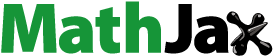
Abstract
Air pollution is an environmental factor associated with an increased risk of neurodegenerative diseases, such as Alzheimer’s and Parkinson’s, characterized by decreased cognitive abilities and memory. The limited models of sporadic Alzheimer’s disease fail to replicate all pathological hallmarks of the disease, making it challenging to uncover potential environmental causes. Environmentally driven models of Alzheimer’s disease are thus timely and necessary. We used live-cell confocal fluorescent imaging combined with high-resolution stimulated emission depletion (STED) microscopy to follow the response of retinoic acid-differentiated human neuroblastoma SH-SY5Y cells to nanomaterial exposure. Here, we report that exposure of the cells to some particulate matter constituents reproduces a neurodegenerative phenotype, including extracellular amyloid beta-containing plaques and decreased neurite length. Consistent with the existing in vivo research, we observed detrimental effects, specifically a substantial reduction in neurite length and formation of amyloid beta plaques, after exposure to iron oxide and diesel exhaust particles. Conversely, after exposure to engineered cerium oxide nanoparticles, the lengths of neurites were maintained, and almost no extracellular amyloid beta plaques were formed. Although the exact mechanism behind this effect remains to be explained, the retinoic acid differentiated SH-SY5Y cell in vitro model could serve as an alternative, environmentally driven model of neurodegenerative diseases, including Alzheimer’s disease.
1. Introduction
1.1. Particulate matter in polluted air is associated with cognitive decline and Alzheimer’s disease, yet causality is still not fully established
Strong correlations between particulate air pollution and dementia suggest airborne particulate matter as a possible environmental trigger of neurodegenerative diseases (Peeples Citation2020; Shi et al. Citation2020, Citation2021). However, whether the particulate matter in air pollution can cause dementia remains controversial (Underwood Citation2017). It is well-established that high levels of air pollution, particularly particulate matter smaller than 2.5 μm (PM2.5), are associated with cognitive decline and Alzheimer’s disease (AD) (Carey et al. Citation2018; Younan et al. Citation2020; Zhang, Chen, and Zhang Citation2018). However, it is important to note that the strength of this association does not necessarily imply causation. How air pollution particles enter the brain and possibly trigger neurodegeneration remains an open question (Underwood Citation2017). Nevertheless, a recent study has observed epigenetic gene regulation changes that are typically found in Alzheimer’s patients in both young adults and mice exposed to particulate air pollution (Calderón-Garcidueñas et al. Citation2020). In another study, the authors indicate that there may be no safe exposure limit to outdoor fine particulate air pollution (PM2.5) in relation to the risk of dementia (Weichenthal et al. Citation2022). Considering the emerging evidence, the Lancet Commission recognized air pollution as a risk factor for dementia, suggesting that reducing air pollution could potentially prevent or delay the onset of dementia, which is vital because pre-symptomatic diagnosis of disease is still lacking (Livingston et al. Citation2020).
AD, in particular, is a most prevalent form of dementia characterized by early memory loss and progressive damage to brain cells in the hippocampus, a region responsible for learning and memory (Drew Citation2018). Despite more than half a century of research, the exact causes of the disease remain uncertain, and no effective treatment yet exists (Drew Citation2018), despite recently FDA-approved therapies (Couzin-Frankel Citation2023; Piller Citation2023). Neurodegenerative diseases, such as AD, Parkinson’s, amyotrophic lateral sclerosis, and Huntington’s disease, are characterized by the misfolding and aggregation of specific proteins into large plaques (Eftekharzadeh, Hyman, and Wegmann Citation2016). Epidemiological data suggest that nanoparticles accumulated in the brain might trigger the formation of AD-like plaques. Furthermore, imaging of thin sections of amyloid cores from AD patients has corroborated the presence of ambient magnetite nanoparticles in the plaques (Plascencia-Villa et al. Citation2016). Although, most studies linking ambient air pollution with neurodegenerative disease have considered PM2.5 as an exposure metric, ultrafine particles (PM0.1) or nanoparticles (NP) are even more numerous, cause more inflammation, and can translocate to essentially all organs (Schraufnagel Citation2020). It has been suggested that combustion emissions such as diesel exhaust particles and engineered nanoparticles are of special importance in neurogenerative disease (Calderón-Garcidueñas and Ayala Citation2022). Existing research thus shows the need for new methods to confirm the causal connection between the components of particulate matter and neurodegeneration.
Based on a recent systematic review of literature focusing on organic environmental pollutants, Lopez-Suarez et al. proposed the SH-SY5Y cell line for neurotoxicity research. Namely, the effects on cultured SH-SY5Y cells include autophagy, several types of cell death, increased oxidative stress, mitochondrial dysfunction, neurotransmitter homeostasis disruption, and neuritic shortening (Lopez-Suarez et al. Citation2022), which are all subcellular/cellular hallmarks of neurodegenerative diseases (NDD) (Wilson et al. Citation2023). The SH-SY5Y cell line is a popular model for Parkinson’s disease (PD) as it possesses many characteristics of dopaminergic neurons (Xie, Hu, and Li Citation2010). The dopaminergic system is also involved in the progression (Martorana and Koch Citation2014), and especially in the early stages of AD (Sala et al., Citation2021). For example, degeneration of dopaminergic neurons in the ventral tegmental area at pre-plaque stages leads to memory dysfunction (Nobili et al. Citation2017). Although amyloid plaques, deposits of the β-amyloid (Aβ) peptide (Glenner and Wong Citation1984), are defining lesions in the Alzheimer’s disease (AD) brain.
Therefore we hypothesize that the retinoic acid-differentiated SH-SY5Y cells are potentially a relevant model for late-onset (sporadic) AD, which is more prevalent (>95%) than the early-onset (familial) variant (Masters et al. Citation2015). Whereas there are models for the familial type of AD, such as transgenic 5XFAD mice that rapidly accumulate massive cerebral Aβ 42 levels, resembling Alzheimer’s disease amyloid plaque formation (Oakley et al. Citation2006), the models for late-onset AD that wouldn’t form amyloid beta plaques spontaneously are still sparse or incomplete. Here we show that retinoic acid-differentiated SH-SY5Y cells do not spontaneously form amyloid beta plaques (Riegerová et al. Citation2021). Therefore, the differentiation process is insufficient to cause the formation of amyloid beta plaques. Instead, other studies often introduce amyloid-beta peptides externally in order to induce plaque formation in a non-genetically modified model that doesn’t produce them spontaneously (He et al. Citation2018).
In this paper, we investigated whether exposure of neuron-like cells to particulate matter constituents could replicate neurodegenerative phenotypes in vitro. These include the formation of extracellular amyloid-β-containing plaques and reductions in both neurite density and length. Employing a high dose in vitro exposure of neurons allowed us to emulate a dose accumulated over a lifetime, delivering it within approximately a day, depending on the sedimentation rate of the nanomaterial. Previously, we employed a similar high-dose in vitro exposure technique in a coculture of lung epithelial cells and alveolar macrophages, successfully replicating phenotypic characteristics observed in long-term lung inflammation occurring 90 days after exposure in mice (Danielsen et al. Citation2020; Kokot et al. Citation2020).
We tested four types of nanomaterials: two engineered nanomaterials and two nanomaterials commonly found in polluted air:
anatase TiO2 nanotubes are an engineered nanomaterial relevant predominantly to occupational risk. In general, TiO2, regardless of its form and crystalline structure, also known as E171, is widely used as a white colorant in food, paints, coatings, pharmaceuticals, cosmetics, and even toothpaste. E171 contains a fraction of nanosized primary particles (<100 nm) (Peters et al. Citation2014, Citation2020). Several in vivo studies reviewed by Song et al. (Song et al. Citation2015) have demonstrated that the TiO2 nanoparticles can be transported and accumulated in the brain, but mostly in intranasal instillation or inhalation studies, via a nose-to-brain path, eventually leading to central nervous system dysfunctions. Another group showed that titanium content in mouse hippocampus after 90 days of continuous intranasal exposure to TiO2 nanoparticles was 0.6 mg/g tissue at the highest administered dose of 10 mg/kg body weight (Ze et al. Citation2014), much higher than the levels observed in humans. In the case of engineered nanoparticles, functionalization with KD8 peptide has been used to enhance their ability to cross the blood-brain barrier and accumulate at the site of Aβ deposits in the brain. Thus, for such purposefully engineered nanoparticles, ingestion or intra-venous injection with subsequent delivery across the blood-brain barrier might also be important (Haochen Zhang et al., Citation2022). Titanium can be found in human tissue at lower average concentrations ranging from 1 μg/g (Peters et al. Citation2020) up to 80 μg/g (Baj et al. Citation2023) in the human brain. Thus, neuroinflammation and spatial memory impairment observed in mice occur at more than ten times higher brain content than in humans. The effect of TiO2 nanoparticles on the brain is thus still controversial since detrimental effects are observed only at very high doses. Here, we also use a very high concentration of 3 mg/g (mass of a nanomaterial (mg) versus mass of cells (g), supplement page 40), akin to in vivo dosages, to show that inflammatory, engineered TiO2 nanotubes induce the formation of extracellular amyloid β-containing plaques and cause shortening of neurites. Yet, they do not cause such extensive neurite shortening as iron oxide and diesel exhaust particles.
Iron oxide (γ-Fe2O3) nanoparticles are commonly found in road traffic emissions from brake wear (Maher Citation2019). Metal ions such as copper, iron, and zinc have been found in Alzheimer’s disease senile plaques. Copper, in particular, binds to beta-amyloid peptides with high affinity, promotes Aβ aggregation, and contributes to neurotoxic reactive oxygen species formation (Du et al. Citation2021). Postmortem analyses of amyloid plaques revealed copper, iron, and zinc accumulation by 5.7, 2.8, and 3.1 times the levels observed in normal brains, respectively (Liu et al. Citation2019). However, the origin of metal ions in Alzheimer’s disease plaques is not understood. Our extensive review of the current literature yielded no references regarding the possible environmental origin of metal oxides. On the other hand, there is plenty of evidence that polluted air from coal combustion contains several metals, including iron (Suarez and Ondov Citation2002). The PM2.5 concentrations of iron oxide particles is high in underground railway stations. Sheikh et al. (Sheikh et al. Citation2022) showed that the majority of iron oxide particulate matter exposure in the London Underground was maghemite, the material used in our study, as confirmed by Mössbauer analyses (Tadic et al. Citation2014). Occupational exposure to welding fume particles (iron-rich particles and magnetite) is associated with an increased risk of developing AD. Finnegan et al. showed that non-chemically preserved AD patients’ postmortem tissue samples contained the average concentration of iron peaking at 400 mg/g (Finnegan et al. Citation2019). Furthermore, airborne iron oxide (magnetite) particles can be concentrated in smaller regions of the human brain (Maher et al. Citation2016), thus reaching even higher local concentrations. Such magnetite nanospheres are ubiquitous and abundant in airborne particulate matter pollution. They are smaller than 200 nm in diameter and can enter the brain directly via the olfactory bulb (Maher et al. Citation2016). Examination of the human frontal cortex brain samples obtained from subjects who lived in Mexico City revealed that magnetite nanoparticles have an external, rather than an endogenous source (Maher et al. Citation2016). Although the highest brain magnetite concentration was 10 µg/g of dry tissue, the transmission electron micrographs of brain thin sections showed that the nanoparticles are in about 1% of the total area; thus in vitro concentrations of 1 mg/g might be more relevant to human exposure to polluted air.
