Shvedova Citation2016; Li, Fujita, and Boraschi Citation2017), and as shown in , only 18% of studies tested for contamination, making it tricky for conclusions to be made. Counterintuitively, some of the highest tested concentrations for cytotoxicity of CNC showed no significant toxicity, whereas a small number of materials showed toxicity in the lowest concentration range tested (). All of the studies falling in these categories had negative controls, although not all positive controls. No commonalities were identified between these materials to explain these results. Similarly, regarding in vitro inflammation, certain of the lowest tested concentrations induced an inflammatory response whereas the highest tested concentrations showed none (). The three studies responsible for these most extreme results all had both positive and negative controls, although did not always control for interferences (Clift et al. Citation2011; Čolić et al. Citation2015; Bhattacharya et al. Citation2017). These low concentrations having induced an effect highlight the need for long-term studies using realistic exposure concentrations to be used for risk assessments.
Sensitivity of cell lines
In addition to considering all different cell lines in in vitro tests without any distinction, we looked at toxicity relating to specific cell lines used in the context of cytotoxicity to identify whether any patterns emerged. Different cell lines used could explain differences in study results, as various cell lines are more or less sensitive. We identified 16 cell lines having been tested against CNC, and 13 for CNF (), from 22 studies. Data from 50 CNC and 45 CNF materials are shown. In the Supplementary material further explanation on the cell lines is given. To visualize the data, we employed a similar method as the probabilistic species sensitivity distribution (PSSD) used in ecotoxicology (Hauser, Li, and Nowack Citation2019; Wigger et al. Citation2020). PSSD curves represent the cumulative sensitivity of species to a substance within an environmental compartment. A Predicted No Effect Concentration (PNEC) is then derived from the HC5 (hazardous concentration for less than 5% of species) (European Chemicals Agency Citation2008). In our case, we replaced the species by cell lines (Bilal et al. Citation2019), to create a probabilistic cell sensitivity distribution (PCSD). Our goal was purely data visualization, and we did not extract a PNEC value from the resulting curve.
Figure 6. Sensitivity of various cell lines to CNC (top) and CNF (bottom). Multiple points for the same cell line (e.g. THP.1) represent different tested concentrations and NOAEL values.
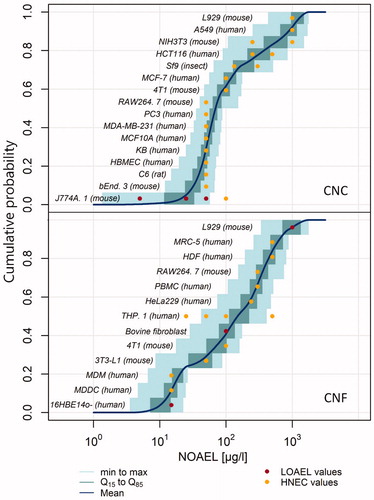
In order to explore whether any patterns exist regarding the cell lines and concentrations tested under the experimental conditions, we extracted endpoints from the compiled studies. These were either Lowest Observed Adverse Effect Level (LOAEL) values or, most frequently, Highest No-Effect Concentration (HNEC) values, when no significant toxicity was observed even for the highest tested concentration. We converted these LOAEL or HNEC values to No Observed Adverse Effect Level (NOAEL) values by using a dose-effect assessment factor (AFe) (European Chemicals Agency Citation2017). We applied an AFe of 2 for LOAEL values, and 1 for HNEC values. As no chronic studies were identified, we did not apply any acute-to chronic ratio. This PCSD allows for a visual representation of the sensitivity of the materials tested under their specific testing conditions. For example, regarding CNF, we see that considering the specific study conditions, 16HBE14o- cells are the most sensitive as their mean NOAEL value is the lowest, while the L929 cells are the least sensitive, having the highest NOAEL mean value. L929 cells are also the least sensitive for CNC under the respective study conditions, and J774A.1 cells the most. Although generalizations cannot be made from these results, gives an overview of what cell lines have been tested with regard to each material, and Table S4 in the Supplementary material gives more detail regarding each cell line. Most cell lines are from humans and mice. For both CNF and CNC, mouse fibroblasts L929 seem to be least affected compared to the other tested cell lines and within the tested conditions. For CNF, monocyte-derived macrophage (MDM) and monocyte-derived dendritic cell (MDDC), both immune cells, were among the most highly affected. Considering the sensitivity of these cell lines, it would be worthwhile to increase the pool of studies relating to the immunotoxicity of NC. Regarding CNF, the human bronchial epithelial cell (16HBE14o-) also appeared amongst the most sensitive cell line (same value as for MDDC and MDM). This is in line with the results found in vivo (), where studies looking at pulmonary exposure observed significant effects on various endpoints. 