ABSTRACT
Introduction: Cardiometabolic diseases (CMD) are a group of interrelated disorders such as metabolic syndrome, type 2 diabetes mellitus and cardiovascular diseases (CVD). As the prevalence of these diseases increases globally, efficient new strategies are necessary to target CMD and modifiable risk factors. In the past decade, evidence has accumulated regarding the influence of gut microbiota (GM) on CMD, providing new targets for therapeutic interventions.
Areas covered: This narrative review discusses the pathophysiologic link between CMD, GM, and potential microbiota-based targets against atherosclerosis and modifiable risk factors for atherosclerosis. Low-grade inflammation can be induced through GM and its derived metabolites. CMD are influenced by GM and microbiota-derived metabolites such as short-chain fatty acids (SCFA), secondary bile acids, trimethylamine N-oxide (TMAO), and the composition of GM can modulate host metabolism. All of the above can lead to promising therapeutic targets.
Expert opinion: Most data are derived from animal models or human association studies; therefore, more translational and interventional research in humans is necessary to validate these promising findings. Reproduced findings such as aberrant microbiota patterns or circulating biomarkers could be targeted depending on individual metabolic profiles, moving toward personalized medicine in CMD.
1. Introduction
Cardiometabolic diseases (CMD) are conditions with shared risk factors and similar phenotypes in different organs which are influenced by a combination of environmental and genetic factors [Citation1]. These cardiometabolic conditions, including type 2 diabetes mellitus (T2DM), metabolic syndrome (MS), obesity, and cardiovascular diseases (CVD), represent a major global healthcare problem [Citation2,Citation3]. This is due to the increasing prevalence of these conditions as well as their high morbidity [Citation4]. Between 1980 and 2014 the global prevalence of T2DM among adults has almost doubled from 4.7% to 8.5%, which represents 422 million people worldwide [Citation5]. T2DM patients have an increased risk to develop CVD and the risk of diabetes increases with age, especially in adults above 65 years [Citation6]. In 2012, 2.2 million deaths occurred globally by complications of T2DM, mainly due to CVD [Citation5]. CVD are still the number one cause of death worldwide, with 17.6 million deaths in 2016 representing 48% of the overall global mortality, mainly due to atherosclerosis [Citation7]. Importantly, oxidative stress and systemic inflammation represent two pathophysiological factors involved in diabetes-induced complications [Citation6]. In recent years, gut microbiota (GM) have been associated with T2DM and CVD [Citation2,Citation8–Citation11].
GM consist of bacteria, fungi, viruses, and protozoa, which together make up for about 1014 or 100 trillion microbes, the majority of which are viruses and bacteria [Citation12]. Bacteria in the gut belong to five phyla: Firmicutes, Bacteroidetes, Proteobacteria, Actinobacteria, and Verrucomicrobia [Citation13]. GM have many different functions in the human body, including the breakdown or fermentation of macronutrients which provide nutrients for human cells as well as for other intestinal bacteria [Citation14]. Bacterial breakdown of food occurs mainly through saccharolysis or proteolysis. Saccharolytic fermentation of carbohydrates leads to the production of short-chain fatty acids (SCFA), predominantly butyrate, acetate, and propionate; three bacterial metabolites whose role in host health has been extensively studied [Citation15,Citation16]. Proteolysis, the fermentation of proteins, minimally contributes to SCFA production and in contrast to the mainly positive associations of carbohydrate derived SCFA on host health, protein derived metabolites such as branched-chain amino acids (BCAA) have been identified as having detrimental effects on host metabolism [Citation17,Citation18]. Microbiota-derived molecules such as lipopolysaccharides (LPS) have been implicated to negatively influence metabolic processes in the intestine and in distant organs [Citation2,Citation8]. This narrative review will focus on the pathophysiologic link between CMD, GM, and GM-derived metabolites. In addition, we will discuss potential therapeutic bacterial targets against atherosclerosis.
2. Gut microbiota and metabolic disorders
2.1. Dysbiosis, low-grade inflammation, and metabolic disorders
Dietary patterns, lifestyle, and disease influence the composition of GM [Citation19,Citation20]. An altered GM composition associated with negative effects is called dysbiosis. Long-term effects of a diet rich in carbohydrates or protein and animal fat shape GM distinctively [Citation21]. Short-term dietary changes only have transient impact on GM and GM usually remain stable during most of adulthood [Citation22,Citation23]. Current evidence for the influence of GM on host metabolism is mainly derived from animal studies. Germ-free mice, which lack GM, have reduced body fat content and accumulation of macrophages in white adipose tissue compared to conventionally raised mice [Citation24]. These parameters coincide with better regulation of glucose and insulin levels compared to conventionally raised mice. Subsequent introduction of Escherichia coli to germ-free mice resulted in impaired glycemic regulation and macrophage accumulation with M1 polarization. Although this implicates a role for the GM in regulation of glucose and insulin metabolism, it is critical to mention that results from mice, in particular simplified models in which GM comprise only one (pathogenic) species, are difficult to translate to humans [Citation24]. However, a Western diet has been shown to increase intestinal E. coli abundances in mice [Citation25]. A Western diet is characterized by a high-caloric intake of fats, readily available carbohydrates and refined sugars, which contributes to the development of metabolic alterations such as insulin resistance, obesity, and T2DM [Citation26,Citation27]. Obesity has been associated with reduced GM diversity, altered relative abundances of the major phyla Firmicutes and Bacteroidetes as well as low-grade inflammation in mice and humans [Citation28–Citation30]. Moreover, individuals with insulin resistance display an altered microbiota composition and an altered microbiota-derived metabolite profile with higher levels of circulating BCAA [Citation31,Citation32]. High levels of BCAA, mainly derived from mammalian muscle protein and associated with specific GM compositions, have recently emerged as contributors to the development of insulin resistance and diabetes [Citation31]. Interestingly, healthy diet interventions (i.e. moderately restrictive diet enriched in fiber) improve GM diversity and metabolic alterations [Citation30]. Furthermore, a successful exercise intervention in pre-diabetic patients is correlated with particular changes in GM function, including a higher capacity for SCFA synthesis and BCAA catabolism [Citation33]. An increase in BCAA catabolism could therefore be beneficial for host metabolism. This might sound paradoxical, as increased BCAA have been associated with insulin resistance [Citation34]. However, elevated BCAA levels leading to insulin resistance have been associated with a deficiency in catabolic capacity of white adipose tissue, liver tissue, and skeletal muscles [Citation31]. This suggests that lifestyle and diet can induce dysbiosis which has been suggested to contribute to the development of obesity and T2D (). In addition to this, dietary ingested lard results in reduced insulin sensitivity, increased Toll-like receptor (TLR) activation, and white adipose tissue inflammation in mice, compared to mice on a diet rich in fish oil. The inflammatory phenotype of lard fed mice has also been linked to differences in GM composition [Citation35].
Figure 1. Suggested influence of gut microbiota (GM) proteolysis and saccharolysis on host insulin resistance and inflammation. The breakdown of proteins (proteolysis) by GM is associated with lower short-chain fatty acid (SCFA) levels as well as increased detrimental metabolites such as imidazole propionate (ImP) from the breakdown of histidine and BCAA from mammalian muscle protein, which is associated with increased intestinal permeability. This results in higher insulin resistance, worsened glucose metabolism as well as increased inflammatory markers. GM fermentation of complex carbohydrates (saccharolysis) such as fibers is associated with increased production of beneficial compounds. Examples of these beneficial compounds are SCFA like propionate and butyrate, secondary bile acids, and lower levels of detrimental metabolites such as BCAA, leading to improved glucose control, lower insulin resistance, and a reduction in inflammation.
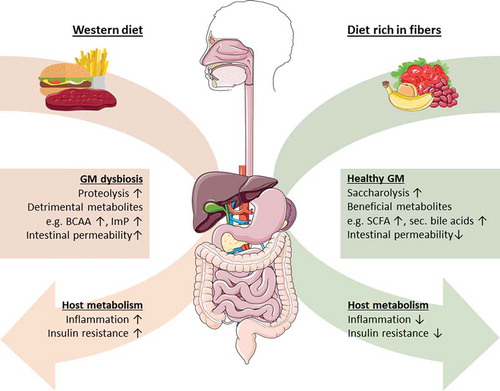
Low-grade inflammation is a chronic sterile inflammation with several mild properties of an acute inflammation such as elevated inflammatory markers but without one clear cause. In contrast, an acute inflammation is generally a physiologic response to an infection or tissue injury. Recently, postprandial inflammation was proposed to have a beneficial physiologic function in food processing and absorption, given that one is not continuously in the postprandial inflammatory state [Citation9,Citation36]. In humans with and without T2DM, inflammatory markers are known to positively correlate with visceral adiposity, the amount of which correlates proportionally with the risk of CVD [Citation37].
Several studies have associated aspects of GM such as a low diversity and the abundance of specific species with markers of systemic inflammation. These markers include increased circulating immune cells and inflammatory molecules such as C-reactive protein (CRP) and cytokines, as well as inflammation within white adipose tissue in animal and human studies [Citation24,Citation30,Citation38]. Indeed, several human studies found an association between a higher white blood cell count and either a low bacterial gene diversity or altered proportions of specific bacteria in the intestine (i.e. dysbiosis) [Citation29,Citation30,Citation39–Citation41]. Furthermore, increased high sensitive-CRP (hs-CRP) has been associated with both, lower intestinal bacterial gene count and the abundance of specific bacteria genera, as Ruminococcus, Faecalibacterium, Lactobacillus, Bifidobacterium, and Streptococcus were inversely associated with hs-CRP [Citation38]. Whereas Clostridium abundance correlates with increased interleukin 5 (IL-5) and IL-17 in humans, Ruminococcaceae and Faecalibacteria abundance, specifically F. prausnitzii, were inversely correlated to IL-6 and IL-17, respectively [Citation38]. Suggesting that some bacteria might stimulate the production of pro-inflammatory cytokines while others display anti-inflammatory properties ().
Table 1. Cardiometabolic effects of specific bacteria.
These data suggest that GM can profoundly influence host metabolism, which results in pro-inflammatory and anti-inflammatory pathway activations depending on the GM composition.
It has been hypothesized that microbiota-produced metabolites can trigger host inflammatory reactions. Two mechanisms by which these molecules enter the body have been described [Citation42]. LPS are major components of the cell membrane of Gram-negative bacteria and are suggested to enter the circulation after translocation from the gut into the bloodstream, triggering an inflammatory response [Citation42]. Although evidence for this concept in humans is very scarce, it has been shown in mice that obesity is associated with impaired intestinal barrier function through loss of intestinal tight junctions [Citation42]. Furthermore, it has been hypothesized that bacteria are being transported from the intestinal lumen by chylomicrons and lipoproteins after dietary lipid ingestion, leading to increased circulating LPS levels [Citation42–Citation45]. A high-fat diet increases circulating LPS in mice, which has been shown to trigger adipose tissue inflammation and liver insulin resistance, resulting in conditions similar to those observed in obesity and T2DM [Citation42]. Adults with T2DM were shown to have a similar metabolic phenotype with increased intestinal permeability and elevated circulating inflammatory markers such as CRP, IL-6, and tumor necrosis factor alpha (TNF-α) [Citation46]. Nevertheless, conflicting results about increased intestinal permeability in human obesity can be found. Indeed, Genser et al. [Citation47] showed increased ex vivo permeability of jejunal tissue from humans with obesity and T2DM only after a lipid challenge and not in fasting conditions. However, circulating LPS has been shown to cause inflammation through the activation of the innate immune system via pattern recognition receptors (PRRs) such as TLR4 and nucleotide-binding oligomerization domain (NOD) like receptors to recognize and react to pathogen-associated molecular patterns (PAMPs) such as LPS [Citation48,Citation49]. Interestingly, the lipid A domain of LPS is the most immunogenic part of LPS and varieties in lipid A composition are found between bacteria species; hence, immunogenicity also varies between bacteria species [Citation50]. The number of acyl chains in the lipid A domain correlates with the host inflammatory response, in addition to this, phosphate groups bound to LPS are also immunogenic. For example, LPS from E. coli usually have more acyl chains than LPS from other bacteria and LPS from E. coli are therefore known to have potent pro-inflammatory properties [Citation50]. In line with this, colonization of germ-free mice with the Gram-negative bacteria E. coli strain W3110 but not with the hypoimmunogenic strain MLK1067, which also contains less acyl chains than W3110, resulted in increased circulating LPS levels [Citation24,Citation51]. Concordant with this, levels of pro-inflammatory TNF-α and the ratio of M1/M2 macrophages, an inflammatory marker often increased in obesity and T2DM, were increased within white adipose tissue. Anti-inflammatory markers, however, were also upregulated, possibly as a reaction against the described pro-inflammatory processes, underlining the complexity of white adipose tissue inflammation [Citation24].