Since iron is present in most diesel exhaust samples, representing on average about 20% w/w (Viskup, Wolf, and Baumgartner Citation2020), we estimate that the brain burden of diesel exhaust particles might reach up to 2 mg/g tissue weight, coinciding with the dosage used in this manuscript. Although iron might not be a good exposure metric for diesel exhaust, we also considered an exposure concentration of 100 μg/m3 as elemental carbon (Debia et al. Citation2017), to estimate the exposure over an entire working life (Supplement Chapter 7 Dose relevance). The specific surface area of diesel exhaust particles is similar to particles generated by welding, with particle sizes mostly below 200 nm, so the concentration of insoluble surface area that might accumulate in the brain may be in the same range as occupational exposure of welding workers, given that the transport mechanisms across barriers are similar for the two types of aerosols.
As an example of reportedly nontoxic particulate matter, we tested CeO2 nanoparticles, which are used as fuel additive in motor vehicles to reduce carbon monoxide, nitrogen oxides, and hydrocarbons in exhaust gases. Thus, the particles are released into the air. The effects of CeO2 nanoparticles reported in the literature are contradictory, demonstrating all kinds of effects, from protective to toxic (Gagnon and Fromm Citation2015). For example, CeO2 nanospheres and nanorods improved cognitive impairment following mild traumatic brain injury in VC57BL/6J male mice at the dose of 0.02 mg/g body weight. However, the concentration in the brain was not reported (Fiorani et al. Citation2015; Gagnon and Fromm Citation2015).
1.2. The challenge of reproducing all hallmarks of Alzheimer’s disease (AD) in animal models: the limitations of genetically modified models and the need for an environmentally triggered model
AD is defined by a triad of pathological hallmarks (1) amyloid β peptide plaques, (2) aggregation of Tau protein, and (3) neuronal degeneration. Accumulation of amyloid β plaques precedes cognitive impairment by at least 20 years (Scheltens et al. Citation2016). Extracellular deposits of amyloid β have long been considered the initial event of the disease process (Leng and Edison Citation2021), at least in less prevalent familial cases (Karran, Mercken, and Strooper Citation2011). The accumulation of Tau into large aggregates, initially appearing in dystrophic neurites surrounding Aβ plaques, is usually referred to as neuritic plaque tau (NP tau, followed by the formation and spread of neurofibrillary tangles (NFT) and neuropil threads to other neurons. Recently He et al. presented a new mouse model, which offered a new way to explain how the amyloid β plaque environment accelerates the spread of Tau pathology in AD patients’ brains, consistent with imaging studies and investigations of postmortem AD brains (He et al. Citation2018). Axonal and dendritic degeneration and neuronal death, reflected in reduced neuronal arborization and decreased neuron density, is the final pathological hallmark that coincides with the appearance of intracellular Tau deposits and symptoms of advanced cognitive decline (Falke et al. Citation2003).
Here, we show that high-dose in vitro exposure of neurons to particulate matter constituents, such as diesel exhaust and iron oxide nanoparticles, replicates hallmarks of AD pathology by triggering amyloid β plaque formation and reducing the density and length of neurites. Since less than 0.1% (Blennow, de Leon, and Zetterberg Citation2006) of AD is caused by a single genetic mutation, the model system consisting of neurons without familial mutations might thus be regarded as an alternative, perhaps more relevant, environmentally driven model of neurodegenerative diseases, including Alzheimer’s disease.
2. Materials and methods
2.1. Cell line
The cells we refer to as neurons throughout the manuscript are retinoic acid (RA) differentiated human neuroblastoma SH-SY5Y cell line. The undifferentiated SH-SY5Y human neuroblastoma cell line is commercially available from the American Type Culture Collection (ATCC, www.atcc.org or www.lgcstandardsatcc.org, catalog number CRL-2266™). The SH-SY5Y is a comparatively homogeneous human neuroblastoma cell line (ATCC® CRL-2266™) is one of the neurosciences’ most used cell lines, either undifferentiated or differentiated into neuron-like cells. The SH-SY5Y cell line exhibits several neuronal markers when differentiated with retinoic acid (RA) to a more mature neuron-like phenotype (Kovalevich and Langford Citation2013). Differentiation with RA results in the cells becoming more polarized, extending long neurites, and forming functional synapses (Martin, Gandawijaya, and Oguro-Ando Citation2022). Besides, as they are human-derived, SH-SY5Y neuroblastoma cells express several human-specific proteins that are missing in rodent primary cultures or animal models. The cells grew as a mixture of floating and adherent cells in DMEM/F-12 (Gibco) medium supplemented with 1% penicillin/streptomycin (Sigma-Aldrich), 2 mM GlutaMax (Gibco), and 10% FBS (BioChrom AG). For SH-SY5Y differentiation, we seeded 1 × 106 cells cm−2 on the collagen-coated, microscopy-adapted plates (8-wells, Ibidi). After 24 hours we added the differentiation medium containing Dulbecco’s modified eagle medium DMEM/F12 without phenol red (#21041025, Thermo Fisher), supplemented with 1% penicillin/streptomycin (Sigma-Aldrich), 2 mM GlutaMax (Gibco), 0,5% FBS (BioChrom AG) and 10 uM retinoic acid (fisher scientific, #AC207341000). Old media was replaced with 100 µL fresh differentiation media every other day, until 7th day when we terminated the differentiation.
2.2. Nanomaterial preparation
We employed a dispersion technique using cup horn sonication to disperse materials, namely anatase TiO2, maghemite γ-Fe2O3, CeO2, and diesel exhaust particles, in a buffer. The buffer solution utilized in this study was 1 mM bicarbonate buffer with a pH of 10, which was specifically chosen due to its low osmolarity and high pH properties that minimize the charge screening of the active surface of the materials.
To ensure uniform dispersion of the materials in the bicarbonate buffer, the materials were resuspended so that each 3 µL of the suspension contained 3 cm2 of the material surface. The weighted mass of each material and the volumes of bicarbonate buffer utilized to prepare the solutions are presented in the .
Table 1. Preparation of nanomaterial dispersions used in this study.
The synthesis of TiO2 nanotubes followed a rigorous procedure and has been extensively documented (Umek et al. Citation2007). In brief, the process began with the controlled hydrothermal synthesis of sodium titanate nanotubes. These sodium titanate nanotubes were subsequently subjected to ion exchange to transform them into hydrogen titanate nanotubes. Finally, through a carefully conducted thermal treatment, the hydrogen titanate nanotubes were successfully converted into the desired TiO2 nanotubes. The detailed methodology and experimental conditions can be found in the comprehensive study by Umek et al. (Citation2007).
The iron oxide nanoparticles in the form of maghemite (γ-Fe2O3) were synthesized by co-precipitation from aqueous solutions of Fe2+ and Fe3+ salts. The synthesis methodology is described in detail in our previous publications (Kralj et al. Citation2012; Kralj and Makovec Citation2014; Nemec et al. Citation2020). The saturation magnetization of as-synthesized nanoparticles is ∼64 emu g−1. The size of nanoparticles was 11.6 ± 2.1 nm which was estimated by analyzing 100 transmission electron microscopy images using ImageJ software. For the preparation of stable colloidal suspension of superparamagnetic nanoparticles its surface was electrostatically stabilized using citric acid as described elsewhere (Nemec and Kralj Citation2021). The final concentration of citric-acid stabilized iron oxide maghemite nanoparticles in aqueous suspension was 1.8 mg ml−1.
DEP9.7 diesel exhaust particles were collected after treatment from an experimental modern heavy-duty diesel engine under highly controlled combustion conditions in a laboratory environment. The particle production and collection have been described in detail previously (Gren et al. Citation2020). The engine was operated to control the physical and chemical properties of the diesel exhaust particles (DEP9.7). To produce the DEP9.7 particles, the engine was run at an engine intake O2 concentration of 9.7% using petroleum-based ultralow-sulfur diesel fuel of Swedish MK1 standard. The diesel engine combustion was modified to this low-temperature combustion condition using a high amount of exhaust gas recirculation. The exhaust gas recirculation lowers flame temperatures and reduces both soot formation and soot oxidation processes. Using the high control of the engine, the DEP9.7 particles were intentionally designed to have a high content of polycyclic aromatic hydrocarbons (PAH) and refractory organic carbon relative to the content of elemental carbon. DEP9.7 has been characterized extensively (Bendtsen et al. Citation2020, p. 6; Gren et al. Citation2020). DEP were collected on a Teflon filter and extracted using methanol with >80% recovery. The DEP9.7 had a primary particle size of 22 nm and an estimated specific surface area of 152 m2g−1. The carbon content in DEP9.7 is 33% elemental carbon and 67% organic carbon of which 33% is refractory organic carbon. DEP9.7 contains a number of metals (Bendtsen et al. Citation2020, p. 6) predominately Cu (2349 µg g−1), Fe (220 µg g−1), Sr (99 µg g−1) and Mn (92 µg g−1). DEP9.7 has a high content of PAH (26.8 mg g−1) and a list of specific PAH can be found in (Bendtsen et al. Citation2020, p. 6). In comparison to four other sample types from the same engine, DEP9.7 had the lowest formation of Reactive Oxygen Species in the cell free DCFH assay on a mass basis. Extractable organic matter from DEP9.7 had the highest genotoxicity potential in lung epithelial (A549) cells of the five samples (Rothmann et al. Citation2023).
CeO2 NPs ‘50nm agglomerates’ composed of 5 nm CeO2 nanoparticles as determined from TEM images (batch AN-123) were produced and characterized by Applied NanoParticles - A Nanotech Engineering Company (Spain) (https://www.appliednanoparticles.eu/). CeO2 NPs have a mean diameter of 6.2 ± 1.3 nm as measured by TEM, the agglomerates have a mean diameter of 200 ± 6 nm as measured by DLS and a zeta potential −32.5 ± 0.07 mV measured in miliQ water at pH 9 at 0.5 mS cm−1.