16HBE14o- should thus be considered and examined in more detail as a predictive in vitro model for pulmonary toxicity. There does not appear to be a pattern regarding tested species, with human and mouse cell lines being dispersed across both CNC and CNF PCSD curves. The results from should be interpreted with caution, as the testing conditions were different for all materials. Data quality is also an issue, and the resulting curve would likely be different if we had EC50 values rather than LOAEL and HNEC values. Interactions between the immune system and nanomaterials are highly relevant for toxicology studies (Jesus et al. Citation2019) and particle induced inflammation remains one of the most studied endpoints in nanotoxicology, as well as being very relevant for nanomedicine. Considering NC, relatively few studies on its pulmonary effects have been conducted, particularly regarding CNC (), and more studies would be needed to fill this gap.
Outlook and conclusion
Considering the interest that NC is receiving from research and industry, as well as the number of novel applications rapidly being developed, it is crucial that in-depth risk analyses are performed to ensure that all safety aspects have been considered before commercialization. In , we present some of the most relevant research needs based on the literature analyses conducted in this review. This list of five research needs is by no means exhaustive, but rather represents the areas where the largest knowledge gaps remain.
Table 3. Relevant identified research needs.
This review shows that up until now, few studies have been carried out regarding the hazards of NC to human health, and that there is room for improvement regarding the quality of those identified. Many study results are not comparable as different assays have been used and the physicochemical properties of the tested materials impact study outcomes, an issue affecting all ENMs. For certain endpoints study findings are ambiguous, for example in the case of in vitro studies looking at inflammation, cytotoxicity, oxidative stress, and genotoxicity. This can likely be attributed to a multitude of factors including variations in performed experiments and investigated materials, testing method, experimental model, dose/concentration range, cell type, exposure duration, and the physicochemical properties of the investigated NC (e.g. cellulose source, length, surface modification, crystallinity, etc.). The effect of various surface modifications also deserves further research. The relation between functionalization and toxicity is currently not straightforward, with studies having tested different types of surface modifications showed diverging results. Further investigations about the materials' surface properties would therefore be useful.
This review also highlights that inhalation of nanocellulose may cause adverse effects to human health. However, the scarcity of studies means that these effects are not yet fully understood or conclusive. Furthermore, little is known about the biopersistence of NC. More research needs to be done to ensure that NC is not affected by the fiber paradigm. Low dose, long-term studies looking at pulmonary exposure are still needed to fill this gap. Only one in vivo study performed a whole animal inhalation test, with all others having used installation or pharyngeal aspiration as exposure route. Occupational exposure to airborne NC during raw materials processing and manufacturing phase likely pose the greatest risk, and appropriate measures should be taken to ensure safe working conditions. Although few in numbers, none of the studies looking at the toxicity of NC via dermal contact have shown any cause for concern. The scenarios relating to dermal exposure pathways therefore probably do not pose a great risk within the occupational setting. The interest NC is generating for wound dressing may however call for more in-depth studies looking at the long-term impacts of NC on skin and especially on open wounds. Furthermore, the sensitivity of certain tested immune cells calls attention to the need for further studies looking at inflammation. Particularly when considering the fiber paradigm, as inflammation, as a mechanism of immune defense (Jesus et al. Citation2019) plays a role in the formation of mesothelioma, this endpoint remains key to ensuring the safe production and use of NC.