In summary, it has been shown that host metabolism can be altered by modulations of GM. Diet, in particular a Western diet, has been implicated to alter intestinal barrier function, at least in mice, which allows translocation of the immunogenic bacterial compound LPS into the circulation. This results in adipose tissue and systemic inflammation in several animal models.
3. Obesity and type 2 diabetes mellitus
The development of T2DM is complex in particular due to its multifactorial pathophysiology. Insulin resistance, mainly driven by obesity, is an essential aspect of T2DM [Citation52]. Obesity results from a positive energy balance and leads to immune cell infiltration within adipose tissue [Citation53], thereby contributing to the low-grade inflammatory tone. Inflammation, hyperglycemia, hyperinsulinemia, lipotoxicity, and genetic predisposition are heavily studied drivers of insulin resistance in obesity [Citation52]. As mentioned in the previous paragraph, increased circulating LPS levels have been associated with inflammation and insulin resistance in mice.
Obesity and T2DM-related inflammation are mediated through different molecular pathways and can in part be explained by GM-derived metabolites such as LPS. This molecule is a well-known activator of the innate immune system through TLR4 and subsequent NF-κB activation, which inhibits the phosphorylation of insulin receptor substrates (IRS) through c-Jun N-terminal protein Kinase (JNK) activation, hence inducing insulin resistance [Citation54]. Pro-inflammatory pathways in obese individuals can also be mediated through adipocytes which eventually activate receptors controlled by secondary bile acids through the following mechanism. In obese individuals, adipocytes secrete monocyte chemoattractant protein 1 (MCP-1) which attracts macrophages and drives insulin resistance. Adipocytes and attracted macrophages secrete cytokines such as IL-1β, IL-6, and TNF-α which also drive insulin resistance through several mechanisms including activation of serine/threonine (Ser/Thr) kinases with subsequent JNK and IRS-2 signaling as well as activation of protein kinase C (PKC) [Citation52]. Interestingly, PKC activation also phosphorylates the nuclear receptor farnesoid X receptor (FXR) and small heterodimer partner (SHP), which control bile acid synthesis, lipid metabolism, and inflammation, and activation of these receptors have been shown to reverse insulin resistance in obese rats [Citation52,Citation55,Citation56]. Natural ligands of FXR include primary and microbially produced secondary bile acids [Citation57], demonstrating that GM-derived metabolites can also antagonize obesity-induced inflammation.
Several studies have linked GM to metabolic disorders. And as previously discussed, bacteria-derived metabolites can also influence host metabolism, some have beneficial effects while others are involved in disease development or progression. For example, SCFA produced by GM upon fiber digestion can improve glucose regulation, insulin sensitivity, and reduce systemic and adipose tissue inflammation [Citation16]. In contrast, elevated levels of BCAA are associated with inflammation and insulin resistance [Citation31]. Imidazole propionate, a GM-produced metabolite has been shown to impair hepatic insulin signaling [Citation58], and TMAO, another GM-produced metabolite has been shown to be atherogenic [Citation8,Citation58]. LPS, which is directly derived from GM can translocate from the gut to the systemic circulation where it induces inflammation [Citation36]. This suggests that GM-derived metabolites can have hormone-like properties as they can influence host metabolism in various ways [Citation8]. Furthermore, some characteristics of the GM have been associated with altered host metabolism. Low bacterial diversity is generally associated with insulin resistance, dyslipidemia, and adiposity [Citation29,Citation30]. Moreover, compared with obese individuals with high bacterial diversity, obese individuals with low bacterial diversity were shown to be at higher risk of gaining weight during a five-year follow-up [Citation29]. Furthermore, obese subjects with low bacterial diversity lose less weight upon a moderate restrictive diet than subjects with a high bacterial diversity [Citation30]. GM might therefore have a predictive value to gauge the susceptibility of individuals to develop CMD. GM of obese subjects have been suggested to extract more energy from the diet due to alterations in relative abundance of Firmicutes and Bacteroidetes compared to individuals with high bacterial richness [Citation59]. Subjects with T2DM in a Chinese cohort were shown to have a distinct GM profile compared to healthy volunteers. Especially butyrate-producing bacteria such as Clostridiales sp. SS3/4, Eubacterium rectale, Faecalibacterium prausnitzii, Roseburia intestinalis, and Roseburia inulinivorans were less abundant in subjects with T2DM with corresponding overgrowth of a diverse group of opportunistic bacteria [Citation10].
3.1. Microbiota-derived metabolites linked to CMD
In the gut, microbiota-derived metabolites are usually derived from either proteolysis or saccharolysis (). Examples of microbiome-derived modulators of host metabolism are SCFA such as butyrate, acetate, and propionate which together make up for >95% of intestinal SCFA [Citation16,Citation58,Citation60]. SCFA are produced in the entire intestinal tract, yet more abundantly in the colon than in the small intestine. SCFA are mostly the product of saccharolysis, driven by bacterial fermentation of complex dietary carbohydrates which are otherwise indigestible by the human digestive tract [Citation61]. Fecal concentrations of acetate are three times higher than propionate and butyrate, the two latter being present in the same concentrations [Citation16]. These three SCFA exert differential roles: while butyrate functions as the preferred energy source for colonic epithelial cells [Citation62], acetate may be used as a fatty acid precursor or for cholesterologenesis and finally propionate acts as a main substrate for gluconeogenesis [Citation63,Citation64]. Butyrate and acetate are also important for epithelial barrier function, since, for example, butyrate has been shown to increase mucus production and tight junction protein expression [Citation65,Citation66]. SCFA can modulate glucose metabolism by regulating insulin sensitivity and by modifying inflammatory responses [Citation16,Citation61]. SCFA have anti-inflammatory properties by inhibiting the NF-κB pathway [Citation67,Citation68]. In mice on a high-fat diet, supplementation of acetate, butyrate, and propionate to the diet reduced body weight and improved insulin sensitivity through the peroxisome proliferator-activated receptor-ɣ [Citation69]. In subjects with the metabolic syndrome, fecal microbiota transplantation (FMT) from lean donors improved insulin sensitivity concomitant with increased levels of intestinal butyrate-producing bacteria [Citation70]. Nevertheless, it remains uncertain whether changes in butyrate caused this improvement in insulin sensitivity, as the study was not designed to evaluate a causal link [Citation70]. Noteworthy, four weeks of oral butyrate supplementation to metabolic syndrome subjects showed no effect on insulin sensitivity [Citation71]. The absence of effect could be due to the oral ingestion of butyrate.
The recently discovered GM-derived metabolite imidazole propionate can also influence host metabolism in mice. This metabolite is produced from dietary histidine upon intestinal proteolysis by GM [Citation58]. Imidazole propionate is elevated in serum and feces of adults with T2DM and when injected to mice, fasting and postprandial glucose levels are elevated and insulin signaling is impaired through IRS degradation [Citation58]. It is likely that many yet to be identified microbiota-derived metabolites influence host metabolism. As these intestinal metabolites are presumably present in big numbers, research methods that allow comprehension of big numbers and complex metabolite patterns such as untargeted metabolomics analysis are necessary to find new drug targets to customize the treatment of CMD [Citation72]. To identify new metabolites that influence host metabolism, various body samples can be studied such as organ tissue, blood plasma or serum, feces, urine, saliva cerebrospinal fluid, and exhaled breath [Citation73]. Metabolites of these samples are then analyzed by nuclear magnetic resonance spectroscopy and mass spectrometry in tandem with methods such as capillary electrophoresis, liquid and gas chromatography, the latter being considered the gold standard for metabolomics analysis until so far [Citation74]. Combing data from different sample types and different CMD ‘states’ (e.g. prediabetic, T2DM, and T2DM with atherosclerosis) could help identify new metabolites that drive CMD and lead to the development of drugs that can target these newly discovered pathways.
3.2. Bile acid metabolism
The role of bile acid metabolism in CMD has been of interest in several studies and GM have an essential role in this regard, as bile acid metabolism is in part dependent on GM [Citation57]. Primary bile acids are produced in the liver from cholesterol and are mostly conjugated to glycine in the human liver to reduce toxicity and enhance solubility into bile [Citation75]. Bile is temporarily stored in the gallbladder until food intake triggers bile release into the duodenum [Citation76]. In the small intestine, 95% of primary bile acids are reabsorbed by enterocytes, mostly in the ileum [Citation75], conversion of the remaining primary bile acids to secondary bile acids occurs in the colon by GM [Citation77]. This so-called enterohepatic circulation is controlled by a negative feedback loop in which bile acids regulate their own production. Importantly, bile acid profiles differ strongly between species due to various reasons. For example, the GM composition in rodent colonies is usually harmonized by coprophagia which is not the case in humans [Citation78]. The primary endpoint of bile acid synthesis and the most abundant bile acids in humans are chenodeoxycholic acid (CDCA) and cholic acid [Citation79]. In contrast, different hydroxylation reactions result in cholic acid and muricholic acid, being the most abundant bile acids in rodents [Citation80]. Before secretion into the canalicular lumen in the liver, conjugation occurs preferably to taurine in mammals, but as humans usually ingest small amounts of taurine, most bile acids are conjugated to glycine in humans [Citation79].
Bile acids also act as key regulatory molecules that influence other metabolic pathways such as lipid, energy, and glucose metabolism [Citation75]. An important receptor involved in the regulation of bile acid metabolism is FXR, which regulates the downstream protein fibroblast growth factor 19 (FGF19). Another important receptor in bile acid metabolism is the Takeda G protein–coupled receptor (TGR5) [Citation81]. FXR is expressed in cells of the intestines, liver, and kidneys, and TGR5 is mainly found in immune cells and intestines [Citation82]. TGR5 has been shown to promote the secretion of the incretin glucagon-like peptide-1 (GLP-1) in murine enteroendocrine cells [Citation83]. GLP-1 has several beneficial properties such as glucose-dependent insulin secretion, attenuation of elevated hepatic glucose production, reducing postprandial lipid levels, enhancing satiety, and reducing systemic inflammation [Citation84]. These factors likely contribute to cardioprotection, which has been confirmed by the use of synthetic GLP-1 receptor agonists, leading to reduced inflammatory markers and atherosclerotic lesions in murine models [Citation84]. TGR5 may also promote mitochondrial uncoupling in brown adipose tissue (BAT) in vitro and increased the activity of BAT as well as whole body energy expenditure in vivo [Citation85]. Activation of the nuclear receptor FXR has been associated with beneficial effects on metabolism such as reduction of body weight and inflammatory markers in mice [Citation86]. CDCA functions as a FXR agonist and administration of CDCA in a rat T2DM model reduced insulin and triglyceride levels but did not reduce body weight while FXR gene expression in the liver was increased [Citation87]. However, the other major bile acid signaling receptor, TGR5, could have also mediated this effect but this was not tested in this study [Citation85,Citation87]. In a mouse model, bacterial bile acid modification in the gut modulated lipid metabolism and contributed to weight gain in the host [Citation88]. Conversion of primary bile acids to secondary bile acids comprises a multitude of biotransformations in which different gut bacteria are necessary for completion; the composition of GM therefore importantly influences the production of secondary bile acids [Citation89]. An essential step in this conversion is completed by the enzyme bile salt hydrolase (BSH), capable of cleaving amide bond C24 [Citation89]. Interestingly, human FMT studies, which modify GM composition, have shown that secondary bile acid profiles can indeed be changed for a short period [Citation90,Citation91]. Dysbiosis in active inflammatory bowel patients has been shown to increase levels of sulfated bile acids, which have lower anti-inflammatory effects in vitro [Citation92]. Dysbiosis in cardiometabolic patients could therefore also lead to reduced anti-inflammatory effects of bile acids. These studies show that features of metabolic disorders can be improved by bile acid signaling in animals and humans through various receptors. These receptors, with bile acids as their natural ligands, can be modulated by GM; however, beneficial findings differ between animal and human studies, possibly due to differences in bile acid profiles [Citation79].
