1. Introduction
The discovery of clustered regularly interspaced short palindromic repeats (CRISPR) and CRISPR-associated protein 9 (Cas9) and their subsequent development for gene modification has revolutionized many scientific fields. First identified as a primitive adaptive immune system found in bacteria and archaea [Citation1], the CRISPR/Cas9 system was quickly adapted to facilitate gene editing by providing a versatile system that allows for direct modification of specific sequences via site-specific Cas9 nuclease-mediated double-strand break (DSB) and utilization of the cellular DNA repair mechanisms to mutate/correct the break [Citation2,Citation3]. It has been used to manipulate and interrogate the genome of a wide range of organisms, including plants, Caenorhabditis elegans, zebrafish, Drosophila, vertebrates, and humans. The ability to create specific gene modifications has cemented the technology as a fundamental research approach. Various modifications of the system have allowed its use to be expanded to include creating a variety of specific site-directed genetic edits (point mutations, translocations, insertions, and deletions) and to conduct high-throughput genetic screening. Mutant Cas9 has been generated that either has less off-target cleavage [Citation4,Citation5], can only nick one strand [Citation6], or lacks the ability to cleave either DNA strand (deactivated Cas9 [dCas9]) [Citation7,Citation8]; the latter fused to reporters, gene activators, or inhibitor proteins is being utilized for dynamic visualization of genomic loci, gene activation or inhibition, or induction of specific epigenetic repressive/activation marks. More recently, dCas9 fused to cytidine deaminase has been used to directly convert cytidine to uracil (C → T) without causing any DNA cleavage, which can correct specific point mutations [Citation9]. The CRISPR/Cas9 technology is now poised to translate to clinical trials and can potentially advance clinical care by manipulating gene expression in humans, from embryonic through somatic cells. This advance holds promise for treating inherited Mendelian disorders of the immune system, as well as complex traits including allergic diseases. Herein, we will review the CRISPR/Cas9 system, its use to date, and its potential applicability to human allergic and immunologic diseases.
2. Applications of CRISPR/Cas9 for genome editing and transcription modulation
The engineered CRIPSR/Cas9 system utilizes a chimeric single-guide RNA (sgRNA) to direct the Streptococcus pyogenes Cas9 (SpCas9) endonuclease to a target DNA sequence, a DSB is induced using its RuvC and HNH nuclease domains (). The sgRNA is a fusion of a CRISPR-targeting RNA (crRNA) array, containing a 20-nucleotide guide sequence, a short direct repeat, and an auxiliary trans-activating crRNA (tracrRNA). In addition to the originally described SpCas9, additional Cas9 orthologs from other bacteria (e.g. Staphylococcus aureus Cas9 [Citation10] and Cpf1 [Citation11]) have comparable cleavage efficiency to and higher specificity than SpCas9.
Figure 1. Basic CRISPR/Cas9 systems for allergic and immunologic diseases. (a) Conventional CRISPR/Cas9 system for gene editing. sgRNA and Cas9 form a ribonucleoprotien complex. sgRNA target sequence is complementary to a specific genomic location and allows binding of the RNP at that loci. Cas9 then creates a double-strand break. Cellular DNA repair mechanisms repair the break. A proportion of these repairs will result in gene knockouts or, if a donor DNA sequence is provided, point mutations or large insertions. Donor DNA sequences contain the desired change flanked by regions homologous to the DNA sequence proximal and distal to the genomic mutation site. Deactivated Cas9 (dCas9) systems can be used to modulate gene expression. Various enhancers or repressors can be fused to Cas9 itself (b), or aptamer technology can be used to allow binding of an enhancer or repressor to the sgRNA (c). After the RNP binds to a specific locus, the enhancer or repressor can modulate the expression of a nearby gene. (d) Using CRISPR/Cas9-aptamer-based gene regulation, it should be possible to achieve multiplex modulation of the expression of transcription factors and cytokine mediators, allowing for repression of the Type 2 T helper (Th2) phenotype associated with atopic disease and promoting development of either a Type 1 T helper (Th1) or a regulatory T cell (Treg) response. Diagrammed are potential targets for such a system. For example, GATA binding protein 3 (GATA-3) is a transcription factor important in the development of Th2 cells and forkhead box p3 (FoxP3) is a transcription factor important in the development of Treg cells. Using CRISPR/Cas9 to repress (blue minus sign) GATA-3 or induce (orange plus sign) FoxP3 expression, it may be possible to skew T cell development away from Th2 development and towards Treg development, respectively. T-box transcription factor TBX21 (T-bet), interleukin 4 (IL-4), interleukin 5 (IL-5), interleukin 13 (IL-13), interferon γ (IFN-γ), interleukin 2 (IL-2), cytotoxic T lymphocyte–associated protein 4 (CTLA-4), interleukin 10 (IL-10), transforming growth factor β (TGF-β), peripheral blood mononuclear cell (PBMC).