Our study aimed to establish patterns regarding these various factors and hazard to human health. The analyses performed regarding existing studies allowed for more detail regarding the current state of knowledge to be established, and thereby forms a clearer picture of future research needs. Not only more, but also better quality studies are still needed to fully understand the effects of NC in its various forms on human health. Considering the results from the quality assessment, using many of these study results for a risk assessment would likely be unsuitable. Checking for interferences and impurities was only done by a small share of the studies, and the lack of positive controls in many should also be considered. More rigorous physicochemical characterizations are needed, as well as more realistic exposure dose, chronic, repeated exposure studies, all with appropriate controls. At this point in time, more studies–and importantly of good quality–are required before being able to come to any firm conclusions regarding the safety of NC.
TNAN-2020-RA-0111-File002.docx
Download MS Word (423.3 KB)Acknowledgements
We would like to thank Edgar Hernandez for his help on an earlier version of this study.
Disclosure statement
No potential conflict of interest was reported by the author(s).
Additional information
Funding
References
- Bhattacharya, K., G. Kiliç, P. M. Costa, B. Fadeel, K. Bhattacharya, G. Kiliç, P. M. Costa, B. Fadeel, P. M. Costa, and B. Fadeel. 2017. “Cytotoxicity Screening and Cytokine Profiling of Nineteen Nanomaterials Enables Hazard Ranking and Grouping Based on Inflammogenic Potential.” Nanotoxicology 11 (6): 809–826. doi:10.1080/17435390.2017.1363309.
- Bilal, M., E. Oh, R. Liu, J. C. Breger, I. L. Medintz, and Y. Cohen. 2019. “Bayesian Network Resource for Meta-Analysis: Cellular Toxicity of Quantum Dots.” Small 15 (34): 1900510, 1–12. doi:10.1002/smll.201900510.
- Blanco, A., M. C. Monte, C. Campano, A. Balea, N. Merayo, and C. Negro. 2018. “Nanocellulose for Industrial Use: Cellulose Nanofibers (CNF), Cellulose Nanocrystals (CNC), and Bacterial Cellulose (BC).” Handbook of Nanomaterials for Industrial Applications. Newark, NJ: Elsevier Inc.
- Camarero-Espinosa, S., C. Endes, S. Mueller, A. Petri-Fink, B. Rothen-Rutishauser, C. Weder, M. Clift, and E. Foster. 2016. “Elucidating the Potential Biological Impact of Cellulose Nanocrystals.” Fibers 4 (4): 21. doi:10.3390/fib4030021.
- Card, J. W., and B. A. Magnuson. 2010. “A Method to Assess the Quality of Studies That Examine the Toxicity of Engineered Nanomaterials.” International Journal of Toxicology 29 (4): 402–410. doi:10.1177/1091581810370720.
- Catalán, J., M. Ilves, H. Järventaus, K.-S. Hannukainen, E. Kontturi, E. Vanhala, H. Alenius, K. M. Savolainen, and H. Norppa. 2015. “Genotoxic and Immunotoxic Effects of Cellulose Nanocrystals In Vitro.” Environmental and Molecular Mutagenesis 56 (2): 171–182. doi:10.1002/em.21913.
- Catalán, J., E. Rydman, K. Aimonen, K. S. Hannukainen, S. Suhonen, E. Vanhala, C. Moreno, et al. 2017. “Genotoxic and Inflammatory Effects of Nanofibrillated Cellulose in Murine Lungs.” Mutagenesis 32 (1): 23–31. doi:10.1093/mutage/gew035.
- Clift, M. J. D., E. J. Foster, D. Vanhecke, D. Studer, P. Wick, P. Gehr, B. Rothen-Rutishauser, and C. Weder. 2011. “Investigating the Interaction of Cellulose Nanofibers Derived from Cotton with a Sophisticated 3D Human Lung Cell Coculture.” Biomacromolecules 12 (10): 3666–3673. doi:10.1021/bm200865j.
- Čolić, M., D. Mihajlović, A. Mathew, N. Naseri, and V. Kokol. 2015. “Cytocompatibility and Immunomodulatory Properties of Wood Based Nanofibrillated Cellulose.” Cellulose 22 (1): 763–778. doi:10.1007/s10570-014-0524-8.
- Čolić, M., S. Tomić, and M. Bekić. 2020. “Immunological Aspects of Nanocellulose.” Immunology Letters 222 (February): 80–89. doi:10.1016/j.imlet.2020.04.004.