The resulting suspensions of the nanomaterials were then subjected to cup horn sonication in an ice bath for 15 min, utilizing 5 seconds on − 5 seconds off regime for a total duration of 30 min, at a power setting of 20–30 W (amplitude 70) to guarantee optimal dispersion.
The volume of material dispersion containing a 10 times larger surface than that of neurons was added in a dropwise manner, prior to microscopy, to cover the surface of the in vitro model. The volume applied to cells never exceeded 10% of the media volume.
2.3. Fluorescent labeling
Different fluorescent labels used in this study are given in , below.
Table 2. List of fluorescent labels used in this study.
In our experimental procedures, a specific set of labels was utilized for each experiment, which will be detailed in the Materials and Methods section and the corresponding figure legends. In general, live neurons were differentiated and incubated with nanomaterial, followed by staining with fluorescent labels according to the manufacturers’ guidelines.
It is noteworthy that CTG was the only label added one day prior to microscopy, while other labels were added immediately before imaging. Additionally, only CTG was washed with PBS, whereas other labels were not washed. Antibodies used in this study will be described later in this section. Briefly, they were conjugated with fluorescent dyes and were added in specified concentrations just prior to microscopy.
Anti- Aβ antibodies are raised against amino acids 1–40 of β-Amyloid of human origin (https://www.scbt.com/p/beta-amyloid-antibody-20-1). The β-Amyloid Antibody (20.1) is a mouse monoclonal antibody of the IgG2b κ isotype specifically designed to target β-amyloid and amyloid precursor protein (APP) fragment from human sources.
The extracellular tau deposits were labeled with Tau (Tau-5) Mouse Monoclonal Antibody (Product # AHB0042) of IgG1 kappa κ isotype. It recognizes both phosphorylated and non- phosphorylated human tau proteins.
Both antibodies were added at 2 μg mL−1 concentration to 7-day RA differentiated neurons in 1% BSA at 37 °C. Imaging started immediately after the addition of antibodies.
2.4. Microscope setup
Following incubation with nanomaterial, the living samples were labeled and imaged using a state-of-the-art imaging system consisting of an Olympus (Olympus IX83) inverted microscope body equipped with a laser-scanning confocal and STED unit (Stedycon, Abberior). Stage top incubator (Okolab H301-MIN) maintains atmosphere with 37 °C, 5% CO2, and >95% humidity to enable long term imaging of living cells.
The images were captured using a confocal microscope equipped with either a 20x magnification objective and 0.8 numerical aperture (NA) lens or a 60× magnification objective with a 1.2 NA lens. The microscope system incorporates four pulsed laser sources (Abberior) with a pulse duration of 120 ps and a maximum power of 50 µW at the sample plane. Additionally, four avalanche photodiode (APD) detectors are utilized for signal detection. The pulse repetition frequency was 80 MHz. The STED depletion laser, operating at a wavelength of 775 nm, had the same repetition frequency as the excitation lasers, a pulse duration of 1.2 ns, and a maximum power of 170 mW at the sample plane.
We detected nanoparticles in the label-free, backscatter detection mode, utilizing the 488/488 ± 5 nm excitation/detection. Due to the large coherence of the laser, the backscattered light exhibited a strong speckle pattern, which was removed by Fourier transform bandpass filter (1–100 pixels) on scattering images. These images were subsequently binarized to obtain nanomaterial masks.
Please see the supplement for information regarding nanomaterial aggregation in cell medium, and automated-multi region of interest (ROI) data analysis and quantification of extracellular amyloid β containing plaques and neurite lengths.
3. Results and discussion
3.1. TiO2 nanotubes trigger the formation of extracellular amyloid β, Tau, and neuronal cytosolic components containing plaques
Since anatase TiO2 nanotubes induce retention of organic debris in alveolae with nanoparticles at the core of these deposits (Danielsen et al. Citation2020; Kokot et al. Citation2020), we tested whether the formation of similar deposits of organic molecules might occur inside the brain. We employed a human neuroblastoma cell line called SH-SY5Y, which is extensively utilized in studies due to their ability to differentiate into neuron-like cells. When differentiated, these cells exhibit characteristics resembling dopaminergic neurons found in the ventral hippocampus, which are involved in cognitive functions (Titulaer et al. Citation2021).
After the differentiation of SH-SY5Y, resulting in neuron-like cells with extensively branched neurites (, green) (Agholme et al. Citation2010; Kovalevich, Santerre, and Langford Citation2021; Kovalevich and Langford Citation2013), we exposed them to TiO2 nanotubes (, red). Most of the added TiO2 nanoparticles aggregate (Supplement Figure S1–S18) and settle to the glass surface never interacting with the cells (purely red signal in ), whereas some portion of added nanoparticles, mostly single nanotubes (Urbančič et al. Citation2018), translocate into the cells and gets excreted after a day (Kokot et al. Citation2020). We identified the nanoparticles that interact with cells by their co-localisation with the cytosolic label CellTracker™ Green CMFDA (CTG) (green, ), with which we labeled the neurons prior to exposure. This label, once internalized by the cells, is acted upon by cellular esterases rendering it fluorescent throughout the cytoplasm. Without this interaction, it remains non fluorescent. In addition, thiol reactivity increases its intracellular retention through several generations (Beem and Segal Citation2013), thus precluding it from freely exiting the cells to adhere to the surface of TiO2 nanotubes that had not interacted with the cells’ interior.
Figure 1. SH-SY5Y-derived neurons exposed to anatase TiO2 nanotubes form amyloid-β and Tau protein-containing extracellular plaques. (A) Representative image of differentiated SH-SY5Y cells in culture; for differentiation, cells were grown for seven days in DMEM/F12, 10% fetal bovine serum, and 10 µM retinoic acid, followed by 7 d in serum-free DMEM/F12 and 10 ng/mL brain-derived neurotrophic factor. Note the altered morphology and pronounced neurite outgrowth upon differentiation. Live cell culture was stained with cytosolic fluorophore CellTracker™ green (green), which is retained and fluoresces only within a cell, a mouse monoclonal antibody raised against amino acids 1–40 of Aβ of human origin, for detection of amyloid precursor protein and Aβ (blue), and Tau-5 mouse monoclonal antibody against total Tau (white). Within the large field of view, the rectangles denote regions enlarged in the panels (B–D) showing examples of heterogeneous extracellular plaques at higher magnification: (B,C) large plaques with low Aβ surface density (fluorescence intensity signal divided by the area of the signal) and high tau surface density; (D) small plaques with high Aβ surface density and low tau surface density; (E) Properties of all the Aβ plaques from 15 regions of interest, each covering 120 × 120 μm2 in terms of surface densities with x-axis: tau antibody, y-axis: Aβ antibody, z-axis: neuronal cytosolic label, size of a sphere: plaque radius, and color: density of nanoparticles in a plaque, each sphere representing one plaque. Most of the nanomaterial is deposited on the glass surface without interacting with neurons (red), whereas the nanomaterial interacting with the neurons contains Aβ (blue), nanomaterial (red), and neuronal cytoplasmic components (green), while Tau (white) can be missing; the grey and blue ellipses denote plaques with high and low density of Tau, such as those in panels B/C and D, respectively. (F) The size distribution of the plaques, showing exponential decay of the number of plaques versus plaque size.
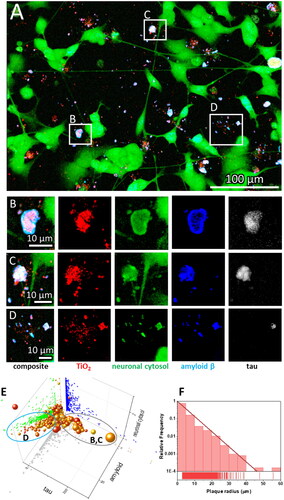
Moreover, we observed that many of the above-mentioned aggregates also contained extracellular deposits of amyloid β (Aβ) peptide, as indicated by immunostaining with a mouse monoclonal antibody raised against amino acids 1–40 of Aβ of human origin, for detection of amyloid precursor protein and Aβ (, blue). Namely, aggregation of Aβ is a proteolytic product of amyloid precursor protein (AβPP) by β- and γ-secretases, resulting in two major Aβ isoforms: Aβ42 (42 residues long) and Aβ40 (40 residues long). Although the concentration of Aβ40 in cerebral spinal fluid is several-fold higher than that of Aβ42, the Aβ42 is the predominant constituent of amyloid β plaques in AD brains (Iwatsubo et al. Citation1994, Citation1995), while Aβ40 is present in only some of the plaques (Gravina et al. Citation1995; Mak et al. Citation1994), suggesting that initial plaque formation involves only Aβ42 (Gu and Guo Citation2013). Therefore, we are aware of the limitation that quantifying only Aβ40 might not be suitable for the detection of early Aβ containing plaques in vitro.
Phosphorylated Tau (p-tau) is also one of the biomarkers for the early stages of AD. For example, Tau phosphorylated at threonine-231 (T231) increase early in the preclinical stage of AD, probably in response to Aβ pathology (Suárez-Calvet et al. Citation2020). Therefore, we labeled the extracellular tau deposits with tau (Tau-5) mouse monoclonal antibody that should recognize both phosphorylated and non-phosphorylated human tau proteins (, white). Since cells can release Tau even if not exposed to a toxicant (Karch, Jeng, and Goate Citation2012), we cannot deduce whether nanoparticles bind Tau within a cell and transport it out or the cells release more Tau, which binds to nanoparticles outside of the cells. Tau is a neuronal microtubule-associated protein found predominantly in axons. The C-terminus binds axonal microtubules while the N- terminus binds neural plasma membrane components (Brandt, Léger, and Lee Citation1995; Padmanabhan et al. Citation2022). Many other roles for Tau were speculated (Sotiropoulos et al. Citation2017), including its role in insulin signaling (Marciniak et al. Citation2017) and being released extracellularly as a free protein (De La-Rocque et al. Citation2021), which can bind to receptors on living cells (Mudher et al. Citation2017), including amyloid precursor protein (Takahashi et al. Citation2015). Yet the precise mechanisms of tau pathology remain unanswered (Huiqin Zhang et al., Citation2021).