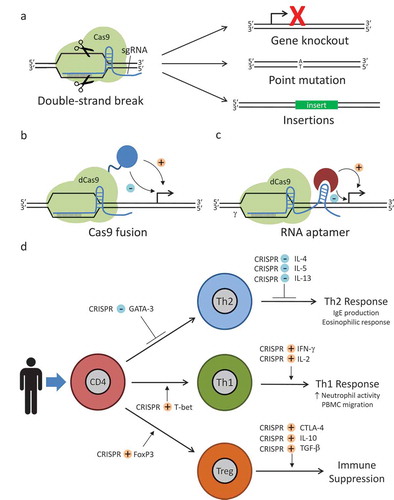
One limitation of the CRISPR/Cas9 system is off-target mutations caused by SpCas9 at regions of partial guide RNA (gRNA) complementarity. To overcome this, Cas9 nickase (Cas9n) [Citation6] and dCas9 fused to the dimerization-dependent, FokI nuclease domain [Citation7,Citation8] were created by introducing D10A and H840A mutations into one or both of the nuclease domains of SpCas9, respectively, so that two gRNAs targeting different strands are required to create a DSB, thereby increasing the specificity. Moreover, a modified SpCas9 [Citation12], which increases the number of targetable loci, and an enhanced specificity SpCas9 [Citation4,Citation5], with substantially reduced off-target cleavage and robust on-target cleavage activity, have been generated. The CRISPR/Cas9 system has been largely used for gene editing of in vitro and animal models of human diseases including Duchenne muscular dystrophy [Citation13–Citation16], cystic fibrosis [Citation17], β-thalassemia [Citation18], cataract [Citation19], and hereditary tyrosinemia type I [Citation20]. The CRISPR/Cas9 system has also been successfully utilized to disrupt DNA viruses, such as herpes simplex virus 1 [Citation21], human immunodeficiency virus (HIV) [Citation22], and hepatitis B virus [Citation23].
Additionally, dCas9 can be used as a flexible, RNA-guided, DNA-binding platform for precise transcriptional control or inducing a repressive/activating epigenetic change () [Citation24]. Although dCas9 binding to promoter regions can moderately impede transcription by disrupting the activity of RNA polymerase, several groups have shown enhanced repression by fusing transcriptional repressor domains, including the Krüppel-associated box (KRAB), chromoshadow, WPRW domain of hes family bHLH transcription factor 1, and mSin3 interaction domain (SID4X), onto the carboxyl terminus of dCas9 [Citation25,Citation26], with KRAB–dCas9 fusion being the most effective at generating a repressive mark [Citation27]. dCas9 fused to transcriptional activators such as the activation domain of p65 or VP64 has also been demonstrated to moderately activate both endogenous and reporter genes in mammalian cells [Citation26,Citation28]. Even greater activation efficiency has been achieved with the SunTag array–dCas9 fusion system, which allows recruitment of multiple copies of VP64–single-chain variable fragment fusion system protein to one dCas9 [Citation29], and the tripartite activator VP64, p65, and R transactivator–dCas9 fusion system [Citation30]. In another approach, the synergistic activation mediator system was generated; the MS2 coat protein (MCP)-interacting RNA aptamers were added into sgRNA, enabling recruitment of the heat-shock factor 1–p65–MCP activator module to dCas9–VP64 [Citation31]. More recently, scaffold RNAs have been generated by incorporating orthogonally acting protein-binding RNA aptamers (MS2, PP7, or com) into sgRNA, which allows simultaneous transcription repression and activation [Citation32,Citation33]. The variety of repression and activation systems provides for precise tuning of gene expression to the desired level.
3. Current status of CRISPR/Cas9 in allergy and immunology
CRISPR/Cas9 gene-editing systems, despite originating only several years ago, have already facilitated many contributions to the field of immunology with significant preclinical results. Perhaps, the most notable of these has been devising CRISPR/Cas9-based systems as possible treatments for HIV infection. In general, the techniques focus on deleting and/or gene editing of C–C motif chemokine receptor 5 or C–X–C motif chemokine receptor 4 (coreceptors for HIV) and/or reactivating latent HIV infections [Citation34]. Chang et al. used CRISPR/Cas9 in induced pluripotent stem cells to develop an in vitro model of Janus kinase 3 (JAK3) deficiency, define a block in T-cell development, and subsequently demonstrate restoration of normal T-cell development after CRISPR/Cas9-mediated correction of the JAK3 deficiency [Citation35]. Kuo et al. have used CRISPR/Cas9 to correct mutations in CD40 ligand found in X-linked hyper immunoglobulin (Ig)M syndrome [Citation36]. Cheong et al. utilized a CRISPR/Cas9 system to induce class-switch recombination of the IgH chain into desired subclasses or as fragment antigen-binding (Fab) fragments with the goal of opening up more careful analysis of Ig subclasses and new methods to easily generate Fab fragments for research or therapeutic uses [Citation37]. Recently, the first two CRISPR/Cas9 clinical trials (a Chinese and an American) have received approval to use CRISPR/Cas9 techniques to engineer T cells to target cancer cells [Citation38]. The former aims to delete the PD-1 receptor gene to increase T-cell immunogenicity against lung cancer cells. The latter will be tested in a variety of cancer types, and in addition to PD-1 receptor gene deletion, it will insert a chimeric antigen receptor (CAR) that targets cancer cells and remove the endogenous T-cell receptor to improve functioning of the CAR.