- DaNa 2016., Methodology of Selection, Aquisition and Evaluation of Toxicological Publications in the Project DaNa [online]. Accessed 5 March 2020. Available from: https://www.nanopartikel.info/files/methodik/DaNa_criteria_checklist_2016tox_en.pdf.
- de Lima, Renata, Leandro Oliveira Feitosa, Cintia Rodrigues Maruyama, Mariana Abreu Barga, Patrícia Cristina Yamawaki, Isolda Jesus Vieira, Eliangela M. Teixeira, Ana Carolina Corrêa, Luiz Henrique Caparelli Mattoso, and Leonardo Fernandes Fraceto. 2012. “Evaluation of the Genotoxicity of Cellulose Nanofibers.” International Journal of Nanomedicine 7: 3555–3565. doi:10.2147/IJN.S30596.
- Dobrovolskaia, M. A., M. Shurin, and A. A. Shvedova. 2016. “Current Understanding of Interactions between Nanoparticles and the Immune System.” Toxicology and Applied Pharmacology 299: 78–89. doi:10.1016/j.taap.2015.12.022.
- Donaldson, K., F. A. Murphy, R. Duffin, and C. A. Poland. 2010. “Asbestos, Carbon Nanotubes and the Pleural Mesothelium: A Review of the Hypothesis regarding the Role of Long Fibre Retention in the Parietal Pleura, Inflammation and Mesothelioma.” Particle and Fibre Toxicology 7 (1): 5. doi:10.1186/1743-8977-7-5.
- Dufresne, A. 2017. “Cellulose Nanomaterial Reinforced Polymer Nanocomposites.” Current Opinion in Colloid & Interface Science 29: 1–8. doi:10.1016/j.cocis.2017.01.004.
- Ede, J., K. Ong, M. Goergen, A. Rudie, C. Pomeroy-Carter, and J. Shatkin. 2019. “Risk Analysis of Cellulose Nanomaterials by Inhalation: Current State of Science.” Nanomaterials 9 (3): 337. doi:10.3390/nano9030337.
- Endes, C., S. Camarero-Espinosa, S. Mueller, E. J. Foster, A. Petri-Fink, B. Rothen-Rutishauser, C. Weder, and M. J. D. Clift. 2016. “A Critical Review of the Current Knowledge Regarding the Biological Impact of Nanocellulose.” Journal of Nanobiotechnology 14 (1): 1–14. doi:10.1186/s12951-016-0230-9.
- Endes, C., S. Mueller, C. Kinnear, D. Vanhecke, E. J. Foster, A. Petri-Fink, C. Weder, M. J. D. Clift, and B. Rothen-Rutishauser. 2015. “Fate of Cellulose Nanocrystal Aerosols Deposited on the Lung Cell Surface In Vitro.” Biomacromolecules 16 (4): 1267–1275. doi:10.1021/acs.biomac.5b00055.
- Endes, C., O. Schmid, C. Kinnear, S. Mueller, S. Camarero-Espinosa, D. Vanhecke, E. J. Foster, et al. 2014. “An In Vitro Testing Strategy towards Mimicking the Inhalation of High Aspect Ratio Nanoparticles.” Particle and Fibre Toxicology 11 (40). doi:10.1186/s12989-014-0040-x.
- European Chemicals Agency 2008., Guidance on Information Requirements and Chemical Safety Assessment Chapter R. 10 : Characterisation of Dose Concentration Response for Environment. Helsinki: Reproduction.
- European Chemicals Agency. 2017. Guidance on Biocidal Products Reguation: Volume III Human Health – Assessment & Evaluation (Parts B + C).
- Farcas, M. T., E. R. Kisin, A. L. Menas, D. W. Gutkin, A. Star, R. S. Reiner, N. Yanamala, K. Savolainen, and A. A. Shvedova. 2017. “Pulmonary Exposure to Cellulose Nanocrystals Caused Deleterious Effects to Reproductive System in Male Mice.” J Toxicol Environ Health A 79 (21): 39–46.
- Future Markets Inc. 2015. The Global Market for Nanocellulose. Edinburgh: Future Markets Inc.