We observed the formation of extracellular deposits of Aβ and Tau ( composite, white deposits near green neurons), which agrees with the current knowledge of extracellular plaque composition found in AD patients. There, two types of brain lesions are commonly observed: Aβ plaques and tau tangles, arising from the aggregation of misfolded Aβ and hyperphosphorylated tau proteins, respectively (Walker Citation2020). Aβ plaques have long been considered the initial event of the disease process (Leng and Edison Citation2021). However, recent studies have shown that Aβ plaques can act as seeds for the initial Tau accumulation into large aggregates containing both proteins. This initial accumulation occurs in dystrophic neurites surrounding Aβ plaques, referred to as neuritic plaque tau (NP Tau), consistent with imaging studies and investigations of postmortem AD brains (He et al. Citation2018). Our data () corroborates this notion, showing protein deposits containing both Aβ and tau protein, resembling structures observed in mice and patients by He et al. (Citation2018).
To examine the heterogeneity of extracellular Aβ plaques, we analyzed 15 regions of interest (ROI), each covering 120 × 120 μm2. An example of the algorithm we used for calculating densities of plaque components is shown in the supplement (Figure S21). We identified more than six thousand plaques, the properties of which are plotted in a 5-dimensional graph in . As the x coordinate, we assigned the Aβ signal density (fluorescence signal intensity divided by the surface area of an extracellular deposit = plaque), as y coordinate, we assigned the Tau signal density, and as z coordinate, we assigned the cytoplasmic signal density. In this way one can see that some of the points lie in the xz plane (y = 0, no tau signal), yet there are no points in the xy (z = 0) and yz (x = 0) plane indicating that all extracellular deposits contain Aβ (blue) and cytoplasm of neurons (green). Each sphere in the graph represents one plaque, with the size of the sphere proportional to the plaque radius and the color representing the density of the nanomaterial according to the color scale. Interestingly, some plaques lack Tau (x axis), whereas others contain lots of it. This observation aligns with the heterogeneous nature of plaques found in AD patients, where there is high diversity in all plaque components except for Aβ, which is universally present in all plaques (Walker Citation2020).
Notably, the amount of Aβ in our plaques is proportional to the amount of cytosolic label observed, indicating that the neurons generate Aβ because fluorescing cell tracker label must have originated from neuronal cytoplasm ( distribution of green dots in an amyloid-neuronal cytosol plane). This is in accordance with the finding that plaque formation initiates within the neuron, and subsequential accumulation of fibrillar Aβ occurs intraluminally (Le Bras Citation2022; Lee et al. Citation2022; Pensalfini et al. Citation2014). Furthermore, many other cell proteins have been identified within Aβ plaques. For example, a comparison of biomolecules in Aβ plaques (amyloidome) of human Aβ plaque regions versus non-plaque regions of AD and healthy controls revealed significantly higher levels of neuronal proteins (Bai et al. Citation2021; Drummond et al. Citation2017; Xiong, Ge, and Ma Citation2019), further strengthening the idea that the plaque initially forms within the neuron.
The number of the plaques decreases exponentially with the radius of a plaque (), with the characteristic length of 3.3 μm and most plaques having a radius below 40 μm, corresponding to a cross-section area of 5000 μm2. Such a size distribution, including exponential behavior and maximum size, is similar to the distribution of Aβ oligomeric structures observed in vitro on lipid membranes by Tahirbegi et al. (Tahirbegi et al. Citation2020). However, the plaque sizes we observed are larger than suggested by Serrano-Pozo et al., who showed that in AD, patients maximum plaque sizes reach up to 500 μm2 (Serrano-Pozo et al. Citation2012). We speculate this difference might be due to the absence of microglia in our cell culture model, as the microglia are known to influence plaque formation (Bai et al. Citation2021; Leng and Edison Citation2021). For example, Shabestari et al. showed that re-engraftment the population of microglia in AD mice brains resulted in the formation of more compact, smaller plaques (Kiani Shabestari et al. Citation2022). Nevertheless, the size of plaques seems to be loosely governed by the density of Tau and Aβ proteins within them. Larger plaques tend to have a higher density of Tau, and smaller ones have higher Aβ density ().
3.2. Amyloid β (Aβ) plaques form at the sites of damaged neurites
Above we have shown that exposure to nanoparticles can trigger the formation of Aβ plaques, possibly due to the direct damage to neurons, as evidenced by the presence of Tau and neuronal cytosolic components within the plaques. This is not that surprising considering our previous studies where we showed that these nanotubes can disturb plasma membrane (Urbančič et al. Citation2018) and cytosolic organelles (Kokot et al. Citation2020), including microtubule structure, within minutes after exposure. We next conducted high temporal and spatial resolution imaging of neurite morphology at different confocal planes () to investigate whether nanotubes can directly damage the neurites they contact. Next to an exemplary Aβ plaque that spans a height of 3 μm, we observed an axon extending from the neuron body at the lowest z plane (, z = 0 μm) that stopped at the AB plaque. Interestingly, another axon above the first remained intact and reached over the plaque three μm above the lowest point (, z = 3 μm).
Figure 2. Neurite dystrophy and early amyloid β plaque formation at the sites of retracted neurites caused by anatase TiO2 nanotubes. (A) a maximum intensity projection image of the confocal planes in (B) Composite confocal fluorescence image of an Aβ containing plaque at three different z heights from the signal of probes for neuronal cytosol (green), TiO2 nanotubes (red), Aβ (blue), and Tau (white); (C) live cell imaging of neurite collapse occurring within minutes after nanoparticle exposure, arrow points to a neurite that collapses in contact with the nanotubes; (D) neuronal plasma membrane labeled with CellMask™ deep red (CMDR) (green), high spatial resolution fluorescence image (stimulated emission depletion STED) of synapse damage and early plaque formation (white arrows) at the sites of damaged synapses (green, MEMBRANE) by the nanotubes (red, NANO) containing Aβ (blue, Aβ).
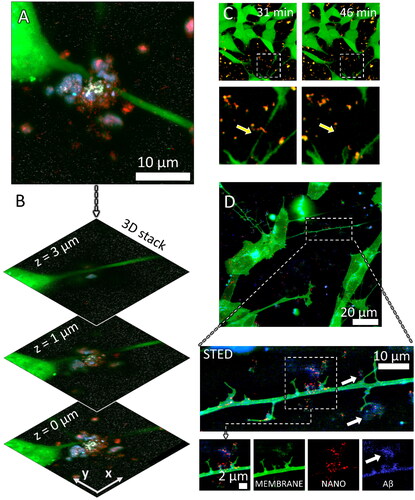
To gain a better understanding of these observations, we employed long-term time-lapse monitoring of neurites (, green) to discover that TiO2 nanotube aggregates (red) quickly colocalized with components of neuronal cytosol get (yellow, overlap of red and green), leading to discontinuation of a neurite within minutes after exposure as indicated by the yellow arrows in . Furthermore, high-resolution imaging using stimulated emission depletion (STED) microscopy revealed damaged synapses (green) where nanotubes (red) concentration was the highest (, white arrows). We also observed an early formation of a plaque near the neurite containing nanoparticles (red, , STED zoom-in NANO), neuronal plasma membrane components (green, MEMBRANE), and Aβ (blue, Aβ). Conversely, in the absence of TiO2 nanotubes, neurites of that same axon retained their integrity and no Aβ was detected in their proximity.
3.3. Diesel exhaust and iron oxide nanoparticles induce the most neurite shortening
Despite a strong epidemiological correlation between exposure to airborne particulate matter and the development of neurodegenerative diseases (Peeples Citation2020; Shi et al. Citation2020, Citation2021), the causal relationship between particulate matter in air pollution and Aβ plaque deposition with neurite degeneration in AD remains controversial (Budson Citation2020; Underwood Citation2017). To address this, we investigated whether various types of particulate matter commonly found in polluted air, including metal oxides and carbonaceous particles, could trigger the formation of Aβ plaques and neurite shortening as observed above for the TiO2 nanotubes.
For this purpose, we compared the effect of TiO2 nanotubes, CeO2, γ-Fe2O3, and diesel exhaust particles on neurite lengths and their number in neuronal cell culture (). We analyzed neurite lengths at 1 and 100 h after exposure to these materials using the Fiji ImageJ with NeuronJ plug-in, a program that can be used for semi-automated tracing of individual neurons (Popko et al. Citation2009). Representative images of chosen neurites, each connecting two distinct cells, are shown in violet in and in the supplement Section 5. The extracted distribution of neurite lengths across multiple images are plotted in the right-most column in .
Figure 3. Neurite length shortening from 1 h to 100 h after exposure to nanomaterial. We determined the lengths of neurites that connected two distinct neuronal cells at 1 and 100 h after exposure to nanomaterials using the NeuronJ plug-in. Representative images of neurites selected for analysis are shown in violet in the left (1 h) and Middle (100 h) column. The right-most column shows the distributions of neurite lengths 1 h (transparent) and 100 h (solid with black edges) after exposure to different nanomaterials: (A) control, (B) TiO2 nanotubes, (C) CeO2, (D) γ-Fe2O3, and (E) diesel exhaust particles exposed neurons. Median values of neurite lengths of all samples were significantly shortened for TiO2 nanotubes, γ-Fe2O3, and diesel exhaust particles (p < 0.001, Mann–Whitney U test).
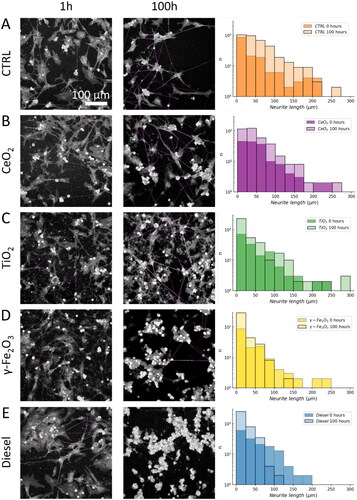
After 100 hours of exposure to nanomaterials, a significant reduction occurred in median length of neurites in neurons exposed to TiO2 nanotubes (from 59.4 μm to 39.8 μm, p < 0.001), γ-Fe2O3 (from 56.8 μm to 32.2 μm, p < 0.001), and diesel exhaust particles (from 60.7 μm to 29.6 μm, p < 0.001), compared to the same sample after 1-hour exposure. In contrast, CeO2 did not exhibit any reduction in length even after 100 h, compared the measurements taken an hour after exposure (Mann–Whitney U test applied due to the non-normal distribution of data). We observed the greatest effect in γ-Fe2O3 and diesel exhaust particles exposed neurons, where the median length decreased almost to a half of that observed in control. Alongside, the relative proportion of short neurites (<10 μm) dramatically increased, while there were almost no very long neurites (>100 μm) left (see histograms of number of neurites (n) versus neurite length in right).