4. Gut microbiota and atherosclerosis
Obesity, chronic low-grade inflammation, and T2DM all increase the risk of atherosclerosis () [Citation2,Citation93]. As metabolic diseases are associated with GM dysbiosis, we will here review the influence of GM on atherosclerosis, since these conditions can have lethal complications such as arterial thrombosis in CMD patients [Citation5,Citation94]. Atherosclerosis is considered a chronic inflammatory disease which is initiated by endothelial cell injury and subsequent plaque formation [Citation95]. GM and microbiota-derived metabolites have been shown to be associated with atherosclerosis [Citation96,Citation97]. Increased levels of plasma endotoxin have indeed been linked to CVD development [Citation98], and consecutive injections of endotoxin seem to accelerate cholesterol accumulation and atherosclerosis in animal models of CVD [Citation99,Citation100]. It has been suggested that activation of macrophages in humans can be linked to Porphyromonas gingivalis in 79% of carotid atherosclerotic plaques [Citation101,Citation102]. Unfortunately, antibiotic treatment against this specific bacterial strain for CVD has not been able to reduce cardiovascular events in a large cohort of patients at risk of CVD [Citation103]. Suggesting, that either long-term modulation of GM is necessary or that several other pathophysiological pathways are also involved. However, other more indirect markers of aberrant GM function have come to the stage, including TMAO.
Figure 2. Simplified overview of gut microbiota (GM) contribution to the development of cardiometabolic diseases based on available animal and human studies. High-fat diet or diets rich in meat and eggs are associated with dysbiosis and have been shown to disrupt the intestinal barrier, this way, bacteria-derived endotoxins such as lipopolysaccharides (LPS), trimethylamine N-oxide (TMAO), and imidazole propionate (ImP) can enter the portal and systemic circulation. Circulating LPS induces systemic inflammation and inflammation of adipose tissue, which is associated with insulin resistance in the liver and muscle tissue, facilitating the onset of type 2 diabetes mellitus (T2DM). T2DM subjects have altered GM compositions, also called dysbiosis. T2DM patients with dysbiosis have an increased intestinal permeability, resulting again in endotoxemia. Inflammation, T2DM, and GM-derived metabolites have been associated with the development of atherosclerosis and can also influence thrombus growth.
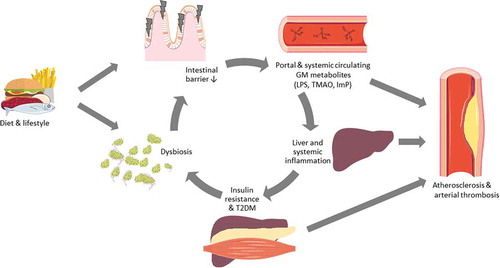
4.1. TMAO and atherosclerosis
TMAO is a bacterial metabolite derived from dietary ingested carnitine and phosphatidylcholine [Citation104]. Phosphatidylcholine is mainly found in meat, fish, and eggs but can also be produced by the liver [Citation105]. However, dietary intake has been shown to be necessary to produce elevated levels of TMAO [Citation97]. Phosphatidylcholine is hydrolyzed by microbiota in the intestine by trimethylamine (TMA) lyase to the gas TMA [Citation104]. The influence of GM on TMAO levels became clear after antibiotic treatment, which completely suppressed circulating TMAO concentrations after a L-carnitine challenge in humans [Citation96]. After TMA production by GM, this metabolite then enters the portal circulation and flavin monooxygenases (FMOs), mainly FMO3, oxidize TMA in the liver to the atherogenic TMAO [Citation106,Citation107]. After oxidation by FMOs, TMAO can enter the circulation and will eventually be excreted by the kidneys [Citation108].
TMAO and a TMAO precursor, y-butyrobetaine, have been associated with atherosclerosis [Citation97,Citation109]. In addition to this, TMAO has been associated with major adverse cardiovascular events. In two large cohort studies of patients with coronary angiography-proven plaques, elevated TMAO levels were associated with major adverse cardiovascular events during follow-up [Citation110,Citation111]. Although this result has not been universally replicated [Citation112], similar data on the association between TMAO and cardiovascular events were found in different studies and meta-analyses. Here, a TMAO threshold of approximately 6 µM or more was found to be a good predictor for adverse cardiac events [Citation113–Citation115]. In mice, FMT from an atherogenic strain which also produces high levels of TMAO to a low atherogenic and low TMAO producing strain transferred the increased susceptibility of atherosclerosis [Citation116]. In addition to this, TMAO levels were elevated after FMT in the low-risk mice [Citation116]. Furthermore, increased levels of FMO3, the most important enzyme in TMAO production, are associated with increased plasma TMAO levels in mice [Citation107]. Accordingly, silencing of FMO3 decreased plasma levels of TMAO [Citation107], revealing FMO3 as a potential target to regulate TMAO levels. In accordance, other murine studies have also suggested a link between TMAO levels and bile acid metabolism as elevated TMAO levels have been shown to increase the susceptibility for atherosclerosis, possibly through modulation of lipid metabolism by bile acids in female mice but not in their male counterparts [Citation107]. Possibly due to hormonal differences. Indeed, it has been proposed that TMAO metabolism is under hormonal control, as FMO3 capacity was reduced based on the TMAO and total TMA ratio during menstruation of a case series with two women [Citation117]. This could explain the gender differences observed in FMO3 expression [Citation107]. However, effects of TMAO and nutrition on atherosclerosis vary across different experimental and nutritional conditions. In a study using Apoprotein E (ApoE) deficient mice fed a Western diet supplemented with choline, TMAO levels were increased in conventionally raised mice compared to germ-free mice. Nevertheless, this was not associated with increased plasma cholesterol levels or aortic lesions size [Citation118]. In addition to this, ApoE-deficient mice, which were also incapable to respond to LPS, had unchanged aortic cholesteryl ester levels, compared to ApoE only deficient mice [Citation119]. As aortic cholesteryl levels are a measure for atherogenesis, this suggests that atherosclerosis can also develop without LPS induced inflammation. However, other GM-derived metabolites such as TMAO were not assessed, leaving the question unanswered as to what role other metabolites might play in the development of atherosclerosis. Furthermore, conventionally raised or germ-free mice deficient for the low-density lipoprotein (LDL) receptor fed a high-fat Western diet, displayed similar aortic lesions in the common carotid artery [Citation120]. Nevertheless, this result is challenged by diverging data obtained in mice deficient for ApoE. Indeed, germ-free ApoE-deficient mice had higher plasma cholesterol levels and altered bile acid signaling, yet reduced atherosclerotic lesions compared to conventionally raised ApoE-deficient mice [Citation121], suggesting the importance of GM in this pathophysiological pathway. In line with this, germ-free ApoE-deficient mice on a low cholesterol diet have more atherosclerotic lesions than conventionally raised mice on the same diet, these differences were not visible when both strains were fed a high-cholesterol diet [Citation122].
Increased TMAO levels seem to influence several aspects of lipid metabolism and lead to increased platelet hyperreactivity, another mechanism involved in complications of atherosclerosis. This phenotype can also be transferred by FMT [Citation107,Citation123]. Increased TMAO levels have been shown to decrease reverse cholesterol transport [Citation96], increase macrophage cholesterol accumulation [Citation97], vascular dysfunction, and endogenous inflammation in mice [Citation124]. A study in healthy controls did not show an effect of increased serum TMAO levels on cardiovascular mortality or hospitalization [Citation125]; however, meta-analyses which included recent negative studies still confirmed the positive association between TMAO, CVD events, and mortality [Citation113–Citation115]. Likewise, FMT was not able to alter TMAO fluxes and vascular inflammation despite an effect on GM composition [Citation126]. These processes can lead to atherosclerosis and as previously discussed, are influenced by the GM, which underlines the critical association between TMAO, GM, and CVD. However, it could also be that TMAO is a surrogate marker for atherosclerosis instead of being the driver of atherosclerosis. Further studies are needed to elucidate the complete mechanistic effect of TMAO on atherosclerosis.
4.2. Gut microbiota strains and atherosclerosis
Research on the composition of GM in patients with atherosclerosis has brought new insights into a potential microbial signature of CVD. Patients with coronary artery disease displayed a different microbiota profile than healthy controls, mainly characterized by an increase in the Lactobacillales order and a decrease at the Bacteroidetes phylum level such as Bacteroides and Prevotella at the genus level [Citation127]. Jie et al. showed that individuals with atherosclerosis displayed decreased numbers of butyrate-producing bacteria such as Roseburia intestinalis and Faecalibacterium prausnitzii and increased Enterobacteriaceae such as E. coli, Klebsiella spp., and Enterobacter aerogenes compared to healthy controls () [Citation128].
Concerning the effect of SCFA in CVD, in ApoE-deficient mice on a chow diet, supplementation of 1% butyrate to their diet resulted in a 50% reduction of atherosclerosis [Citation129]. In agreement, the butyrate-producing bacteria Roseburia intestinalis displayed a negative relationship with atherosclerosis and systemic inflammation in ApoE-deficient mice [Citation130]. In a meta-analysis including solely human studies, no association between dietary choline, betaine and CVD or mortality was found [Citation131]. An effect of bile acid metabolism on atherosclerosis possibly through GLP-1 has been established. The bile acid receptor TGR5 can promote the secretion of GLP-1, which has been shown to reduce monocyte adhesion and atherosclerotic lesions [Citation83,Citation132]. These data show that GM produce metabolites that affect the risk of CVD through different pathways such as by modulation of bile acids, SCFA, TMAO, lipid and glucose metabolism, and GM composition.
5. Potential therapeutic targets
5.1. Bacterial metabolites
Several microbiota-derived metabolites have been reviewed in this article that may increase or reduce the risk of CMD and atherosclerosis in particular. Targeting these metabolites could potentially modulate the risk of atherosclerosis, as is aimed in several studies. To reduce the synthesis of TMAO, the production of several precursors could be influenced. For example, the enzyme TMA-lyase (CutC gene), which hydrolyzes choline to TMA, is a promising target to prevent the production of TMAO [Citation133]. Bacteria that entail Cut gene clusters for this enzyme are Actinobacteria, Proteobacteria, and Firmicutes [Citation133]. To reduce TMAO synthesis, the production pathway of TMA was shown to be impaired by the usage of the choline analog 3,3-dimethyl-1-butanol (DMB) in ApoE-deficient mice on a high-choline or L-carnitine diet [Citation134]. In the same study, the use of DMB successfully inhibited TMA-lyase activity in a non-lethal manner, resulting in reduced TMAO levels but more importantly lower endogenous macrophage foam cell recruitment and decreased development of atherosclerosis [Citation134]. Phospholipase D is another enzyme which is used and even preferred by many bacteria to hydrolyze phosphatidylcholine to choline [Citation135]. As phosphatidylcholine is abundant in various foods, this enzyme has recently been shown to importantly influence TMA production and thus affect TMAO levels [Citation135]. Future interventional studies could target this enzyme to inhibit TMAO production, which thus far, has not been studied to our knowledge. As dietary compounds are the precursors of TMAO, research on specific diets has also been conducted. A pilot study revealed that an intermittent fasting regimen or a hypocaloric diet regimen decreased TMAO levels [Citation136,Citation137]; however, beneficial clinical outcome measures were not assessed. As arterial thrombosis ultimately occurs by coagulating blood, the effect of GM on coagulation has also been studied. In the study of Jäckel et al. [Citation138], germ-free mice displayed less thrombus growth than conventionally raised mice in a carotid artery thrombosis model. Additionally, germ-free mice had lower circulating von Willebrand factor levels and lower liver gene expression of von Willebrand factor. Since TLR2 deficient mice displayed a similar phenotype with reduced thrombus growth, the authors demonstrated that GM are involved in thrombus growth through TLR2 activation [Citation138]. Concordantly, the biosynthesis pathway of peptidoglycans, which are TLR2 agonists, was upregulated in the metagenome of patients with symptomatic carotid atherosclerosis [Citation139,Citation140]. This concept has already been translated to an intervention study, as oral aspirin treatment improved platelet hyperresponsiveness but also increased circulating TMAO levels [Citation141].