- Gottschalk, F., and B. Nowack. 2011. “The Release of Engineered Nanomaterials to the Environment.” Journal of Environmental Monitoring 13 (5): 1145–1155. doi:10.1039/c0em00547a.
- Hadrup, N., K. Bram, T. Berthing, H. Wol, S. Bengtson, C. Kofoed, R. Espersen, et al. 2019. “Pulmonary Effects of Nano Fibrillated Celluloses in Mice Suggest That Carboxylation Lowers the Inflammatory and Acute Phase Responses.” Environmental Toxicology and Pharmacology 66: 116–125. doi:10.1016/j.etap.2019.01.003.
- Hanif, Z., F. R. Ahmed, S. W. Shin, Y. K. Kim, and S. H. Um. 2014. “Size- and Dose-Dependent Toxicity of Cellulose Nanocrystals (CNC) on Human Fibroblasts and Colon Adenocarcinoma.” Colloids and Surfaces. B, Biointerfaces 119: 162–165. doi:10.1016/j.colsurfb.2014.04.018.
- Hauser, M., G. Li, and B. Nowack. 2019. “Environmental Hazard Assessment for Polymeric and Inorganic Nanobiomaterials Used in Drug Delivery.” Journal of Nanobiotechnology 17 (1): 1–10. doi:10.1186/s12951-019-0489-8.
- Hoeng, F., A. Denneulin, and J. Bras. 2016. “Use of Nanocellulose in Printed Electronics: A Review.” Nanoscale 8 (27): 13131–13154. doi:10.1039/c6nr03054h.
- Ilves, M., S. Vilske, K. Aimonen, H. K. Lindberg, S. Pesonen, I. Wedin, M. Nuopponen, et al. 2018. “Nanofibrillated Cellulose Causes Acute Pulmonary Inflammation That Subsides within a Month.” Nanotoxicology 12 (7): 729–746. doi:10.1080/17435390.2018.1472312.
- Jesus, S., M. Schmutz, C. Som, G. Borchard, P. Wick, and O. Borges. 2019. Hazard Assessment of Polymeric Nanobiomaterials for Drug Delivery : What Can We Learn From Literature So Far, 7 (October).
- Jimenez, A. S., F. Jaramillo, U. D. Hemraz, Y. Boluk, K. Ckless, and R. Sunasee. 2017. “Effect of Surface Organic Coatings of Cellulose Nanocrystals on the Viability of Mammalian Cell Lines.” Nanotechnology, Science and Applications 10: 123–136. doi:10.2147/NSA.S145891.
- Jonoobi, M., R. Oladi, Y. Davoudpour, K. Oksman, A. Dufresne, Y. Hamzeh, and R. Davoodi. 2015. “Different Preparation Methods and Properties of Nanostructured Cellulose from Various Natural Resources and Residues: A Review.” Cellulose 22 (2): 935–969. doi:10.1007/s10570-015-0551-0.
- Krug, H. F., and P. Wick. 2011. “Nanotoxicology: An Interdisciplinary Challenge.” Angewandte Chemie 50 (6): 1260–1278. doi:10.1002/anie.201001037.
- Li, Y., M. Fujita, and D. Boraschi. 2017. “Endotoxin Contamination in Nanomaterials Leads to the Misinterpretation of Immunosafety Results.” Frontiers in Immunology 8 (MAY): 472–477. doi:10.3389/fimmu.2017.00472.
- Lie, E., E. Ålander, and T. Lindström. 2017. Possible Toxicological Effects of Nanocellulose – An Updated Literature Study, No 2. Stockholm: Innventia.
- Lopes, V. R., C. Sanchez-Martinez, M. Strømme, and N. Ferraz. 2017. “In Vitro Biological Responses to Nanofibrillated Cellulose by Human Dermal, Lung and Immune Cells: Surface Chemistry Aspect.” Particle and Fibre Toxicology 14 (1): 1–13. doi:10.1186/s12989-016-0182-0.
- Mahmoud, K. A., J. A. Mena, K. B. Male, S. Hrapovic, A. Kamen, and J. H. T. Luong. 2010. “Effect of Surface Charge on the Cellular Uptake and Cytotoxicity of Fluorescent Labeled Cellulose Nanocrystals.” ACS Applied Materials & Interfaces 2 (10): 2924–2932. doi:10.1021/am1006222.