In terms of using CRISPR/Cas9 to investigate allergic diseases, most work has focused on utilizing the technology to investigate the role of particular genes. For example, Chu et al. used CRISPR/Cas9 to knockout MUC18, also known as CD146 or melanoma cell adhesion molecule, in human primary nasal airway epithelial cells with a reduced interleukin 8 (IL-8) response upon Toll-like receptor agonist stimulation, suggesting a pro-inflammatory role for MUC18 in response to bacterial or viral stimulation [Citation39]. Moreover, CRISPR/Cas9 is rapidly becoming the primary tool to create mutant mouse models of diseases, including allergic and immunologic diseases, due to the ease, precision, and flexibility of this technique.
4. Future of CRISPR/Cas9 in allergy and immunology
With the ease of designing this DNA targeting technology and a variety of extremely useful technological adaptations, including epigenetic modification and multiplex gene regulation, CRISPR/Cas9 is poised to become a dominant methodology in the study and potential treatment of allergic and immunologic diseases. lists some of the basic CRISPR/Cas9 approaches and provides examples of their potential uses in clinical allergy and immunology. Its most straightforward utilization is for diseases with Mendelian inheritance. By simply changing the gRNA sequence, and a donor sequence, almost any locus in the genome can be targeted. This affords researchers enormous flexibility in generating disease models of Mendelian diseases or devising treatment approaches for genetic correction. This approach is highlighted by the studies on CD40L and JAK3 deficiencies noted above. These, and other studies, are helping to lay the groundwork for successful treatment of allergic and immunologic diseases with defined Mendelian etiology.
Table 1. Basic CRISPR/Cas9 systems with potential uses in clinical allergy and immunology.
In clinical practice, it has become increasingly more common to sequence anything from specific genes to whole genomes when establishing a diagnosis in patients with rare or complex presentations. It is not uncommon to observe mutations of unknown significance in these results. Utilizing CRISPR/Cas9, researchers are now able to recreate these mutations in established cell lines or mouse models, making analysis of these mutations far easier than it has been with previously available technologies. Additionally, CRISPR/Cas9 can be used to modify SNPs that contribute to allergic disease, initially starting off in experimental systems and then advancing to therapy.
In the case of primary immunodeficiencies, CRISPR/Cas9 and other gene-editing techniques have a distinct advantage over traditional gene therapy. In traditional gene therapy, a correct version of a gene is inserted semi-randomly into the genome of cells, leading to amelioration of disease (e.g. adenosine deaminase-severe combined immunodeficiency, X-linked SCID, or Wiskott–Aldrich syndrome). Though this approach may be sufficient for many immunodeficiencies, gain-of-function (GOF) mutations or genes under tight regulatory control require correction of the existing gene to restore normal function or regulation. Examples include X-linked agammaglobulinemia, signal transducer and activator of transcription 3 (STAT3) loss of function, STAT3 GOF, STAT1 GOF, or activated PI3K-δ syndrome. It should be noted that therapeutic efficacy of gene editing depends on a variety of factors, including editing efficiency, cell fitness, efficient delivery to target tissue, and specificity of editing [Citation40]. Though many of these factors represent significant obstacles, the fact that hematopoietic cells are the most common target for allergic and immunologic diseases is of a distinct benefit, as autologous hematopoietic stem cell transplant provides a method to engraft hematopoietic stem cells that have been corrected ex vivo, completely or partially replacing the hematopoietic compartment.