As bile acids are also known to regulate lipid, energy and glucose metabolism, modulation of bile acids has also been studied to influence CVD [Citation75]. The secondary bile acid ursodeoxycholic acid (UDCA) has recently been investigated in obese mice [Citation142]. Administration of UDCA was shown to improve metabolic markers such as macrophage-induced inflammation, free fatty acids, triglycerides, blood glucose, liver lipid content, and mitochondrial function in mice [Citation142,Citation143]. As UDCA is already approved to treat primary biliary cholangitis by the U.S. Food and Drug Administration for humans, usage of this drug is an attractive option as experience in humans with this compound already exists [Citation142]. Another attractive target is FXR, which is involved in the regulation of glucose and lipid metabolism [Citation87]. Administration of the selective FXR receptor agonist Fexeramine reduced systemic inflammation, hepatic glucose production, and diet-induced weight gain while promoting browning of white adipose tissue and thermogenesis in mice [Citation86]. Other studies demonstrated the protective effect of FXR against atherosclerosis in different models [Citation144,Citation145]. Combined activation of the two bile acid receptors FXR and TGR5 by INT-767 reversed obesity and atherosclerosis in TGR5, FXR, ApoE, and SHP-deficient mice [Citation146]. Conversely, the inhibition of both receptors in a LDL, TGR5, and FXR knockout mouse model induced vascular inflammation and atherosclerosis through NFκB-signaling [Citation82], demonstrating the protective role of bile acid metabolism in the development of atherosclerosis in mice.
Various other GM-based metabolites have been shown to influence (the risk of) CVD and could therefore be targeted to prevent and reduce CVD. The two metabolites tryptamine and indole-3- acetate are dependent on GM for their production and are suppressed by a high-fat diet in mice [Citation147]. These two metabolites had anti-inflammatory effects on macrophages and reduced fatty acid production in the same model [Citation147]. BCAA represent another set of compounds associated with CVD in humans [Citation148]. Two bacterial species, Prevotella copri and Bacteroides vulgatus were identified as main driving species linking insulin resistance and increased circulating BCAA in humans [Citation34]. This study contributes to the notion that elimination or depletion of some bacteria could have beneficial effects on host cardiometabolic status. Rodent models with elevated expression of BSH, a bacterial enzyme involved in bile acid fermentation, displayed reduced weight gain, liver triglycerides, and plasma cholesterol in mice, suggesting that targeting bile acid composition through the GM could improve some CMD features [Citation88]. The aforementioned benefits could therefore be yielded by bacteria with high BSH activity.
Since SCFA have important beneficial roles in host metabolism in mouse models [Citation16,Citation61], several drugs mimicking SCFA have been tested. Sodium butyrate is able to reduce obesity-induced inflammation in mice on a high-fat diet [Citation149], as did tributyrin in mice, which is a butyrate analog consisting of a triglyceride with three butyrate moieties [Citation150]. More interestingly, supplementation with the latter compound also inhibited macrophage accumulation in atherosclerotic plaque, lipid deposition, and atherosclerosis in mice [Citation130]. Research on gene expression is indicating more functions of other secondary metabolites of the microbiota, as gene clusters show antibiotic properties or protease inhibitory activity against proteases in human cells [Citation151,Citation152], revealing new targets to influence host metabolism.
Several metabolites and receptors provide leads for promising targets to either treat risk factors of atherosclerosis or directly intervene against an increased coagulation tendency and atherosclerosis development. Research in more clinically relevant models is necessary to validate the recent results and allow translation to humans.
5.2. Administration of single strains and microbiota replacement
To directly influence GM, the most obvious measure is to supplement specific bacteria directly into the gastrointestinal tract. The effect of single bacteria or whole microbiota administration on atherosclerosis has been evaluated in different models. In vitro, Bifidobacteria have been shown to remove cholesterol from the surrounding environment in the presence of bile acids [Citation153]. The bacterium Lactobacillus plantarum which is found in fermented vegetable foods and milk products reduced circulating cholesterol and triglycerides in hypercholesterolemic mice [Citation154]. L. plantarum also decreased TMAO levels in mice and could inhibit TMAO-induced atherosclerosis [Citation155]. Similar results were found upon administration of L. rhamnosis to ApoE-deficient mice on a high-fat diet. Indeed, L. rhamnosis attenuated oxidative stress, chronic inflammation, and the development of atherosclerosis [Citation156]. Daily ingestion of L. plantarum reduced LDL and triglycerides in human subjects with normal or mildly elevated cholesterol levels. Nevertheless, while this human study partially translated findings observed in mice, clinically relevant outcomes such as CVD events were not analyzed [Citation157]. Since Akkermansia muciniphila is decreased in obese T2DM mice, this strain represents a potential probiotic target to treat CMD [Citation158]. Feeding of A. muciniphila to mice, reversed atherosclerotic risk factors such as adipose tissue inflammation, fat-mass, insulin resistance, metabolic endotoxemia and even athersclerotic lesions in obese mice with T2DM and ApoE deficient mice, respectively [Citation158,Citation159]. Interestingly, the pasteurization of A. muciniphila even enhanced the beneficial effect on most atherosclerotic risk factors [Citation158,Citation160]. Although administration of A. muciniphila is effective in mice and was also shown to be safe in humans [Citation160], unfortunately, no clinically meaningful effect on the above-mentioned metabolic alterations was observed in humans [Citation161]. It probably suggests that A. muciniphila is not causal in CMD, but rather a surrogate marker which serves as a thermometer of intestinal health.
Effects of FMT on atherosclerosis have been limited so far. However, CMD risk factors can be targeted using this innovative treatment. FMT from lean donors to metabolic syndrome patients improved insulin resistance concomitantly with increasing the potentially beneficial butyrate-producing GM [Citation70,Citation129]. Vegetarians and vegans have lower endogenous TMAO levels than individuals following an omnivorous diet [Citation96], due to their low carnitine intake or beneficial properties of their GM. However, FMT from lean-vegan donors to patients with metabolic syndrome did not change TMAO levels or markers of vascular inflammation [Citation126]. In a recent study, mice with autoimmune myocarditis who received FMT from healthy mice had less myocardial injury due to reduced inflammatory infiltration [Citation162]. As FMT showed only limited results so far, this technique could be improved by enriching the transplant with bacteria strains that have beneficial metabolic effects to optimize the effect on host metabolism. Finally, since most studies are currently carried out in mice models, more translational research is necessary to confirm the beneficial effects found from the use of single-strain probiotics or FMT.
5.3. Antibiotics
Due to the important role of GM in CMD, as reviewed herein, targeting microbiota directly with antibiotics has been evaluated in recent studies. Treatment with a broad-spectrum antibiotic cocktail reduced glucose intolerance, inflammation, and hepatic steatosis in hamsters fed a high-fat diet [Citation163]. In a similar setup with obese mice, glucose tolerance, insulin levels, systemic inflammatory markers, as well as the number of macrophages in adipose tissue were reduced by the use of antibiotics [Citation164]. Administration of broad-spectrum antibiotics in healthy subjects diminished plasma TMAO levels until antibiotics withdrawal [Citation110]. The effect on potential clinical outcomes should still be evaluated. Nevertheless, a rodent study demonstrated endothelial dysfunction reversal after a poorly absorbed antibiotic cocktail supplementation in old mice [Citation165]. Another study showed that oral vancomycin administration in metabolic syndrome subjects influenced bile acid metabolism and decreased insulin sensitivity [Citation166]. In contrast, Reijnders et al. did not find any effect of vancomycin treatment on metabolism despite major changes in GM [Citation167]. This was most likely due to a difference in baseline GM diversity between both studies, as subjects with lower baseline diversity are probably more vulnerable to adverse antibiotic-induced metabolic effects. However, long-term use of antibiotics in women between 40 and 59 years and 60 years and older was associated with an increased risk of CVD [Citation168].
The use of antibiotics has not yet shown promising translational data, in fact, the use of antibiotics was associated with increased chances of adverse outcomes. Based on these data, the use of antibiotics alone will therefore not be a promising treatment in the future. However, in combination with other interventions which target GM such as pathway targeting, metabolite targeting, or live microbiota transplantation, beneficial results could be achieved. For example, a study evaluating if antibiotic treatment prior to FMT or probiotics supplementation improves GM engraftment and CMD outcomes would be an interesting future direction.
6. Conclusion
CMD share pathophysiological mechanisms, phenotypes and several risk factors, which are shown to be influenced by GM-derived metabolites. Low-grade inflammation can be induced by diet and microbiota-derived metabolites. Low-grade inflammation and microbiota-derived metabolites but also specific bacteria species can influence host metabolism in various ways which can result in metabolic syndrome-like conditions, T2DM, and CVD. Host metabolism is modulated either beneficially or adversely by microbiota-related metabolites such as SCFA, secondary bile acids, TMAO, or by the GM composition and function per se. Targeting of these metabolism modulators showed promising results to improve cardiometabolic disease markers, paving the way for new prevention and treatment strategies. However, most research is based on animal models while human research on this topic is scarce and associative of nature. Furthermore, most human studies did not focus on clinically relevant outcome measures but on associative biomarkers of CMD. Therefore, more translational research in humans is necessary to confirm the beneficial effects of GM interventions for CMD with the focus on clinically relevant outcome measures.
7. Expert opinion
The pathophysiology of the different CMD is similar and the link between GM and CMD is becoming more cleare due to recent studies. Several animal studies showed promising results in alleviating or even reversing metabolic disorders and atherosclerosis. This could have new implications for diagnostic and therapeutic interventions, although evidence in human studies remains scarce. Recent studies provide insights into the pathophysiology and risk factors of CMD. A high-fat diet, low-grade inflammation, and several metabolites can modulate risk factors for CMD in host metabolism through various pathways and initiate the onset of CMD. However, much of the underlying effects are not fully explained and mostly studied in animal models. Therefore, more research is necessary to understand the complex mechanisms by which GM metabolites influence the risk of CMD through modulation of inflammatory pathways as well as host glucose and lipid metabolism. Known metabolites with beneficial or detrimental effects can already be influenced by interventions on GM. For example, TMAO levels can be lowered by targeting production enzymes, diet, and lifestyle, but it is still not clear if TMAO should be used as a therapeutic or diagnostic marker. Bile acid signaling can be influenced by altering GM composition or targeting specific enzymes. Direct modulation of GM by the use of antibiotics, probiotics, or FMT also changes host metabolism. This knowledge provides opportunities for novel interventions.
If validation of previously found results is possible in humans, optimizing the composition of GM and its related metabolites to enhance their beneficial effects could further improve cardiometabolic health. If GM signatures, GM metabolites, or reliable biomarkers can be found that allow for identification of patients at risk for CMD. Modulation of these markers could then lead to new effective preventive, diagnostic, and therapeutic strategies against CMD. There is still much to study, for example, the signature of healthy GM is still unknown until so far. And given the vast amount of different species in GM, it can be expected that we are only beginning to see the ‘tip of the iceberg’, with more individual metabolites that modulate host metabolism to be identified. Providing new opportunities to influence GM to modulate CMD. Several important metabolites are already identified that modulate host metabolism using high-throughput analysis methods such as metabolomics. The search for more important signaling molecules could be extended to methods being used less frequently in the current field such as large sample proteomics analyses of the blood, to broaden the perspective of involved mechanisms between GM and host metabolism interactions resulting in CMD. This could contribute to act against the global increase of CMD. Metagenomic analysis of the GM composition using genome sequencing methods are currently still costly endeavors. However, if this method would be used on a broader level, commercialization would decrease the costs significantly, making broad application of this method more feasible, provided that a reference standard for healthy and unhealthy GM compositions can be formulated. A new approach could also focus on the difference in composition of microbiota in different areas of the intestines, including the small intestine. As metabolites are produced in different areas of the intestines, interventions specific for local microbiota composition could be tailored which might provide unique therapeutic targets. For this, the use of capsules that dispense their content only in their target organ, for example, the small intestines or colon, could be used to deliver the therapeutic agent. In addition to this, research on alternative microbes outside of the bacterial kingdom such as fungi or viruses could contribute to a more comprehensive understanding of the endocrine function of the GM and possibly provide new therapeutic targets for CMD.