- Menas, A. L., N. Yanamala, M. T. Farcas, M. Russo, S. Friend, P. M. Fournier, A. Star, et al. 2017. “Fibrillar vs Crystalline Nanocellulose Pulmonary Epithelial Cell Responses: Cytotoxicity or Inflammation?” Chemosphere 171: 671–680. doi:10.1016/j.chemosphere.2016.12.105.
- Nordli, H. R., G. Chinga-Carrasco, A. M. Rokstad, and B. Pukstad. 2016. “Producing Ultrapure Wood Cellulose Nanofibrils and Evaluating the Cytotoxicity Using Human Skin Cells.” Carbohydrate Polymers 150: 65–73. doi:10.1016/j.carbpol.2016.04.094.
- O’Connor, B., R. Berry, and R. Goguen. 2014. “Commercialization of Cellulose Nanocrystal (NCCTM) Production: A Business Case Focusing on the Importance of Proactive EHS Management.” Nanotechnology Environmental Health and Safety: Risks, Regulation, and Management (2nd ed.). Amsterdam: Elsevier Inc.
- Ong, K. J., J. A. Shatkin, K. Nelson, J. D. Ede, and T. Retsina. 2017. “Establishing the Safety of Novel Bio-Based Cellulose Nanomaterials for Commercialization.” NanoImpact 6: 19–29. doi:10.1016/j.impact.2017.03.002.
- Park, E. J., T. O. Khaliullin, M. R. Shurin, E. R. Kisin, N. Yanamala, B. Fadeel, J. Chang, and A. A. Shvedova. 2018. “Fibrous Nanocellulose, Crystalline Nanocellulose, Carbon Nanotubes, and Crocidolite Asbestos Elicit Disparate Immune Responses upon Pharyngeal Aspiration in Mice.” Journal of Immunotoxicology 15 (1): 12–23. doi:10.1080/1547691X.2017.1414339.
- Pereira, M. M., N. R. B. Raposo, R. Brayner, E. M. Teixeira, V. Oliveira, C. C. R. Quintão, L. S. A. Camargo, L. H. C. Mattoso, and H. M. Brandão. 2013. “Cytotoxicity and Expression of Genes Involved in the Cellular Stress Response and Apoptosis in Mammalian Fibroblast Exposed to Cotton Cellulose Nanofibers.” Nanotechnology 24 (7): 075103. doi:10.1088/0957-4484/24/7/075103.
- Piccinno, F., R. Hischier, A. Saba, D. Mitrano, S. Seeger, and C. Som. 2016. “Multi-Perspective Application Selection: A Method to Identify Sustainable Applications for New Materials Using the Example of Cellulose Nanofiber Reinforced Composites.” Journal of Cleaner Production 112: 1199–1210. doi:10.1016/j.jclepro.2015.06.105.
- Pitkänen, M., H. Kangas, O. Laitinen, A. Sneck, P. Lahtinen, M. S. Peresin, and J. Niinimäki. 2014. “Characteristics and Safety of Nano-Sized Cellulose Fibrils.” Cellulose 21 (6): 3871–3886. doi:10.1007/s10570-014-0397-x.
- Roman, M. 2015. “Toxicity of Cellulose Nanocrystals: A Review.” Industrial Biotechnology 11 (1): 25–33. doi:10.1089/ind.2014.0024.
- Roman, M., S. Dong, A. Hirani, and Y. W. Lee. 2009. “Cellulose Nanocrystals for Drug Delivery.” In: Polysaccharide Materials: Performance by Design, 4–81. Washington, DC: American Chemical Society.
- Schmutz, M., C. Som, H. F. Krug, and B. Nowack. 2017. “Digging below the Surface: The Hidden Quality of the OECD Nanosilver Dossier.” Environmental Science: Nano 4 (6): 1209–1215. doi:10.1039/C7EN00088J.