3.4. Amyloid β (Aβ) plaques might have a protective role in neuronal survival after nanomaterial exposure
To gain insight into why some types of particulate matter (CeO2) do not affect neurite lengths and density to such an extent as other types (TiO2 nanotubes, γ-Fe2O3 and diesel exhaust particles) we analyzed the amount of Aβ deposited after nanomaterial exposure and assessed its colocalization with nanomaterial ().
Figure 4. Particulate matter triggered amyloid β-containing plaques in neuronal cell culture 2 days after exposure to a nanomaterial. Confocal images of differentiated SH-SY5Y live neuronal cells were stained with fluorescent CellMask™ deep red dye (membrane, green), unlabeled nanoparticles were detected via scattering (nanoparticles, red), Aβ plaques were immunostained with mouse monoclonal anti-β-amyloid antibody (santa cruzblue, # sc-53822; blue color) conjugated with ATTO490 LS, antibodies were added to living cells in 10 nM concentration. (A) control – unexposed neurons; (B) neurons exposed to TiO2 nanotubes; (C) CeO2 nanoparticles; (D) γ-Fe2O3 nanoparticles; (E) Diesel exhaust particles; (F) neurite length density (z axis), amount of Aβ (x axis), and proportion of nanoparticles colocalized with Aβ (y axis), each symbol represents values obtained from one ROI; (G) neurite density was calculated as total neurite length per total area (green bars), amount of Aβ was estimated as an integral of fluorescence intensity signal of Aβ antibody (blue bars), each symbol represents value obtained from one region of interest (ROI with a size of 400 μm × 400 μm, the height of the bar represents mean value, whiskers are standard deviation, long horizontal line is the median).
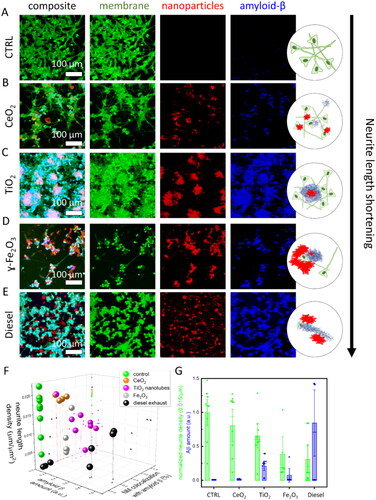
We first notice that all types of particles, except CeO2 nanoparticles (), trigger the release of Aβ (compare the height of blue bars in and the intensities of blue signal in ). TiO2 nanotubes triggered the formation of the large plaques containing neuronal plasma membrane (green), nanomaterial (red), and Aβ (blue), resulting in the overlapping signal of all three colors (, light pink). TiO2 nanotubes were also the most efficiently covered with Aβ, up to about 80% (pink spheres in ).
On the other hand, iron oxide and diesel exhaust particles colocalized less with Aβ, about 40% and less than 20% on average, respectively (gray and black spheres in ). In addition, diesel exhaust also trigged the highest release of Aβ (blue bar in ), but these aggregates colocalized with neurons rather than the nanoparticles themselves (; cyan color indicated colocalization of plasma membrane and Aβ signal). Furthermore, diesel exhaust particles caused massive neuronal death across the whole sample, as indicated by the presence of numerous rounded neurons with high membrane label intensity and no axons in . Such effects were also observed by others in a coculture system (Block et al. Citation2004; Seo et al. Citation2023). Interestingly, in the regions where the amount of Aβ deposits was high and diesel exhaust particles colocalized with Aβ deposits, the lengths of neurites were about ten times longer compared to regions with minimal Aβ deposition. Each such region is presented by a black sphere with higher Aβ amount values in . One can therefore see two groups of black spheres indicating the potential protective role of Aβ accumulation at the diesel exhaust particles. We speculate that this observation could potentially provide a mechanistic explanation to the conundrum observed in AD patients who possess a high burden of Aβ plaques but do not experience adverse symptoms (Gómez-Isla and Frosch Citation2022).
In , we show that exposure to nanomaterials that induce higher Aβ deposits (blue bars) generally leads to shortening of neurites (green bars) compared to the control. Despite inducing more Aβ deposits than γ-Fe2O3 nanoparticles, TiO2 nanotubes cause less neurite loss. This discrepancy might be attributed to the property of TiO2 nanotubes to bind more Aβ, thus again highlighting a protective role of Aβ deposition surrounding the nanomaterial.
These findings suggest that Aβ plaque formation does not necessarily coincide with neurite loss, which could explain why not all individuals with plaques develop AD (Gómez-Isla and Frosch Citation2022), as well as why numerous clinical trials directed at reducing Aβ have failed in humans, despite their success in genetic mouse models of AD. Namely, it has been proposed that the reason for the failures of clinical trials in humans is that mouse models only exhibit plaques without other hallmarks of the disease, whereas AD patients develop plaques, tau deposits, and neuronal degeneration (Busche and Hyman Citation2020). Therefore, the induction of Aβ and tau plaque formation accompanied by neurite atrophy and neuronal degeneration through particulate matter exposure might represent a more relevant model of AD compared to existing genetically induced mouse models.
3.5. Conclusion
We here report the formation of extracellular Aβ plaques, resembling those found in AD, after exposure of an in vitro neuronal cell culture to engineered anatase TiO2 nanotubes, which we previously showed to trigger chronic lung inflammation and nanoparticle-containing organic debris formation in mice (Danielsen et al. Citation2020; Kokot et al. Citation2020). These plaques closely resemble those in mice and AD patients observed by He et al. (He et al. Citation2018), which were induced in wild-type mice via intracerebral injection of Tau. Here we show that similar plaques can be triggered with exposure to some nanomaterials. We exposed SH-SY5Y-derived human neurons to a very high dose of engineered anatase TiO2 nanotubes and monitored the formation of Aβ and Tau-containing plaques 24–48 h later. TiO2 nanotubes caused neurites (i.e. cellular protrusions extending from neurons) to shorten (median length decreased from 59.4 μm to 39.8 μm, p < 0.001) and atrophy (), causing a decrease in neurite lengths (). Oppositely, CeO2 nanoparticles do not cause neurite shortening () and do not induce Aβ plaque formation, which is consistent with their beneficial effect observed in traumatic brain injury (Fiorani et al. Citation2015; Gagnon and Fromm Citation2015).
We also tested other nanomaterials that can be found in polluted air, diesel exhaust and iron oxide particles, which are associated with cognitive decline in areas with high air pollution (Underwood Citation2017). Iron oxide (γ-Fe2O3 (from 56.8 μm to 32.2 μm, p < 0.001)) and diesel exhaust particles (from 60.7 μm to 29.6 μm, p < 0.001), decreased neurite lengths the most () and induced the accumulation of Aβ mainly in apoptotic neurons, as suggested by their rounded-up morphology, typical of apoptotic cells. On the other hand, TiO2 nanotubes induced the accumulation of Aβ in extracellular space often co-localized with the nanoparticles, suggesting that aggregation of the nanoparticles with Aβ might be a protective mechanism that ‘hides’ the material’s surface and prevents its harmful contact with neurons, thus safeguarding neurites.
Overall, this study identifies how certain nanoparticles relevant to particulate matter in ambient air pollution might contribute to AD by triggering hallmarks of the disease: shortening of neurites, formation of Aβ plaques and, in the case of TiO2, accumulation of Tau protein within these plaques. Furthermore, we speculate that Aβ plaque colocalization with a nanomaterial could potentially protect neurites. While the exact mechanism behind this effect remains to be explained, it is possible that exposure to different types of ambient air pollution could explain earlier observations of individuals experiencing a high burden of plaques yet no adverse cognitive symptoms (Gómez-Isla and Frosch Citation2022).
Authors contributions
Conceptualization and design: A.S., T.K., I.U. and J.Š.; data collection: A.S.; analysis and interpretation of the data: A.S., T.K., I.U. and J.Š.; the drafting of the paper: A.S., T.K.; revising the paper critically for intellectual content: A.S., L.M.A.C, V. M., S.K., J.P., U.V., S.Z.N., I.U., T.K., and J.Š.; All authors gave the final approval of the version to be published; all authors agree to be accountable for all aspects of the work.
Supplemental Material
Download MS Word (104.9 MB)Acknowledgments
Louise Gren, Polona Umek, and Martí Busquets Fité are acknowledged for providing TEM images of diesel exhaust, TiO2, and CeO2 particles, respectively.
Disclosure statement
No potential conflict of interest was reported by the author(s).
Data availability statement
The authors confirm that the data supporting the findings of this study are available upon request within the article and its supplementary materials.
Additional information
Funding
References
- Agholme, L., T. Lindström, K. Kågedal, J. Marcusson, and M. Hallbeck. 2010. “An in Vitro Model for Neuroscience: Differentiation of SH-SY5Y Cells into Cells with Morphological and Biochemical Characteristics of Mature Neurons.” Journal of Alzheimer’s Disease 20 (4): 1069–1082. https://doi.org/10.3233/JAD-2010-091363.
- Bai, B., D. Vanderwall, Y. Li, X. Wang, S. Poudel, H. Wang, K. K. Dey, P.-C. Chen, K. Yang, and J. Peng. 2021. “Proteomic Landscape of Alzheimer’s Disease: Novel Insights into Pathogenesis and Biomarker Discovery.” Molecular Neurodegeneration 16 (1): 55. https://doi.org/10.1186/s13024-021-00474-z.
- Baj, J., B. Kowalska, W. Flieger, E. Radzikowska-Büchner, A. Forma, M. Czeczelewski, P. Kędzierawski, et al. 2023. “Assessment of the Concentration of 51 Elements in the Liver and in Various Parts of the Human Brain—Profiling of the Mineral Status.” Nutrients 15 (12): 2799. https://doi.org/10.3390/nu15122799.
- Beem, E., and M. S. Segal. 2013. “Evaluation of Stability and Sensitivity of Cell Fluorescent Labels When Used for Cell Migration.” Journal of Fluorescence 23 (5): 975–987. https://doi.org/10.1007/s10895-013-1224-8.
- Bendtsen, K. M., L. Gren, V. B. Malmborg, P. C. Shukla, M. Tunér, Y. J. Essig, A. M. Krais, et al. 2020. “Particle Characterization and Toxicity in C57BL/6 Mice following Instillation of Five Different Diesel Exhaust Particles Designed to Differ in Physicochemical Properties.” Particle and Fibre Toxicology 17 (1): 38. https://doi.org/10.1186/s12989-020-00369-9.
- Blennow, K., M. J. de Leon, and H. Zetterberg. 2006. “Alzheimer’s Disease.” Lancet 368 (9533): 387–403. https://doi.org/10.1016/S0140-6736(06)69113-7.