The first important step for future research is to validate animal studies in humans, as translatability from animal models resulted in varying degrees of success so far. If data can be validated in humans, a catalog of metabolites containing relevant effects on metabolism and diseases should be established. This could facilitate individualized treatment strategies, targeting specific conditions such as insulin resistance by a combination of novel therapeutic bacterial strains to treat hypercholesterolemia, hypertriglyceridemia, inflammation, or atherosclerosis. Personalized interventions can target patient-specific metabolic dysregulations to maximize treatment efficacy. Future studies should focus on exploration of the beneficial effects of combining therapies. For example, on TMAO depletion and modulation of bile acid signaling, to improve host metabolism dependent on individual metabolism dysregulations or GM dysbiosis of patients, moving toward GM-based personalized interventions to target CMD.
Article highlights
Gut microbiota (GM) are involved in inducing low-grade inflammation which contributes to the development of cardiometabolic diseases (CMD).
CMD such as metabolic syndrome (MS), type 2 diabetes mellitus (T2DM), and atherosclerosis can be induced by GM-derived metabolites in animal models.
Several GM-derived metabolites influence CMD such as short-chain fatty acids (SCFA), secondary bile acids, and trimethylamine N-oxide (TMAO). CMD are associated with GM dysbiosis, characterized by altered GM diversity and composition.
Atherosclerosis and factors involved in the development of atherosclerosis such as low-grade inflammation, MS and T2DM may be therapeutically targeted by influencing GM.
Novel strategies targeting GM to prevent or treat atherosclerosis include altering the production of SCFA, intermediates of secondary bile acid metabolism and TMAO. Current and potential initiatives to achieve this, e.g. using novel drugs or probiotics, will be discussed.
Declaration of interest
MV Warmbrunn is an owner of Nature Plus. M Nieuwdorp is a member of the scientific advisory board of Caelus Health. MV Warmbrunn is supported by a CVON In control grant (2018-27). M Nieuwdorp is supported by a ZONMW VIDI grant 2013 (016.146.327). Icons for figures were provided by Les Laboratoires Servier, the SMART (Servier Medical ART) image collection (https://smart.servier.com/). The authors have no other relevant affiliations or financial involvement with any organization or entity with a financial interest in or financial conflict with the subject matter or materials discussed in the manuscript apart from those disclosed.
Reviewer disclosures
Peer reviewers on this manuscript have no relevant financial or other relationships to disclose.
Additional information
Funding
References
- Balakumar P, Maung UK, Jagadeesh G. Prevalence and prevention of cardiovascular disease and diabetes mellitus. Pharmacol Res. 2016 Nov;113(Pt A):600–609. PubMed PMID: 27697647
- Aron-Wisnewsky J, Clement K. The gut microbiome, diet, and links to cardiometabolic and chronic disorders. Nat Rev Nephrol. 2016 Mar;12(3):169–181. PubMed PMID: 26616538
- Mortality GBD, Causes of Death C. Global, regional, and national age-sex specific all-cause and cause-specific mortality for 240 causes of death, 1990–2013: a systematic analysis for the Global Burden of Disease Study 2013. Lancet. 2015 Jan 10;385(9963):117–171. PubMed PMID: 25530442; PubMed Central PMCID: PMCPMC4340604.
- Arroyo-Johnson C, Mincey KD. Obesity epidemiology worldwide. Gastroenterol Clin North Am. 2016 Dec;45(4): 571–579. PubMed PMID: 27837773; PubMed Central PMCID: PMCPMC5599163.
- World Health Organization. Global report on diabetes. 2016.
- Halter JB, Musi N, McFarland Horne F, et al. Diabetes and cardiovascular disease in older adults: current status and future directions. Diabetes. 2014 Aug;63(8):2578–2589. PubMed PMID: 25060886; PubMed Central PMCID: PMCPMC4113072.
- Benjamin EJ, Muntner P, Alonso A, et al. Heart disease and stroke statistics-2019 update: a report from the American Heart Association. Circulation. 2019 Mar 5;139(10):e56–e528. PubMed PMID: 30700139.
- Tang WHW, Backhed F, Landmesser U, et al. Intestinal microbiota in cardiovascular health and disease: JACC state-of-the-art review. J Am Coll Cardiol. 2019 Apr 30;73(16):2089–2105. PubMed PMID: 31023434; PubMed Central PMCID: PMCPMC6518422.
- Tilg H, Zmora N, Adolph TE, et al. The intestinal microbiota fuelling metabolic inflammation. Nat Rev Immunol. 2020;20(1):40–54. PubMed PMID: 31388093
- Qin J, Li Y, Cai Z, et al. A metagenome-wide association study of gut microbiota in type 2 diabetes. Nature. 2012 Oct 4;490(7418):55–60. PubMed PMID: 23023125.
- Karlsson FH, Tremaroli V, Nookaew I, et al. Gut metagenome in European women with normal, impaired and diabetic glucose control. Nature. 2013 Jun 6;498(7452):99–103. PubMed PMID: 23719380.
- Ley RE, Peterson DA, Gordon JI. Ecological and evolutionary forces shaping microbial diversity in the human intestine. Cell. 2006 Feb 24;124(4):837–848. PubMed PMID: 16497592.
- Qin J, Li R, Raes J, et al. A human gut microbial gene catalogue established by metagenomic sequencing. Nature. 2010 Mar 4;464(7285):59–65. PubMed PMID: 20203603; PubMed Central PMCID: PMCPMC3779803.
- Scott KP, Gratz SW, Sheridan PO, et al. The influence of diet on the gut microbiota. Pharmacol Res. 2013 Mar;69(1):52–60. PubMed PMID: 23147033.
- Sekirov I, Russell SL, Antunes LC, et al. Gut microbiota in health and disease. Physiol Rev. 2010 Jul;90(3):859–904. PubMed PMID: 20664075.
- Canfora EE, Jocken JW, Blaak EE. Short-chain fatty acids in control of body weight and insulin sensitivity. Nat Rev Endocrinol. 2015 Oct;11(10):577–591. PubMed PMID: 26260141
- Falony G, Vieira-Silva S, Raes J. Richness and ecosystem development across faecal snapshots of the gut microbiota. Nat Microbiol. 2018 May;3(5):526–528. 10.1038/s41564-018-0143-5. PubMed PMID: 29693658
- Tang WH, Kitai T, Hazen SL. Gut microbiota in cardiovascular health and disease. Circ Res. 2017 Mar 31;120(7):1183–1196. PubMed PMID: 28360349; PubMed Central PMCID: PMCPMC5390330.
- Smits SA, Leach J, Sonnenburg ED, et al. Seasonal cycling in the gut microbiome of the Hadza hunter-gatherers of Tanzania. Science. 2017 Aug 25;357(6353):802–806. PubMed PMID: 28839072; PubMed Central PMCID: PMCPMC5891123.
- De Filippis F, Pellegrini N, Vannini L, et al. High-level adherence to a Mediterranean diet beneficially impacts the gut microbiota and associated metabolome. Gut. 2016 Nov;65(11):1812–1821. PubMed PMID: 26416813.
- Wu GD, Chen J, Hoffmann C, et al. Linking long-term dietary patterns with gut microbial enterotypes. Science. 2011 Oct 7;334(6052):105–108. PubMed PMID: 21885731; PubMed Central PMCID: PMCPMC3368382.
- David LA, Maurice CF, Carmody RN, et al. Diet rapidly and reproducibly alters the human gut microbiome. Nature. 2014 Jan 23;505(7484):559–563. PubMed PMID: 24336217; PubMed Central PMCID: PMCPMC3957428.
- Yatsunenko T, Rey FE, Manary MJ, et al. Human gut microbiome viewed across age and geography. Nature. 2012 May 9;486(7402):222–227. PubMed PMID: 22699611; PubMed Central PMCID: PMCPMC3376388.
- Caesar R, Reigstad CS, Backhed HK, et al. Gut-derived lipopolysaccharide augments adipose macrophage accumulation but is not essential for impaired glucose or insulin tolerance in mice. Gut. 2012 Dec;61(12):1701–1707. PubMed PMID: 22535377; PubMed Central PMCID: PMCPMC3505865.
- Martinez-Medina M, Denizot J, Dreux N, et al. Western diet induces dysbiosis with increased E coli in CEABAC10 mice, alters host barrier function favouring AIEC colonisation. Gut. 2014 Jan;63(1):116–124. PubMed PMID: 23598352.
- Kopp W. How western diet and lifestyle drive the pandemic of obesity and civilization diseases. Diabetes Metab Syndr Obes. 2019;12:2221–2236. PubMed PMID: 31695465; PubMed Central PMCID: PMCPMC6817492
- Sottero B, Gargiulo S, Russo I, et al. Postprandial dysmetabolism and oxidative stress in Type 2 Diabetes: pathogenetic mechanisms and therapeutic strategies. Med Res Rev. 2015 Sep;35(5):968–1031. PubMed PMID: 25943420.
- Ley RE, Backhed F, Turnbaugh P, et al. Obesity alters gut microbial ecology. Proc Natl Acad Sci U S A. 2005 Aug 2;102(31):11070–11075. PubMed PMID: 16033867; PubMed Central PMCID: PMCPMC1176910.
- Le Chatelier E, Nielsen T, Qin J, et al. Richness of human gut microbiome correlates with metabolic markers. Nature. 2013 Aug 29;500(7464):541–546. PubMed PMID: 23985870.
- Cotillard A, Kennedy SP, Kong LC, et al. Dietary intervention impact on gut microbial gene richness. Nature. 2013 Aug 29;500(7464):585–588. PubMed PMID: 23985875.
- Arany Z, Neinast M. Branched chain amino acids in metabolic disease. Curr Diab Rep. 2018 Aug 15;18(10):76. 10.1007/s11892-018-1048-7. PubMed PMID: 30112615
- Allin KH, Tremaroli V, Caesar R, et al. Aberrant intestinal microbiota in individuals with prediabetes. Diabetologia. 2018 Apr;61(4):810–820. 10.1007/s00125-018-4550-1. PubMed PMID: 29379988; PubMed Central PMCID: PMCPMC6448993
- Liu Y, Wang Y, Ni Y, et al. Gut microbiome fermentation determines the efficacy of exercise for diabetes prevention. Cell Metab. 2019 Nov 27. DOI:10.1016/j.cmet.2019.11.001. [ PubMed PMID: 31786155].
- Pedersen HK, Gudmundsdottir V, Nielsen HB, et al. Human gut microbes impact host serum metabolome and insulin sensitivity. Nature. 2016 Jul 21;535(7612):376–381. PubMed PMID: 27409811.
- Caesar R, Tremaroli V, Kovatcheva-Datchary P, et al. Crosstalk between Gut microbiota and dietary lipids aggravates WAT inflammation through TLR signaling. Cell Metab. 2015 Oct 6;22(4):658–668. PubMed PMID: 26321659; PubMed Central PMCID: PMCPMC4598654.
- Meessen ECE, Warmbrunn MV, Nieuwdorp M, et al. Human postprandial nutrient metabolism and low-grade inflammation: a narrative review. Nutrients. 2019 Dec 7;11(12):3000. PubMed PMID: 31817857.
- Smith JD, Borel AL, Nazare JA, et al. Visceral adipose tissue indicates the severity of cardiometabolic risk in patients with and without type 2 diabetes: results from the INSPIRE ME IAA study. J Clin Endocrinol Metab. 2012 May;97(5):1517–1525. PubMed PMID: 22337910.