- Seabra, A. B., J. S. Bernardes, W. J. Fávaro, A. J. Paula, and N. Durán. 2018. “Cellulose Nanocrystals as Carriers in Medicine and Their Toxicities: A Review.” Carbohydrate Polymers 181: 514–527. (December 2017), doi:10.1016/j.carbpol.2017.12.014.
- Shatkin, J. A., and B. Kim. 2015. “Cellulose Nanomaterials: Life Cycle Risk Assessment, and Environmental Health and Safety Roadmap.” Environmental Science: Nano 2 (5): 477–499. doi:10.1039/C5EN00059A.
- Shvedova, A. A., E. R. Kisin, N. Yanamala, M. T. Farcas, A. L. Menas, A. Williams, P. M. Fournier, et al. 2015. “Gender Differences in Murine Pulmonary Responses Elicited by Cellulose Nanocrystals.” Particle and Fibre Toxicology 13 (1): 1–20. doi:10.1186/s12989-016-0140-x.
- Stockmann-Juvala, H., P. Taxell, and T. Santonen. 2014. Formulating Occupational Exposure Limits Values (OELs) (Inhalation & Dermal). Helsinki: Scaffold.
- Stoudmann, N., B. Nowack, and C. Som. 2019. “Prospective Environmental Risk Assessment of Nanocellulose for.” Environmental Science: Nano 6 (8): 2520–2531. doi:10.1039/C9EN00472F.
- Usov, I., G. Nyström, J. Adamcik, S. Handschin, C. Schütz, A. Fall, L. Bergström, and R. Mezzenga. 2015. “Understanding Nanocellulose Chirality and Structure-Properties Relationship at the Single Fibril Level.” Nature Communications 6 (1), 1–11. doi:10.1038/ncomms8564.
- Vartiainen, J., T. Pöhler, K. Sirola, L. Pylkkänen, H. Alenius, J. Hokkinen, U. Tapper, et al. 2011. “Health and Environmental Safety Aspects of Friction Grinding and Spray Drying of Microfibrillated Cellulose.” Cellulose 18 (3): 775–786. doi:10.1007/s10570-011-9501-7.
- Ventura, C., A. F. Lourenço, A. Sousa-Uva, P. J. T. Ferreira, and M. J. Silva. 2018. “Evaluating the Genotoxicity of Cellulose Nanofibrils in a co-Culture of Human Lung Epithelial Cells and Monocyte-Derived Macrophages.” Toxicology Letters 291: 173–183. doi:10.1016/j.toxlet.2018.04.013.
- Voisin, H., L. Bergström, P. Liu, and A. P. Mathew. 2017. “Nanocellulose-Based Materials for Water Purification.” Nanomaterials, 7 (3): 57. doi:10.3390/nano7030057.
- Wigger, H., D. Kawecki, B. Nowack, and V. Adam. 2020. “Systematic Consideration of Parameter Uncertainty and Variability in Probabilistic Species Sensitivity Distributions.” Integrated Environmental Assessment and Management 16 (2): 211–212. doi:10.1002/ieam.4214.
- Yanamala, N., M. T. Farcas, M. K. Hatfield, E. R. Kisin, V. E. Kagan, C. L. Geraci, and A. A. Shvedova. 2014. “In Vivo Evaluation of the Pulmonary Toxicity of Cellulose Nanocrystals: A Renewable and Sustainable Nanomaterial of the Future.” ACS Sustainable Chemistry & Engineering 2 (7): 1691–1698. doi:10.1021/sc500153k.
- Yang, X., E. Bakaic, T. Hoare, and E. D. Cranston. 2013. “Injectable Polysaccharide Hydrogels Reinforced with Cellulose Nanocrystals: Morphology, Rheology, Degradation, and Cytotoxicity.” Biomacromolecules 14 (12): 4447–4455. doi:10.1021/bm401364z.
- Zoppe, J. O., V. Ruottinen, J. Ruotsalainen, S. Rönkkö, L. S. Johansson, A. Hinkkanen, K. Järvinen, and J. Seppälä. 2014. “Synthesis of Cellulose Nanocrystals Carrying Tyrosine Sulfate Mimetic Ligands and Inhibition of Alphavirus Infection.” Biomacromolecules 15 (4): 1534–1542. doi:10.1021/bm500229d.