- Block, M. L., X. Wu, Z. Pei, G. Li, T. Wang, L. Qin, B. Wilson, J. Yang, J. S. Hong, and B. Veronesi. 2004. “Nanometer Size Diesel Exhaust Particles Are Selectively Toxic to Dopaminergic Neurons: The Role of Microglia, Phagocytosis, and NADPH Oxidase.” FASEB Journal 18 (13): 1618–1620. https://doi.org/10.1096/fj.04-1945fje.
- Brandt, R., J. Léger, and G. Lee. 1995. “Interaction of Tau with the Neural Plasma Membrane Mediated by Tau’s Amino-Terminal Projection Domain.” The Journal of Cell Biology 131 (5): 1327–1340. https://doi.org/10.1083/jcb.131.5.1327.
- Budson, A. E. 2020. “Does air Pollution Cause Alzheimer’s Disease?” Harvard Health Blog. https://www.health.harvard.edu/blog/does-air-pollution-cause-alzheimers-disease-2020072320627
- Busche, M. A., and B. T. Hyman. 2020. “Synergy between Amyloid-β and Tau in Alzheimer’s Disease.” Nature Neuroscience 23 (10): 1183–1193. https://doi.org/10.1038/s41593-020-0687-6.
- Calderón-Garcidueñas, L., and A. Ayala. 2022. “Air Pollution, Ultrafine Particles, and Your Brain: Are Combustion Nanoparticle Emissions and Engineered Nanoparticles Causing Preventable Fatal Neurodegenerative Diseases and Common Neuropsychiatric Outcomes?” Environmental Science & Technology 56 (11): 6847–6856. https://doi.org/10.1021/acs.est.1c04706.
- Calderón-Garcidueñas, L., A. Herrera-Soto, N. Jury, B. A. Maher, A. González-Maciel, R. Reynoso-Robles, P. Ruiz-Rudolph, B. van Zundert, and L. Varela-Nallar. 2020. “Reduced Repressive Epigenetic Marks, Increased DNA Damage and Alzheimer’s Disease Hallmarks in the Brain of Humans and Mice Exposed to Particulate Urban Air Pollution.” Environmental Research 183: 109226. https://doi.org/10.1016/j.envres.2020.109226.
- Carey, I. M., H. R. Anderson, R. W. Atkinson, S. D. Beevers, D. G. Cook, D. P. Strachan, D. Dajnak, J. Gulliver, and F. J. Kelly. 2018. “Are Noise and Air Pollution Related to the Incidence of Dementia? A Cohort Study in London, England.” BMJ Open 8 (9): e022404. https://doi.org/10.1136/bmjopen-2018-022404.
- Couzin-Frankel, J. 2023. “Promising Alzheimer’s Therapies Shrink Brains.” Science (New York, N.Y.) 380 (6640): 19–19. https://doi.org/10.1126/science.adi1220.
- Danielsen, P. H., K. B. Knudsen, J. Štrancar, P. Umek, T. Koklič, M. Garvas, E. Vanhala, et al. 2020. “Effects of Physicochemical Properties of TiO2 Nanomaterials for Pulmonary Inflammation, Acute Phase Response and Alveolar Proteinosis in Intratracheally Exposed Mice.” Toxicology and Applied Pharmacology 386: 114830. https://doi.org/10.1016/j.taap.2019.114830.
- De La-Rocque, S., E. Moretto, I. Butnaru, and G. Schiavo. 2021. “Knockin’ on Heaven’s Door: Molecular Mechanisms of Neuronal Tau Uptake.” Journal of Neurochemistry 156 (5): 563–588. https://doi.org/10.1111/jnc.15144.
- Debia, M., C. Couture, P.-E. Njanga, E. Neesham-Grenon, G. Lachapelle, H. Coulombe, S. Hallé, and S. Aubin. 2017. “Diesel Engine Exhaust Exposures in Two Underground Mines.” International Journal of Mining Science and Technology 27 (4): 641–645. https://doi.org/10.1016/j.ijmst.2017.05.011.
- Drew, L. 2018. “An Age-Old Story of Dementia.” Nature 559 (7715): S2–S3. https://doi.org/10.1038/d41586-018-05718-5.
- Drummond, E., S. Nayak, A. Faustin, G. Pires, R. A. Hickman, M. Askenazi, M. Cohen, et al. 2017. “Proteomic Differences in Amyloid Plaques in Rapidly Progressive and Sporadic Alzheimer’s Disease.” Acta Neuropathologica 133 (6): 933–954. https://doi.org/10.1007/s00401-017-1691-0.
- Du, Z., M. Li, J. Ren, and X. Qu. 2021. “Current Strategies for Modulating Aβ Aggregation with Multifunctional Agents.” Accounts of Chemical Research 54 (9): 2172–2184. https://doi.org/10.1021/acs.accounts.1c00055.
- Eftekharzadeh, B., B. T. Hyman, and S. Wegmann. 2016. “Structural Studies on the Mechanism of Protein Aggregation in Age Related Neurodegenerative Diseases.” Mechanisms of Ageing and Development 156: 1–13. https://doi.org/10.1016/j.mad.2016.03.001.
- Falke, E., J. Nissanov, T. W. Mitchell, D. A. Bennett, J. Q. Trojanowski, and S. E. Arnold. 2003. “Subicular Dendritic Arborization in Alzheimer’s Disease Correlates with Neurofibrillary Tangle Density.” The American Journal of Pathology 163 (4): 1615–1621. https://doi.org/10.1016/S0002-9440(10)63518-3.
- Finnegan, M. E., N. P. Visanji, I. Romero-Canelon, E. House, S. Rajan, J. F. W. Mosselmans, L.-N. Hazrati, J. Dobson, and J. F. Collingwood. 2019. “Synchrotron XRF Imaging of Alzheimer’s Disease Basal Ganglia Reveals Linear Dependence of High-Field Magnetic Resonance Microscopy on Tissue Iron Concentration.” Journal of Neuroscience Methods 319: 28–39. https://doi.org/10.1016/j.jneumeth.2019.03.002.
- Fiorani, L., M. Passacantando, S. Santucci, S. Di Marco, S. Bisti, and R. Maccarone. 2015. “Cerium Oxide Nanoparticles Reduce Microglial Activation and Neurodegenerative Events in Light Damaged Retina.” PLOS One 10 (10): e0140387. https://doi.org/10.1371/journal.pone.0140387.
- Gagnon, J., and K. M. Fromm. 2015. “Toxicity and Protective Effects of Cerium Oxide Nanoparticles (Nanoceria) Depending on Their Preparation Method, Particle Size, Cell Type, and Exposure Route.” European Journal of Inorganic Chemistry 2015 (27): 4510–4517. https://doi.org/10.1002/ejic.201500643.
- Glenner, G. G., and C. W. Wong. 1984. “Alzheimer’s Disease: initial Report of the Purification and Characterization of a Novel Cerebrovascular Amyloid Protein.” Biochemical and Biophysical Research Communications 120 (3): 885–890. https://doi.org/10.1016/s0006-291x(84)80190-4.
- Gómez-Isla, T., and M. P. Frosch. 2022. “Lesions without Symptoms: Understanding Resilience to Alzheimer Disease Neuropathological Changes.” Nature Reviews Neurology 18 (6): 323–332. https://doi.org/10.1038/s41582-022-00642-9.
- Gravina, S. A., L. Ho, C. B. Eckman, K. E. Long, L. Otvos, L. H. Younkin, N. Suzuki, and S. G. Younkin. 1995. “Amyloid Beta Protein (a Beta) in Alzheimer’s Disease Brain. Biochemical and Immunocytochemical Analysis with Antibodies Specific for Forms Ending at a Beta 40 or a Beta 42(43).” The Journal of Biological Chemistry 270 (13): 7013–7016. https://doi.org/10.1074/jbc.270.13.7013.
- Gren, L., V. B. Malmborg, N. R. Jacobsen, P. C. Shukla, K. M. Bendtsen, A. C. Eriksson, Y. J. Essig, et al. 2020. “Effect of Renewable Fuels and Intake O2 Concentration on Diesel Engine Emission Characteristics and Reactive Oxygen Species (ROS) Formation.” Atmosphere 11 (6): 641. https://doi.org/10.3390/atmos11060641.
- Gu, L., and Z. Guo. 2013. “Alzheimer’s Aβ42 and Aβ40 Peptides Form Interlaced Amyloid Fibrils.” Journal of Neurochemistry 126 (3): 305–311. https://doi.org/10.1111/jnc.12202.
- He, Z., J. L. Guo, J. D. McBride, S. Narasimhan, H. Kim, L. Changolkar, B. Zhang, et al. 2018. “Amyloid-β Plaques Enhance Alzheimer’s Brain Tau-Seeded Pathologies by Facilitating Neuritic Plaque Tau Aggregation.” Nature Medicine 24 (1): 29–38. https://doi.org/10.1038/nm.4443.
- Iwatsubo, T., D. M. Mann, A. Odaka, N. Suzuki, and Y. Ihara. 1995. “Amyloid Beta Protein (a Beta) Deposition: A Beta 42(43) Precedes a Beta 40 in Down Syndrome.” Annals of Neurology 37 (3): 294–299. https://doi.org/10.1002/ana.410370305.
- Iwatsubo, T., A. Odaka, N. Suzuki, H. Mizusawa, N. Nukina, and Y. Ihara. 1994. “Visualization of a Beta 42(43) and a Beta 40 in Senile Plaques with End-Specific a Beta Monoclonals: Evidence That an Initially Deposited Species is a Beta 42(43).” Neuron 13 (1): 45–53. https://doi.org/10.1016/0896-6273(94)90458-8.
- Karch, C. M., A. T. Jeng, and A. M. Goate. 2012. “Extracellular Tau Levels Are Influenced by Variability in Tau That is Associated with Tauopathies.” The Journal of Biological Chemistry 287 (51): 42751–42762. https://doi.org/10.1074/jbc.M112.380642.
- Karran, E., M. Mercken, and B. D. Strooper. 2011. “The Amyloid Cascade Hypothesis for Alzheimer’s Disease: An Appraisal for the Development of Therapeutics.” Nature Reviews Drug Discovery 10 (9): 698–712. https://doi.org/10.1038/nrd3505.
- Kiani Shabestari, S., S. Morabito, E. P. Danhash, A. McQuade, J. R. Sanchez, E. Miyoshi, J. P. Chadarevian, et al. 2022. “Absence of Microglia Promotes Diverse Pathologies and Early Lethality in Alzheimer’s Disease Mice.” Cell Reports 39 (11): 110961. https://doi.org/10.1016/j.celrep.2022.110961.