- van den Munckhof ICL, Kurilshikov A, Ter Horst R, et al. Role of gut microbiota in chronic low- grade inflammation as potential driver for atherosclerotic cardiovascular disease: a systematic review of human studies. Obes Rev. 2018 Dec;19(12):1719–1734. PubMed PMID: 30144260.
- Mikelsaar M, Stsepetova J, Hutt P, et al. Intestinal Lactobacillus sp. is associated with some cellular and metabolic characteristics of blood in elderly people. Anaerobe. 2010 Jun;16(3):240–246. PubMed PMID: 20223288.
- Furet JP, Kong LC, Tap J, et al. Differential adaptation of human gut microbiota to bariatric surgery-induced weight loss: links with metabolic and low-grade inflammation markers. Diabetes. 2010 Dec;59(12):3049–3057. PubMed PMID: 20876719; PubMed Central PMCID: PMCPMC2992765.
- Aron-Wisnewsky J, Prifti E, Belda E, et al. Major microbiota dysbiosis in severe obesity: fate after bariatric surgery. Gut. 2019 Jan;68(1):70–82. PubMed PMID: 29899081.
- Cani PD, Amar J, Iglesias MA, et al. Metabolic endotoxemia initiates obesity and insulin resistance. Diabetes. 2007 Jul;56(7):1761–1772. PubMed PMID: 17456850.
- Vreugdenhil AC, Rousseau CH, Hartung T, et al. Lipopolysaccharide (LPS)-binding protein mediates LPS detoxification by chylomicrons. J Immunol. 2003 Feb 1;170(3):1399–1405. PubMed PMID: 12538700.
- Neal MD, Leaphart C, Levy R, et al. Enterocyte TLR4 mediates phagocytosis and translocation of bacteria across the intestinal barrier. J Immunol. 2006 Mar 1;176(5):3070–3079. PubMed PMID: 16493066.
- Saad MJ, Santos A, Prada PO. Linking gut microbiota and inflammation to obesity and insulin resistance. Physiology (Bethesda). 2016 Jul;31(4):283–293. PubMed PMID: 27252163
- Horton F, Wright J, Smith L, et al. Increased intestinal permeability to oral chromium (51 Cr) - EDTA in human Type 2 diabetes. Diabet Med. 2014 May;31(5):559–563. PubMed PMID: 24236770.
- Genser L, Aguanno D, Soula HA, et al. Increased jejunal permeability in human obesity is revealed by a lipid challenge and is linked to inflammation and type 2 diabetes. J Pathol. 2018 Oct;246(2):217–230. PubMed PMID: 29984492.
- Cani PD. Human gut microbiome: hopes, threats and promises. Gut. 2018 Sep;67(9):1716–1725. PubMed PMID: 29934437; PubMed Central PMCID: PMCPMC6109275
- Cao X. Self-regulation and cross-regulation of pattern-recognition receptor signalling in health and disease. Nat Rev Immunol. 2016 Jan;16(1):35–50. PubMed PMID: 26711677
- Steimle A, Autenrieth IB, Frick JS. Structure and function: lipid A modifications in commensals and pathogens. Int J Med Microbiol. 2016 Aug;306(5):290–301. PubMed PMID: 27009633
- Li Y, Wang Z, Chen J, et al. Influence of lipid A acylation pattern on membrane permeability and innate immune stimulation. Mar Drugs. 2013 Aug 26;11(9):3197–3208. PubMed PMID: 24065161; PubMed Central PMCID: PMCPMC3806461.
- Boucher J, Kleinridders A, Kahn CR. Insulin receptor signaling in normal and insulin-resistant states. Cold Spring Harb Perspect Biol. 2014 Jan 1;6(1):a009191–a009191. PubMed PMID: 24384568; PubMed Central PMCID: PMCPMC3941218.
- Osborn O, Olefsky JM. The cellular and signaling networks linking the immune system and metabolism in disease. Nat Med. 2012 Mar 6;18(3):363–374. PubMed PMID: 22395709.
- Kim JJ, Sears DD. TLR4 and insulin resistance. Gastroenterol Res Pract. 2010;2010:1–11. PubMed PMID: 20814545; PubMed Central PMCID: PMCPMC2931384
- Cipriani S, Mencarelli A, Palladino G, et al. FXR activation reverses insulin resistance and lipid abnormalities and protects against liver steatosis in Zucker (fa/fa) obese rats. J Lipid Res. 2010 Apr;51(4):771–784. PubMed PMID: 19783811; PubMed Central PMCID: PMCPMC2842143.
- Gineste R, Sirvent A, Paumelle R, et al. Phosphorylation of farnesoid X receptor by protein kinase C promotes its transcriptional activity. Mol Endocrinol. 2008 Nov;22(11):2433–2447. PubMed PMID: 18755856.
- Ryan PM, Stanton C, Caplice NM. Bile acids at the cross-roads of gut microbiome-host cardiometabolic interactions. Diabetol Metab Syndr. 2017;9:102. PubMed PMID: 29299069; PubMed Central PMCID: PMCPMC5745752
- Koh A, Molinaro A, Stahlman M, et al. Microbially produced imidazole propionate impairs insulin signaling through mTORC1. Cell. 2018 Nov 1;175(4):947–961 e17. PubMed PMID: 30401435.
- Turnbaugh PJ, Ley RE, Mahowald MA, et al. An obesity-associated gut microbiome with increased capacity for energy harvest. Nature. 2006 Dec 21;444(7122):1027–1031. PubMed PMID: 17183312.
- Gao Z, Yin J, Zhang J, et al. Butyrate improves insulin sensitivity and increases energy expenditure in mice. Diabetes. 2009 Jul;58(7):1509–1517. PubMed PMID: 19366864; PubMed Central PMCID: PMCPMC2699871.
- Wong JM, de Souza R, Kendall CW, et al. Colonic health: fermentation and short chain fatty acids. J Clin Gastroenterol. 2006 Mar;40(3):235–243. PubMed PMID: 16633129.
- Cummings JH, Pomare EW, Branch WJ, et al. Short chain fatty acids in human large intestine, portal, hepatic and venous blood. Gut. 1987 Oct;28(10):1221–1227. PubMed PMID: 3678950; PubMed Central PMCID: PMCPMC1433442.
- Al-Lahham SH, Peppelenbosch MP, Roelofsen H, et al. Biological effects of propionic acid in humans; metabolism, potential applications and underlying mechanisms. Biochim Biophys Acta. 2010 Nov;1801(11):1175–1183. PubMed PMID: 20691280.
- Delzenne NM, Williams CM. Prebiotics and lipid metabolism. Curr Opin Lipidol. 2002 Feb;13(1):61–67. PubMed PMID: 11790964
- Peng L, He Z, Chen W, et al. Effects of butyrate on intestinal barrier function in a Caco-2 cell monolayer model of intestinal barrier. Pediatr Res. 2007 Jan;61(1):37–41. PubMed PMID: 17211138.
- Bordin M, D’Atri F, Guillemot L, et al. Histone deacetylase inhibitors up-regulate the expression of tight junction proteins. Mol Cancer Res. 2004 Dec;2(12):692–701. PubMed PMID: 15634758
- Tedelind S, Westberg F, Kjerrulf M, et al. Anti-inflammatory properties of the short-chain fatty acids acetate and propionate: a study with relevance to inflammatory bowel disease. World J Gastroenterol. 2007 May 28;13(20):2826–2832. PubMed PMID: 17569118; PubMed Central PMCID: PMCPMC4395634.
- Fukae J, Amasaki Y, Yamashita Y, et al. Butyrate suppresses tumor necrosis factor alpha production by regulating specific messenger RNA degradation mediated through a cis-acting AU-rich element. Arthritis Rheum. 2005 Sep;52(9):2697–2707. PubMed PMID: 16142751.
- den Besten G, Bleeker A, Gerding A, et al. Short-chain fatty acids protect against high-fat diet-induced obesity via a PPARgamma-dependent switch from lipogenesis to fat oxidation. Diabetes. 2015 Jul;64(7):2398–2408. PubMed PMID: 25695945.
- Vrieze A, Van Nood E, Holleman F, et al. Transfer of intestinal microbiota from lean donors increases insulin sensitivity in individuals with metabolic syndrome. Gastroenterology. 2012 Oct;143(4):913–6 e7. PubMed PMID: 22728514.
- Bouter K, Bakker GJ, Levin E, et al. Differential metabolic effects of oral butyrate treatment in lean versus metabolic syndrome subjects. Clin Transl Gastroenterol. 2018 May 25;9(5):155. 10.1038/s41424-018-0025-4. PubMed PMID: 29799027; PubMed Central PMCID: PMCPMC5968024
- Wu WK, Hsu CC, Sheen LY, et al. Measurement of gut microbial metabolites in cardiometabolic health and translational research. Rapid Commun Mass Spectrom. 2019 Jul 25. DOI:10.1002/rcm.8537. [ PubMed PMID: 31344762].
- Chen MX, Wang SY, Kuo CH, et al. Metabolome analysis for investigating host-gut microbiota interactions. J Formos Med Assoc. 2019 Mar;118 Suppl 1:S10–S22. PubMed PMID: 30269936.
- Vernocchi P, Del Chierico F, Putignani L. Gut microbiota profiling: metabolomics based approach to unravel compounds affecting human health. Front Microbiol. 2016;7:1144. PubMed PMID: 27507964; PubMed Central PMCID: PMCPMC4960240
- Chiang JY. Bile acids: regulation of synthesis. J Lipid Res. 2009 Oct;50(10):1955–1966. PubMed PMID: 19346330; PubMed Central PMCID: PMCPMC2739756
- Sayin SI, Wahlstrom A, Felin J, et al. Gut microbiota regulates bile acid metabolism by reducing the levels of tauro-beta-muricholic acid, a naturally occurring FXR antagonist. Cell Metab. 2013 Feb 5;17(2):225–235. PubMed PMID: 23395169.
- Ajouz H, Mukherji D, Shamseddine A. Secondary bile acids: an underrecognized cause of colon cancer. World J Surg Oncol. 2014 May 24;12:164. PubMed PMID: 24884764; PubMed Central PMCID: PMCPMC4041630.
- Parker KD, Albeke SE, Gigley JP, et al. Microbiome composition in both wild-type and disease model mice is heavily influenced by mouse facility. Front Microbiol. 2018;9:1598. PubMed PMID: 30079054; PubMed Central PMCID: PMCPMC6062620
- van Nierop FS, Scheltema MJ, Eggink HM, et al. Clinical relevance of the bile acid receptor TGR5 in metabolism. Lancet Diabetes Endocrinol. 2017 Mar;5(3):224–233. PubMed PMID: 27639537.
- Lefebvre P, Cariou B, Lien F, et al. Role of bile acids and bile acid receptors in metabolic regulation. Physiol Rev. 2009 Jan;89(1):147–191. PubMed PMID: 19126757.
- Hylemon PB, Zhou H, Pandak WM, et al. Bile acids as regulatory molecules. J Lipid Res. 2009 Aug;50(8):1509–1520. PubMed PMID: 19346331; PubMed Central PMCID: PMCPMC2724047.
- Miyazaki-Anzai S, Masuda M, Kohno S, et al. Simultaneous inhibition of FXR and TGR5 exacerbates atherosclerotic formation. J Lipid Res. 2018 Sep;59(9):1709–1713. PubMed PMID: 29976576; PubMed Central PMCID: PMCPMC6121929.
- Katsuma S, Hirasawa A, Tsujimoto G. Bile acids promote glucagon-like peptide-1 secretion through TGR5 in a murine enteroendocrine cell line STC-1. Biochem Biophys Res Commun. 2005 Apr 1;329(1):386–390. PubMed PMID: 15721318.
- Drucker DJ. The cardiovascular biology of glucagon-like Peptide-1. Cell Metab. 2016 Jul 12;24(1):15–30. PubMed PMID: 27345422.
- Broeders EP, Nascimento EB, Havekes B, et al. The bile acid chenodeoxycholic acid increases human brown adipose tissue activity. Cell Metab. 2015 Sep 1;22(3):418–426. PubMed PMID: 26235421.