- Kokot, H., B. Kokot, A. Sebastijanović, C. Voss, R. Podlipec, P. Zawilska, T. Berthing, et al. 2020. “Prediction of Chronic Inflammation for Inhaled Particles: The Impact of Material Cycling and Quarantining in the Lung Epithelium.” Advanced Materials 32 (47): 2003913. https://doi.org/10.1002/adma.202003913.
- Kovalevich, J., and D. Langford. 2013. “Considerations for the Use of SH-SY5Y Neuroblastoma Cells in Neurobiology.” Methods in Molecular Biology 1078: 9–21. https://doi.org/10.1007/978-1-62703-640-5_2.
- Kovalevich, J., M. Santerre, and D. Langford. 2021. “Considerations for the Use of SH-SY5Y Neuroblastoma Cells in Neurobiology.” Methods in Molecular Biology 2311: 9–23. https://doi.org/10.1007/978-1-0716-1437-2_2.
- Kralj, S., and D. Makovec. 2014. “The Chemically Directed Assembly of Nanoparticle Clusters from Superparamagnetic Iron-Oxide Nanoparticles.” RSC Advances 4 (25): 13167–13171. https://doi.org/10.1039/c4ra00776j.
- Kralj, S., M. Rojnik, R. Romih, M. Jagodič, J. Kos, and D. Makovec. 2012. “Effect of Surface Charge on the Cellular Uptake of Fluorescent Magnetic Nanoparticles.” Journal of Nanoparticle Research 14 (10): 1151. https://doi.org/10.1007/s11051-012-1151-7.
- Le Bras, A. 2022. “New Insights into the Origin of Amyloid Plaques.” Lab Animal 51 (7): 187–187. https://doi.org/10.1038/s41684-022-01007-x.
- Lee, J.-H., D.-S. Yang, C. N. Goulbourne, E. Im, P. Stavrides, A. Pensalfini, H. Chan, et al. 2022. “Faulty Autolysosome Acidification in Alzheimer’s Disease Mouse Models Induces Autophagic Build-up of Aβ in Neurons, Yielding Senile Plaques.” Nature Neuroscience 25 (6): 688–701. https://doi.org/10.1038/s41593-022-01084-8.
- Leng, F., and P. Edison. 2021. “Neuroinflammation and Microglial Activation in Alzheimer Disease: Where Do We Go from Here?” Nature Reviews Neurology 17 (3): 157–172. https://doi.org/10.1038/s41582-020-00435-y.
- Liu, Y., M. Nguyen, A. Robert, and B. Meunier. 2019. “Metal Ions in Alzheimer’s Disease: A Key Role or Not?” Accounts of Chemical Research 52 (7): 2026–2035. https://doi.org/10.1021/acs.accounts.9b00248.
- Livingston, G., J. Huntley, A. Sommerlad, D. Ames, C. Ballard, S. Banerjee, C. Brayne, et al. 2020. “Dementia Prevention, Intervention, and Care: 2020 Report of the Lancet Commission.” Lancet 396 (10248): 413–446. https://doi.org/10.1016/S0140-6736(20)30367-6.
- Lopez-Suarez, L., S. A. Awabdh, X. Coumoul, and C. Chauvet. 2022. “The SH-SY5Y Human Neuroblastoma Cell Line, a Relevant in Vitro Cell Model for Investigating Neurotoxicology in Human: Focus on Organic Pollutants.” Neurotoxicology 92: 131–155. https://doi.org/10.1016/j.neuro.2022.07.008.
- Maher, B. A. 2019. “Airborne Magnetite- and Iron-Rich Pollution Nanoparticles: Potential Neurotoxicants and Environmental Risk Factors for Neurodegenerative Disease, Including Alzheimer’s Disease.” Journal of Alzheimer’s Disease 71 (2): 361–375. https://doi.org/10.3233/JAD-190204.
- Maher, B. A., I. A. M. Ahmed, V. Karloukovski, D. A. MacLaren, P. G. Foulds, D. Allsop, D. M. A. Mann, R. Torres-Jardón, and L. Calderon-Garciduenas. 2016. “Magnetite Pollution Nanoparticles in the Human Brain.” Proceedings of the National Academy of Sciences 113 (39): 10797–10801. https://doi.org/10.1073/pnas.1605941113.
- Mak, K., F. Yang, H. V. Vinters, S. A. Frautschy, and G. M. Cole. 1994. “Polyclonals to Beta-Amyloid(1-42) Identify Most Plaque and Vascular Deposits in Alzheimer Cortex, but Not Striatum.” Brain Research 667 (1): 138–142. https://doi.org/10.1016/0006-8993(94)91725-6.
- Marciniak, E., A. Leboucher, E. Caron, T. Ahmed, A. Tailleux, J. Dumont, T. Issad, et al. 2017. “Tau Deletion Promotes Brain Insulin Resistance.” The Journal of Experimental Medicine 214 (8): 2257–2269. https://doi.org/10.1084/jem.20161731.
- Martin, E.-R., J. Gandawijaya, and A. Oguro-Ando. 2022. “A Novel Method for Generating Glutamatergic SH-SY5Y Neuron-like Cells Utilizing B-27 Supplement.” Frontiers in Pharmacology 13: 943627. https://doi.org/10.3389/fphar.2022.943627.
- Martorana, A., and G. Koch. 2014. “Is Dopamine Involved in Alzheimer’s Disease?” Frontiers in Aging Neuroscience 6: 252. https://doi.org/10.3389/fnagi.2014.00252.
- Masters, C. L., R. Bateman, K. Blennow, C. C. Rowe, R. A. Sperling, and J. L. Cummings. 2015. “Alzheimer’s Disease.” Nature Reviews Disease Primers 1 (1): 15056. https://doi.org/10.1038/nrdp.2015.56.
- Mudher, A., M. Colin, S. Dujardin, M. Medina, I. Dewachter, S. M. Alavi Naini, E.-M. Mandelkow, et al. 2017. “What is the Evidence That Tau Pathology Spreads through Prion-like Propagation?” Acta Neuropathologica Communications 5 (1): 99. https://doi.org/10.1186/s40478-017-0488-7.
- Nemec, S., and S. Kralj. 2021. “A Versatile Interfacial Coassembly Method for Fabrication of Tunable Silica Shells with Radially Aligned Dual Mesopores on Diverse Magnetic Core Nanoparticles.” ACS Applied Materials & Interfaces 13 (1): 1883–1894. https://doi.org/10.1021/acsami.0c17863.
- Nemec, S., S. Kralj, C. Wilhelm, A. Abou-Hassan, M.-P. Rols, and J. Kolosnjaj-Tabi. 2020. “Comparison of Iron Oxide Nanoparticles in Photothermia and Magnetic Hyperthermia: Effects of Clustering and Silica Encapsulation on Nanoparticles’ Heating Yield.” Applied Sciences 10 (20): 7322. https://doi.org/10.3390/app10207322.
- Nobili, A., E. C. Latagliata, M. T. Viscomi, V. Cavallucci, D. Cutuli, G. Giacovazzo, P. Krashia, et al. 2017. “Dopamine Neuronal Loss Contributes to Memory and Reward Dysfunction in a Model of Alzheimer’s Disease.” Nature Communications 8 (1): 14727. https://doi.org/10.1038/ncomms14727.
- Oakley, H., S. L. Cole, S. Logan, E. Maus, P. Shao, J. Craft, A. Guillozet-Bongaarts, et al. 2006. “Intraneuronal β-Amyloid Aggregates, Neurodegeneration, and Neuron Loss in Transgenic Mice with Five Familial Alzheimer’s Disease Mutations: Potential Factors in Amyloid Plaque Formation.” The Journal of Neuroscience 26 (40): 10129–10140. https://doi.org/10.1523/JNEUROSCI.1202-06.2006.
- Padmanabhan, P., A. Kneynsberg, E. Cruz, R. Amor, J.-B. Sibarita, and J. Götz. 2022. “Single-Molecule Imaging Reveals Tau Trapping at Nanometer-Sized Dynamic Hot Spots near the Plasma Membrane That Persists after Microtubule Perturbation and Cholesterol Depletion.” The EMBO Journal 41 (19): e111265. https://doi.org/10.15252/embj.2022111265.
- Peeples, L. 2020. “How Air Pollution Threatens Brain Health.” Proceedings of the National Academy of Sciences 117 (25): 13856–13860. https://doi.org/10.1073/pnas.2008940117.
- Pensalfini, A., R. Albay, S. Rasool, J. W. Wu, A. Hatami, H. Arai, L. Margol, et al. 2014. “Intracellular Amyloid and the Neuronal Origin of Alzheimer Neuritic Plaques.” Neurobiology of Disease 71: 53–61. https://doi.org/10.1016/j.nbd.2014.07.011.
- Peters, R. J. B., A. G. Oomen, G. van Bemmel, L. van Vliet, A. K. Undas, S. Munniks, R. L. A. W. Bleys, P. C. Tromp, W. Brand, and M. van der Lee. 2020. “Silicon Dioxide and Titanium Dioxide Particles Found in Human Tissues.” Nanotoxicology 14 (3): 420–432. https://doi.org/10.1080/17435390.2020.1718232.
- Peters, R. J. B., G. van Bemmel, Z. Herrera-Rivera, H. P. F. G. Helsper, H. J. P. Marvin, S. Weigel, P. C. Tromp, A. G. Oomen, A. G. Rietveld, and H. Bouwmeester. 2014. “Characterization of Titanium Dioxide Nanoparticles in Food Products: analytical Methods to Define Nanoparticles.” Journal of Agricultural and Food Chemistry 62 (27): 6285–6293. https://doi.org/10.1021/jf5011885.
- Piller, C. 2023. “Report on Trial Death Stokes Alzheimer’s Drug Fears.” Science 380 (6641): 122–123. https://doi.org/10.1126/science.adi2242.
- Plascencia-Villa, G., A. Ponce, J. F. Collingwood, M. J. Arellano-Jiménez, X. Zhu, J. T. Rogers, I. Betancourt, M. José-Yacamán, and G. Perry. 2016. “High-Resolution Analytical Imaging and Electron Holography of Magnetite Particles in Amyloid Cores of Alzheimer’s Disease.” Scientific Reports 6 (1): 24873. https://doi.org/10.1038/srep24873.
- Popko, J., A. Fernandes, D. Brites, and L. M. Lanier. 2009. “Automated Analysis of NeuronJ Tracing Data.” Cytometry A 75 (4): 371–376. https://doi.org/10.1002/cyto.a.20660.
- Riegerová, P., J. Brejcha, D. Bezděková, T. Chum, E. Mašínová, N. Čermáková, S. V. Ovsepian, M. Cebecauer, and M. Štefl. 2021. “Expression and Localization of AβPP in SH-SY5Y Cells Depends on Differentiation State.” Journal of Alzheimer’s Disease 82 (2): 485–491. https://doi.org/10.3233/JAD-201409.