- Fang S, Suh JM, Reilly SM, et al. Intestinal FXR agonism promotes adipose tissue browning and reduces obesity and insulin resistance. Nat Med. 2015 Feb;21(2):159–165. PubMed PMID: 25559344; PubMed Central PMCID: PMCPMC4320010.
- Zhang HM, Wang X, Wu ZH, et al. Beneficial effect of farnesoid X receptor activation on metabolism in a diabetic rat model. Mol Med Rep. 2016 Mar;13(3):2135–2142. PubMed PMID: 26782298.
- Joyce SA, MacSharry J, Casey PG, et al. Regulation of host weight gain and lipid metabolism by bacterial bile acid modification in the gut. Proc Natl Acad Sci U S A. 2014 May 20;111(20):7421–7426. PubMed PMID: 24799697; PubMed Central PMCID: PMCPMC4034235.
- Joyce SA, Gahan CG. Bile acid modifications at the microbe-host interface: potential for nutraceutical and pharmaceutical interventions in host health. Annu Rev Food Sci Technol. 2016;7:313–333. PubMed PMID: 26772409
- Kootte RS, Levin E, Salojarvi J, et al. Improvement of insulin sensitivity after lean donor feces in metabolic syndrome is driven by baseline intestinal microbiota composition. Cell Metab. 2017 Oct 3;26(4):611–619 e6. PubMed PMID: 28978426.
- de Groot P, Scheithauer T, Bakker GJ, et al. Donor metabolic characteristics drive effects of faecal microbiota transplantation on recipient insulin sensitivity, energy expenditure and intestinal transit time. Gut. 2019 May 30. DOI:10.1136/gutjnl-2019-318320. [ PubMed PMID: 31147381].
- Duboc H, Rajca S, Rainteau D, et al. Connecting dysbiosis, bile-acid dysmetabolism and gut inflammation in inflammatory bowel diseases. Gut. 2013 Apr;62(4):531–539. PubMed PMID: 22993202.
- Meigs JB, Wilson PW, Fox CS, et al. Body mass index, metabolic syndrome, and risk of type 2 diabetes or cardiovascular disease. J Clin Endocrinol Metab. 2006 Aug;91(8):2906–2912. PubMed PMID: 16735483.
- Falk E, Fernandez-Ortiz A. Role of thrombosis in atherosclerosis and its complications. Am J Cardiol. 1995 Feb 23;75(6):3B–11B. PubMed PMID: 7863969.
- Rafieian-Kopaei M, Setorki M, Doudi M, et al. Atherosclerosis: process, indicators, risk factors and new hopes. Int J Prev Med. 2014 Aug;5(8):927–946. PubMed PMID: 25489440; PubMed Central PMCID: PMCPMC4258672
- Koeth RA, Wang Z, Levison BS, et al. Intestinal microbiota metabolism of L-carnitine, a nutrient in red meat, promotes atherosclerosis. Nat Med. 2013 May;19(5):576–585. PubMed PMID: 23563705; PubMed Central PMCID: PMCPMC3650111.
- Wang Z, Klipfell E, Bennett BJ, et al. Gut flora metabolism of phosphatidylcholine promotes cardiovascular disease. Nature. 2011 Apr 7;472(7341):57–63. PubMed PMID: 21475195; PubMed Central PMCID: PMCPMC3086762.
- Wiedermann CJ, Kiechl S, Dunzendorfer S, et al. Association of endotoxemia with carotid atherosclerosis and cardiovascular disease: prospective results from the Bruneck Study. J Am Coll Cardiol. 1999 Dec;34(7):1975–1981. PubMed PMID: 10588212.
- Caesar R, Fak F, Backhed F. Effects of gut microbiota on obesity and atherosclerosis via modulation of inflammation and lipid metabolism. J Intern Med. 2010 Oct;268(4):320–328. PubMed PMID: 21050286
- Lehr HA, Sagban TA, Ihling C, et al. Immunopathogenesis of atherosclerosis: endotoxin accelerates atherosclerosis in rabbits on hypercholesterolemic diet. Circulation. 2001 Aug 21;104(8):914–920. PubMed PMID: 11514379.
- Koren O, Spor A, Felin J, et al. Human oral, gut, and plaque microbiota in patients with atherosclerosis. Proc Natl Acad Sci U S A. 2011 Mar;15(108 Suppl 1):4592–4598. PubMed PMID: 20937873; PubMed Central PMCID: PMCPMC3063583.
- Figuero E, Sanchez-Beltran M, Cuesta-Frechoso S, et al. Detection of periodontal bacteria in atheromatous plaque by nested polymerase chain reaction. J Periodontol. 2011 Oct;82(10):1469–1477. PubMed PMID: 21453047.
- Grayston JT, Kronmal RA, Jackson LA, et al. Azithromycin for the secondary prevention of coronary events. N Engl J Med. 2005 Apr 21;352(16):1637–1645. PubMed PMID: 15843666.
- Janeiro MH, Ramirez MJ, Milagro FI, et al. Implication of Trimethylamine N-Oxide (TMAO) in disease: potential biomarker or new therapeutic target. Nutrients. 2018 Oct 1;10(10):1398. PubMed PMID: 30275434; PubMed Central PMCID: PMCPMC6213249.
- van der Veen JN, Kennelly JP, Wan S, et al. The critical role of phosphatidylcholine and phosphatidylethanolamine metabolism in health and disease. Biochim Biophys Acta Biomembr. 2017 Sep;1859(9 Pt B):1558–1572. PubMed PMID: 28411170.
- Lang DH, Yeung CK, Peter RM, et al. Isoform specificity of trimethylamine N-oxygenation by human flavin-containing monooxygenase (FMO) and P450 enzymes: selective catalysis by FMO3. Biochem Pharmacol. 1998 Oct 15;56(8):1005–1012. PubMed PMID: 9776311.
- Bennett BJ, de Aguiar Vallim TQ, Wang Z, et al. Trimethylamine-N-oxide, a metabolite associated with atherosclerosis, exhibits complex genetic and dietary regulation. Cell Metab. 2013 Jan 8;17(1):49–60. PubMed PMID: 23312283; PubMed Central PMCID: PMCPMC3771112.
- Hai X, Landeras V, Dobre MA, et al. Mechanism of Prominent Trimethylamine Oxide (TMAO) accumulation in hemodialysis patients. PLoS One. 2015;10(12):e0143731. PubMed PMID: 26650937; PubMed Central PMCID: PMCPMC4674074
- Koeth RA, Lam-Galvez BR, Kirsop J, et al. l-Carnitine in omnivorous diets induces an atherogenic gut microbial pathway in humans. J Clin Invest. 2019 Jan 2;129(1):373–387. PubMed PMID: 30530985; PubMed Central PMCID: PMCPMC6307959.
- Tang WH, Wang Z, Levison BS, et al. Intestinal microbial metabolism of phosphatidylcholine and cardiovascular risk. N Engl J Med. 2013 Apr 25;368(17):1575–1584. PubMed PMID: 23614584; PubMed Central PMCID: PMCPMC3701945.
- Wang Z, Tang WH, Buffa JA, et al. Prognostic value of choline and betaine depends on intestinal microbiota-generated metabolite trimethylamine-N-oxide. Eur Heart J. 2014 Apr;35(14):904–910. PubMed PMID: 24497336; PubMed Central PMCID: PMCPMC3977137.
- Skagen K, Troseid M, Ueland T, et al. The Carnitine-butyrobetaine-trimethylamine-N-oxide pathway and its association with cardiovascular mortality in patients with carotid atherosclerosis. Atherosclerosis. 2016 Apr;247:64–69. PubMed PMID: 26868510.
- Heianza Y, Ma W, Manson JE, et al. Gut microbiota metabolites and risk of major adverse cardiovascular disease events and death: a systematic review and meta-analysis of prospective studies. J Am Heart Assoc. 2017 Jun 29;6(7). PubMed PMID: 28663251; PubMed Central PMCID: PMCPMC5586261. DOI:10.1161/JAHA.116.004947.
- Qi J, You T, Li J, et al. Circulating trimethylamine N-oxide and the risk of cardiovascular diseases: a systematic review and meta-analysis of 11 prospective cohort studies. J Cell Mol Med. 2018 Jan;22(1):185–194. PubMed PMID: 28782886; PubMed Central PMCID: PMCPMC5742728.
- Schiattarella GG, Sannino A, Toscano E, et al. Gut microbe-generated metabolite trimethylamine-N-oxide as cardiovascular risk biomarker: a systematic review and dose- response meta-analysis. Eur Heart J. 2017 Oct 14;38(39):2948–2956. PubMed PMID: 29020409.
- Gregory JC, Buffa JA, Org E, et al. Transmission of atherosclerosis susceptibility with gut microbial transplantation. J Biol Chem. 2015 Feb 27;290(9):5647–5660. PubMed PMID: 25550161; PubMed Central PMCID: PMCPMC4342477.
- Shimizu M, Cashman JR, Yamazaki H. Transient trimethylaminuria related to menstruation. BMC Med Genet. 2007 Jan 27;8:2. PubMed PMID: 17257434; PubMed Central PMCID: PMCPMC1790885.
- Lindskog Jonsson A, Caesar R, Akrami R, et al. Impact of gut microbiota and diet on the development of atherosclerosis in Apoe(-/-) mice. Arterioscler Thromb Vasc Biol. 2018 Oct;38(10):2318–2326. PubMed PMID: 29903735; PubMed Central PMCID: PMCPMC6166703.
- Wright SD, Burton C, Hernandez M, et al. Infectious agents are not necessary for murine atherogenesis. J Exp Med. 2000 Apr 17;191(8):1437–1442. PubMed PMID: 10770809; PubMed Central PMCID: PMCPMC2193142.
- Kiouptsi K, Jackel S, Pontarollo G, et al. The microbiota promotes arterial thrombosis in low- density lipoprotein receptor-deficient mice. mBio. 2019 Oct 22;10(5). PubMed PMID: 31641089; PubMed Central PMCID: PMCPMC6805995. DOI:10.1128/mBio.02298-19.
- Kasahara K, Tanoue T, Yamashita T, et al. Commensal bacteria at the crossroad between cholesterol homeostasis and chronic inflammation in atherosclerosis. J Lipid Res. 2017 Mar;58(3):519–528. PubMed PMID: 28130274; PubMed Central PMCID: PMCPMC5335582.
- Stepankova R, Tonar Z, Bartova J, et al. Absence of microbiota (germ-free conditions) accelerates the atherosclerosis in ApoE-deficient mice fed standard low cholesterol diet. J Atheroscler Thromb. 2010 Aug 31;17(8):796–804. PubMed PMID: 20379054.
- Zhu W, Gregory JC, Org E, et al. Gut microbial metabolite TMAO enhances platelet hyperreactivity and thrombosis risk. Cell. 2016 Mar 24;165(1):111–124. PubMed PMID: 26972052; PubMed Central PMCID: PMCPMC4862743.
- Seldin MM, Meng Y, Qi H, et al. Trimethylamine N-oxide promotes vascular inflammation through signaling of mitogen-activated protein kinase and nuclear factor-kappaB. J Am Heart Assoc. 2016 Feb 22;5(2). PubMed PMID: 26903003; PubMed Central PMCID: PMCPMC4802459. DOI:10.1161/JAHA.115.002767.
- Kaysen GA, Johansen KL, Chertow GM, et al. Associations of trimethylamine n-oxide with nutritional and inflammatory biomarkers and cardiovascular outcomes in patients new to dialysis. J Ren Nutr. 2015 Jul;25(4):351–356. PubMed PMID: 25802017; PubMed Central PMCID: PMCPMC4469547.
- Smits LP, Kootte RS, Levin E, et al. Effect of vegan fecal microbiota transplantation on carnitine- and choline-derived trimethylamine-N-oxide production and vascular inflammation in patients with metabolic syndrome. J Am Heart Assoc. 2018 Mar 26;7(7). PubMed PMID: 29581220; PubMed Central PMCID: PMCPMC5907601. DOI:10.1161/JAHA.117.008342.