- Rothmann, M. H., P. Møller, Y. J. Essig, L. Gren, V. B. Malmborg, M. Tunér, J. Pagels, A. M. Krais, and M. Roursgaard. 2023. “Genotoxicity by Rapeseed Methyl Ester and Hydrogenated Vegetable Oil Combustion Exhaust Products in Lung Epithelial (A549) Cells.” Mutagenesis 38 (4): 238–249. https://doi.org/10.1093/mutage/gead016.
- Sala, A., S. P. Caminiti, L. Presotto, A. Pilotto, C. Liguori, A. Chiaravalloti, V. Garibotto, et al. 2021. “In Vivo Human Molecular Neuroimaging of Dopaminergic Vulnerability along the Alzheimer’s Disease Phases.” Alzheimer’s Research & Therapy 13 (1): 187. https://doi.org/10.1186/s13195-021-00925-1.
- Scheltens, P., K. Blennow, M. M. B. Breteler, B. de Strooper, G. B. Frisoni, S. Salloway, and W. M. Van der Flier. 2016. “Alzheimer’s Disease.” Lancet 388 (10043): 505–517. https://doi.org/10.1016/S0140-6736(15)01124-1.
- Schraufnagel, D. E. 2020. “The Health Effects of Ultrafine Particles.” Experimental & Molecular Medicine 52 (3): 311–317. https://doi.org/10.1038/s12276-020-0403-3.
- Seo, S., M. Jang, H. Kim, J. H. Sung, N. Choi, K. Lee, and H. N. Kim. 2023. “Neuro-Glia-Vascular-on-a-Chip System to Assess Aggravated Neurodegeneration via Brain Endothelial Cells upon Exposure to Diesel Exhaust Particles.” Advanced Functional Materials 33 (12): 2210123. https://doi.org/10.1002/adfm.202210123.
- Serrano-Pozo, A., M. L. Mielke, A. Muzitansky, T. Gómez-Isla, J. H. Growdon, B. J. Bacskai, R. A. Betensky, M. P. Frosch, and B. T. Hyman. 2012. “Stable Size Distribution of Amyloid Plaques over the Course of Alzheimer Disease.” Journal of Neuropathology and Experimental Neurology 71 (8): 694–701. https://doi.org/10.1097/NEN.0b013e31825e77de.
- Sheikh, H. A., P. Y. Tung, E. Ringe, and R. J. Harrison. 2022. “Magnetic and Microscopic Investigation of Airborne Iron Oxide Nanoparticles in the London Underground.” Scientific Reports 12 (1): 20298. https://doi.org/10.1038/s41598-022-24679-4.
- Shi, L., K. Steenland, H. Li, P. Liu, Y. Zhang, R. H. Lyles, W. J. Requia, et al. 2021. “A National Cohort Study (2000–2018) of Long-Term Air Pollution Exposure and Incident Dementia in Older Adults in the United States.” Nature Communications 12 (1): 6754. https://doi.org/10.1038/s41467-021-27049-2.
- Shi, L., X. Wu, M. D. Yazdi, D. Braun, Y. A. Awad, Y. Wei, P. Liu, et al. 2020. “Long-Term Effects of PM2·5 on Neurological Disorders in the American Medicare Population: A Longitudinal Cohort Study.” The Lancet Planetary Health 4 (12): e557–e565. https://doi.org/10.1016/S2542-5196(20)30227-8.
- Song, B., J. Liu, X. Feng, L. Wei, and L. Shao. 2015. “A Review on Potential Neurotoxicity of Titanium Dioxide Nanoparticles.” Nanoscale Research Letters 10 (1): 342. https://doi.org/10.1186/s11671-015-1042-9.
- Sotiropoulos, I., M.-C. Galas, J. M. Silva, E. Skoulakis, S. Wegmann, M. B. Maina, D. Blum, et al. 2017. “Atypical, Non-Standard Functions of the Microtubule Associated Tau Protein.” Acta Neuropathologica Communications 5 (1): 91. https://doi.org/10.1186/s40478-017-0489-6.
- Suarez, A. E., and J. M. Ondov. 2002. “Ambient Aerosol Concentrations of Elements Resolved by Size and by Source: Contributions of Some Cytokine-Active Metals from Coal- and Oil-Fired Power Plants.” Energy & Fuels 16 (3): 562–568. https://doi.org/10.1021/ef010170c.
- Suárez-Calvet, M., T. K. Karikari, N. J. Ashton, J. Lantero Rodríguez, M. Milà-Alomà, J. D. Gispert, G. Salvadó, et al. 2020. “Novel Tau Biomarkers Phosphorylated at T181, T217 or T231 Rise in the Initial Stages of the Preclinical Alzheimer’s Continuum When Only Subtle Changes in Aβ Pathology Are Detected.” EMBO Molecular Medicine 12 (12): e12921. https://doi.org/10.15252/emmm.202012921.
- Tadic, M., S. Kralj, M. Jagodic, D. Hanzel, and D. Makovec. 2014. “Magnetic Properties of Novel Superparamagnetic Iron Oxide Nanoclusters and Their Peculiarity under Annealing Treatment.” Applied Surface Science 322: 255–264. https://doi.org/10.1016/j.apsusc.2014.09.181.
- Tahirbegi, B., A. J. Magness, M. E. Piersimoni, T. Knöpfel, K. R. Willison, D. R. Klug, and L. Ying. 2020. “A Novel Aβ40 Assembly at Physiological Concentration.” Scientific Reports 10 (1): 9477. https://doi.org/10.1038/s41598-020-66373-3.
- Takahashi, M., H. Miyata, F. Kametani, T. Nonaka, H. Akiyama, S. Hisanaga, and M. Hasegawa. 2015. “Extracellular Association of APP and Tau Fibrils Induces Intracellular Aggregate Formation of Tau.” Acta Neuropathologica 129 (6): 895–907. https://doi.org/10.1007/s00401-015-1415-2.
- Titulaer, J., C. Björkholm, K. Feltmann, T. Malmlöf, D. Mishra, C. Bengtsson Gonzales, B. Schilström, and Å. Konradsson-Geuken. 2021. “The Importance of Ventral Hippocampal Dopamine and Norepinephrine in Recognition Memory.” Frontiers in Behavioral Neuroscience 15: 667244. https://doi.org/10.3389/fnbeh.2021.667244.
- Umek, P., R. C. Korosec, B. Jancar, R. Dominko, and D. Arcon. 2007. “The Influence of the Reaction Temperature on the Morphology of Sodium Titanate 1D Nanostructures and Their Thermal Stability.” Journal of Nanoscience and Nanotechnology 7 (10): 3502–3508. https://doi.org/10.1166/jnn.2007.838.
- Underwood, E. 2017. “The Polluted Brain.” Science 355 (6323): 342–345. https://doi.org/10.1126/science.355.6323.342.
- Urbančič, I., M. Garvas, B. Kokot, H. Majaron, P. Umek, H. Cassidy, M. Škarabot, et al. 2018. “Nanoparticles Can Wrap Epithelial Cell Membranes and Relocate Them across the Epithelial Cell Layer.” Nano Letters 18 (8): 5294–5305. https://doi.org/10.1021/acs.nanolett.8b02291.
- Viskup, R., C. Wolf, and W. Baumgartner. 2020. Major Chemical Elements in Soot and Particulate Matter Exhaust Emissions Generated from in-Use Diesel Engine Passenger Vehicles. London, UK: IntechOpen Limited. https://doi.org/10.5772/intechopen.90452.
- Walker, L. C. 2020. “Aβ Plaques.” Free Neuropathology 1: 31. https://doi.org/10.17879/freeneuropathology-2020-3025.
- Weichenthal, S., L. Pinault, T. Christidis, R. T. Burnett, J. R. Brook, Y. Chu, D. L. Crouse, et al. 2022. “How Low Can You Go? Air Pollution Affects Mortality at Very Low Levels.” Science Advances 8 (39): eabo3381. https://doi.org/10.1126/sciadv.abo3381.
- Wilson, D. M., M. R. Cookson, L. Van Den Bosch, H. Zetterberg, D. M. Holtzman, and I. Dewachter. 2023. “Hallmarks of Neurodegenerative Diseases.” Cell 186 (4): 693–714. https://doi.org/10.1016/j.cell.2022.12.032.
- Xie, H., L. Hu, and G. Li. 2010. “SH-SY5Y Human Neuroblastoma Cell Line: In Vitro Cell Model of Dopaminergic Neurons in Parkinson’s Disease.” Chinese Medical Journal 123 (8): 1086–1092.
- Xiong, F., W. Ge, and C. Ma. 2019. “Quantitative Proteomics Reveals Distinct Composition of Amyloid Plaques in Alzheimer’s Disease.” Alzheimer’s & Dementia 15 (3): 429–440. https://doi.org/10.1016/j.jalz.2018.10.006.
- Younan, D., A. J. Petkus, K. F. Widaman, X. Wang, R. Casanova, M. A. Espeland, M. Gatz, et al. 2020. “Particulate Matter and Episodic Memory Decline Mediated by Early Neuroanatomic Biomarkers of Alzheimer’s Disease.” Brain: a Journal of Neurology 143 (1): 289–302. https://doi.org/10.1093/brain/awz348.
- Ze, Y., L. Sheng, X. Zhao, J. Hong, X. Ze, X. Yu, X. Pan, et al. 2014. “TiO2 Nanoparticles Induced Hippocampal Neuroinflammation in Mice.” PLOS One 9 (3): e92230. https://doi.org/10.1371/journal.pone.0092230.
- Zhang, H., D. Yu, S. Liu, C. Liu, Z. Liu, J. Ren, and X. Qu. 2022. “NIR-II Hydrogen-Bonded Organic Frameworks (HOFs) Used for Target-Specific Amyloid-β Photooxygenation in an Alzheimer’s Disease Model.” Angewandte Chemie 61 (2): e202109068. https://doi.org/10.1002/anie.202109068.
- Zhang, H., Y. Cao, L. Ma, Y. Wei, and H. Li. 2021. “Possible Mechanisms of Tau Spread and Toxicity in Alzheimer’s Disease.” Frontiers in Cell and Developmental Biology 9: 707268. https://doi.org/10.3389/fcell.2021.707268.
- Zhang, X., X. Chen, and X. Zhang. 2018. “The Impact of Exposure to Air Pollution on Cognitive Performance.” Proceedings of the National Academy of Sciences 115 (37): 9193–9197. https://doi.org/10.1073/pnas.1809474115.