- Emoto T, Yamashita T, Sasaki N, et al. Analysis of gut microbiota in coronary artery disease patients: a possible link between gut microbiota and coronary artery disease. J Atheroscler Thromb. 2016 Aug 1;23(8):908–921. PubMed PMID: 26947598.
- Jie Z, Xia H, Zhong SL, et al. The gut microbiome in atherosclerotic cardiovascular disease. Nat Commun. 2017 Oct 10;8(1):845. PubMed PMID: 29018189; PubMed Central PMCID: PMCPMC5635030.
- Aguilar EC, Leonel AJ, Teixeira LG, et al. Butyrate impairs atherogenesis by reducing plaque inflammation and vulnerability and decreasing NFkappaB activation. Nutr Metab Cardiovasc Dis. 2014 Jun;24(6):606–613. PubMed PMID: 24602606.
- Kasahara K, Krautkramer KA, Org E, et al. Interactions between Roseburia intestinalis and diet modulate atherogenesis in a murine model. Nat Microbiol. 2018 Dec;3(12):1461–1471. 10.1038/s41564-018-0272-x. PubMed PMID: 30397344; PubMed Central PMCID: PMCPMC6280189
- Meyer KA, Shea JW. Dietary choline and betaine and risk of CVD: a systematic review and meta-analysis of prospective studies. Nutrients. 2017 Jul 7;9(7):711. PubMed PMID: 28686188; PubMed Central PMCID: PMCPMC5537826.
- Arakawa M, Mita T, Azuma K, et al. Inhibition of monocyte adhesion to endothelial cells and attenuation of atherosclerotic lesion by a glucagon-like peptide-1 receptor agonist, exendin- 4. Diabetes. 2010 Apr;59(4):1030–1037. PubMed PMID: 20068138; PubMed Central PMCID: PMCPMC2844811.
- Craciun S, Balskus EP. Microbial conversion of choline to trimethylamine requires a glycyl radical enzyme. Proc Natl Acad Sci U S A. 2012 Dec 26;109(52):21307–21312. PubMed PMID: 23151509; PubMed Central PMCID: PMCPMC3535645.
- Wang Z, Roberts AB, Buffa JA, et al. Non-lethal inhibition of gut microbial trimethylamine production for the treatment of atherosclerosis. Cell. 2015 Dec 17;163(7):1585–1595. PubMed PMID: 26687352; PubMed Central PMCID: PMCPMC4871610.
- Chittim CL, Martinez Del Campo A, Balskus EP. Gut bacterial phospholipase Ds support disease-associated metabolism by generating choline. Nat Microbiol. 2019 Jan;4(1):155–163. 10.1038/s41564-018-0294-4. PubMed PMID: 30455469
- Washburn RL, Cox JE, Muhlestein JB, et al. Pilot study of novel intermittent fasting effects on metabolomic and trimethylamine N-oxide changes during 24-hour water-only fasting in the FEELGOOD trial. Nutrients. 2019 Jan 23;11(2):246. PubMed PMID: 30678028; PubMed Central PMCID: PMCPMC6412259.
- Erickson ML, Malin SK, Wang Z, et al. Effects of lifestyle intervention on plasma trimethylamine N-oxide in obese adults. Nutrients. 2019 Jan 16;11(1):179. PubMed PMID: 30654453; PubMed Central PMCID: PMCPMC6356515.
- Jackel S, Kiouptsi K, Lillich M, et al. Gut microbiota regulate hepatic von Willebrand factor synthesis and arterial thrombus formation via Toll-like receptor-2. Blood. 2017 Jul 27;130(4):542–553. PubMed PMID: 28572286.
- Karlsson FH, Fak F, Nookaew I, et al. Symptomatic atherosclerosis is associated with an altered gut metagenome. Nat Commun. 2012;3:1245. PubMed PMID: 23212374; PubMed Central PMCID: PMCPMC3538954
- Oliveira-Nascimento L, Massari P, Wetzler LM. The role of TLR2 in infection and immunity. Front Immunol. 2012;3:79. PubMed PMID: 22566960; PubMed Central PMCID: PMCPMC3342043
- Zhu W, Wang Z, Tang WHW, et al. Gut microbe-generated trimethylamine N-oxide from dietary choline is prothrombotic in subjects. Circulation. 2017 Apr 25;135(17):1671–1673. PubMed PMID: 28438808; PubMed Central PMCID: PMCPMC5460631.
- Zhang Y, Zheng X, Huang F, et al. Ursodeoxycholic acid alters bile acid and fatty acid profiles in a mouse model of diet-induced obesity. Front Pharmacol. 2019;10:842. PubMed PMID: 31402868; PubMed Central PMCID: PMCPMC6669341
- Chen YS, Liu HM, Lee TY. Ursodeoxycholic acid regulates hepatic energy homeostasis and white adipose tissue macrophages polarization in leptin-deficiency obese mice. Cells. 2019 Mar 16;8(3). PubMed PMID: 30884843; PubMed Central PMCID: PMCPMC6468643. DOI:10.3390/cells8030253
- Hartman HB, Gardell SJ, Petucci CJ, et al. Activation of farnesoid X receptor prevents atherosclerotic lesion formation in LDLR-/- and apoE-/- mice. J Lipid Res. 2009 Jun;50(6):1090–1100. PubMed PMID: 19174369; PubMed Central PMCID: PMCPMC2681391.
- Mencarelli A, Renga B, Distrutti E, et al. Antiatherosclerotic effect of farnesoid X receptor. Am J Physiol Heart Circ Physiol. 2009 Feb;296(2):H272–H281. PubMed PMID: 19028791.
- Jadhav K, Xu Y, Xu Y, et al. Reversal of metabolic disorders by pharmacological activation of bile acid receptors TGR5 and FXR. Mol Metab. 2018 Mar;9:131–140. PubMed PMID: 29361497; PubMed Central PMCID: PMCPMC5870099.
- Krishnan S, Ding Y, Saedi N, et al. Gut microbiota-derived tryptophan metabolites modulate inflammatory response in hepatocytes and macrophages. Cell Rep. 2018 Apr 24;23(4):1099–1111. PubMed PMID: 29694888; PubMed Central PMCID: PMCPMC6392449.
- Tobias DK, Lawler PR, Harada PH, et al. Circulating branched-chain amino acids and incident cardiovascular disease in a prospective cohort of US women. Circ Genom Precis Med. 2018 Apr;11(4):e002157. PubMed PMID: 29572205; PubMed Central PMCID: PMCPMC5880282.
- Wang X, He G, Peng Y, et al. Sodium butyrate alleviates adipocyte inflammation by inhibiting NLRP3 pathway. Sci Rep. 2015 Aug;3(5):12676. PubMed PMID: 26234821; PubMed Central PMCID: PMCPMC4522654.
- Vinolo MA, Rodrigues HG, Festuccia WT, et al. Tributyrin attenuates obesity-associated inflammation and insulin resistance in high-fat-fed mice. Am J Physiol Endocrinol Metab. 2012 Jul 15;303(2):E272–E282. PubMed PMID: 22621868.
- Guo CJ, Chang FY, Wyche TP, et al. Discovery of reactive microbiota-derived metabolites that inhibit host proteases. Cell. 2017 Jan 26;168(3):517–526 e18. PubMed PMID: 28111075; PubMed Central PMCID: PMCPMC5302092.
- Donia MS, Cimermancic P, Schulze CJ, et al. A systematic analysis of biosynthetic gene clusters in the human microbiome reveals a common family of antibiotics. Cell. 2014 Sep 11;158(6):1402–1414. PubMed PMID: 25215495; PubMed Central PMCID: PMCPMC4164201.
- Tahri K, Grille JP, Schneider F. Bifidobacteria strain behavior toward cholesterol: coprecipitation with bile salts and assimilation. Curr Microbiol. 1996;33(3):187–193.
- Nguyen TD, Kang JH, Lee MS. Characterization of Lactobacillus plantarum PH04, a potential probiotic bacterium with cholesterol-lowering effects. Int J Food Microbiol. 2007 Feb 15;113(3):358–361. PubMed PMID: 17140690.
- Qiu L, Tao X, Xiong H, et al. Lactobacillus plantarum ZDY04 exhibits a strain-specific property of lowering TMAO via the modulation of gut microbiota in mice. Food Funct. 2018 Aug 15;9(8):4299–4309. PubMed PMID: 30039147.
- Fang Y, Chen HQ, Zhang X, et al. Probiotic administration of lactobacillus rhamnosus GR-1 attenuates atherosclerotic plaque formation in ApoE-/- mice fed with a high-fat diet. Eur Rev Med Pharmacol Sci. 2019 Apr;23(8):3533–3541. PubMed PMID: 31081110.
- Costabile A, Buttarazzi I, Kolida S, et al. An in vivo assessment of the cholesterol-lowering efficacy of Lactobacillus plantarum ECGC 13110402 in normal to mildly hypercholesterolaemic adults. PLoS One. 2017;12(12):e0187964. PubMed PMID: 29228000; PubMed Central PMCID: PMCPMC5724841
- Everard A, Belzer C, Geurts L, et al. Cross-talk between Akkermansia muciniphila and intestinal epithelium controls diet-induced obesity. Proc Natl Acad Sci U S A. 2013 May 28;110(22):9066–9071. PubMed PMID: 23671105; PubMed Central PMCID: PMCPMC3670398.
- Li J, Lin S, Vanhoutte PM, et al. akkermansia muciniphila protects against atherosclerosis by preventing metabolic endotoxemia-induced inflammation in Apoe-/- mice. Circulation. 2016 Jun 14;133(24):2434–2446. PubMed PMID: 27143680.
- Plovier H, Everard A, Druart C, et al. A purified membrane protein from Akkermansia muciniphila or the pasteurized bacterium improves metabolism in obese and diabetic mice. Nat Med. 2017 Jan;23(1):107–113. PubMed PMID: 27892954.
- Depommier C, Everard A, Druart C, et al. Supplementation with Akkermansia muciniphila in overweight and obese human volunteers: a proof-of-concept exploratory study. Nat Med. 2019 Jul;25(7):1096–1103. 10.1038/s41591-019-0495-2. PubMed PMID: 31263284; PubMed Central PMCID: PMCPMC6699990
- Hu XF, Zhang WY, Wen Q, et al. Fecal microbiota transplantation alleviates myocardial damage in myocarditis by restoring the microbiota composition. Pharmacol Res. 2019 Jan;139:412–421. PubMed PMID: 30508676.
- Sun L, Pang Y, Wang X, et al. Ablation of gut microbiota alleviates obesity-induced hepatic steatosis and glucose intolerance by modulating bile acid metabolism in hamsters. Acta Pharm Sin B. 2019 Jul;9(4):702–710. PubMed PMID: 31384531; PubMed Central PMCID: PMCPMC6664038.
- Carvalho BM, Guadagnini D, Tsukumo DML, et al. Modulation of gut microbiota by antibiotics improves insulin signalling in high-fat fed mice. Diabetologia. 2012 Oct;55(10):2823–2834. 10.1007/s00125-012-2648-4. PubMed PMID: 22828956
- Brunt VE, Gioscia‐Ryan RA, Richey JJ, et al. Suppression of the gut microbiome ameliorates age-related arterial dysfunction and oxidative stress in mice. J Physiol. 2019 May;597(9):2361–2378. PubMed PMID: 30714619; PubMed Central PMCID: PMCPMC6487935.
- Vrieze A, Out C, Fuentes S, et al. Impact of oral vancomycin on gut microbiota, bile acid metabolism, and insulin sensitivity. J Hepatol. 2014 Apr;60(4):824–831. PubMed PMID: 24316517.
- Reijnders D, Goossens GH, Hermes GD, et al. Effects of Gut microbiota manipulation by antibiotics on host metabolism in obese humans: a randomized double-blind placebo- controlled trial. Cell Metab. 2016 Jul 12;24(1):63–74. PubMed PMID: 27411009.
- Heianza Y, Zheng Y, Ma W, et al. Duration and life-stage of antibiotic use and risk of cardiovascular events in women. Eur Heart J. 2019 Apr;24(40):3838–3845. PubMed PMID: 31216010.