In applying CRISPR/Cas9 systems to complex allergic diseases, which can result from the interplay of genetic, epigenetic, and environmental factors, multiplex gene modulation systems will be of potential use (). For example, there are potential applications for asthma. Alveolar macrophages (AMs) and the mediators that they produce have been implicated in the pathogenesis of asthma [Citation41], and it is possible to perform gene therapy on AMs [Citation42] as a potential asthma therapy. Given the complex pathways involved in asthma pathology, multiple genes may need to be modulated in order to have a demonstrable effect on the overall response. Using one of the above multiplex strategies, a specific set of pro-inflammatory genes could be downregulated (e.g. CD80, CD86, IL-17, TNF, IL-1β, IL-8, etc.), while anti-inflammatory genes could be simultaneously upregulated (e.g. IL-10). Similar applications could be devised for other complex allergic diseases.
From simple Mendelian disorders to complex multifactorial diseases, there is no doubt that CRISPR/Cas9 systems will revolutionize both the investigation and treatment of allergic and immunologic diseases. Building upon the original design, multiplex epigenetic modifications and gene modulation are now possible, opening up new research and therapeutic potentials that are poised to transform the field.
Declaration of interest
The authors have no relevant affiliations or financial involvement with any organization or entity with a financial interest in or financial conflict with the subject matter or materials discussed in the manuscript. This includes employment, consultancies, honoraria, stock ownership or options, expert testimony, grants or patents received or pending, or royalties.
Additional information
Funding
References
- Horvath P, Barrangou R. CRISPR/Cas, the immune system of bacteria and archaea. Science. 2010;327(5962):167–170.
- Cong L, Ran FA, Cox D, et al. Multiplex genome engineering using CRISPR/Cas systems. Science. 2013;339(6121):819–823.
- Mali P, Yang L, Esvelt KM, et al. RNA-guided human genome engineering via Cas9. Science. 2013;339(6121):823–826.
- Kleinstiver BP, Pattanayak V, Prew MS, et al. High-fidelity CRISPR-Cas9 nucleases with no detectable genome-wide off-target effects. Nature. 2016;529:490–495.
- Slaymaker IM, Gao L, Zetsche B, et al. Rationally engineered Cas9 nucleases with improved specificity. Science. 2016;351(6268):84–88.
- Shen B, Zhang W, Zhang J, et al. Efficient genome modification by CRISPR-Cas9 nickase with minimal off-target effects. Nat Methods. 2014;11(4):399–402.
- Tsai SQ, Wyvekens N, Khayter C, et al. Dimeric CRISPR RNA-guided FokI nucleases for highly specific genome editing. Nat Biotechnol. 2014;32(6):569–576.
- Guilinger JP, Thompson DB, Liu DR. Fusion of catalytically inactive Cas9 to FokI nuclease improves the specificity of genome modification. Nat Biotechnol. 2014;32(6):577–582.
- Komor AC, Kim YB, Packer MS, et al. Programmable editing of a target base in genomic DNA without double-stranded DNA cleavage. Nature. 2016;533(7603):420–424.
- Ran FA, Cong L, Yan WX, et al. In vivo genome editing using Staphylococcus aureus Cas9. Nature. 2015;520(7546):186–191.
- Zetsche B, Gootenberg Jonathan S, Abudayyeh Omar O, et al. Cpf1 is a single RNA-guided endonuclease of a class 2 CRISPR-Cas system. Cell. 2015;163(3):759–771.
- Kleinstiver BP, Prew MS, Tsai SQ, et al. Engineered CRISPR-Cas9 nucleases with altered PAM specificities. Nature. 2015;523(7561):481–485.
- Long C, Amoasii L, Mireault AA, et al. Postnatal genome editing partially restores dystrophin expression in a mouse model of muscular dystrophy. Science. 2016;351(6271):400–403.
- Nelson CE, Hakim CH, Ousterout DG, et al. In vivo genome editing improves muscle function in a mouse model of Duchenne muscular dystrophy. Science. 2016;351(6271):403–407.
- Ousterout DG, Kabadi AM, Thakore PI, et al. Multiplex CRISPR/Cas9-based genome editing for correction of dystrophin mutations that cause Duchenne muscular dystrophy. Nat Commun. 2015;6:6244.
- Ousterout DG, Perez-Pinera P, Thakore PI, et al. Reading frame correction by targeted genome editing restores dystrophin expression in cells from Duchenne muscular dystrophy patients. Mol Ther. 2013;21(9):1718–1726.
- Schwank G, Koo BK, Sasselli V, et al. Functional repair of CFTR by CRISPR/Cas9 in intestinal stem cell organoids of cystic fibrosis patients. Cell Stem Cell. 2013;13(6):653–658.
- Xie F, Ye L, Chang JC, et al. Seamless gene correction of beta-thalassemia mutations in patient-specific iPSCs using CRISPR/Cas9 and piggyBac. Genome Res. 2014;24(9):1526–1533.
- Wu Y, Liang D, Wang Y, et al. Correction of a genetic disease in mouse via use of CRISPR-Cas9. Cell Stem Cell. 2013;13(6):659–662.
- Yin H, Xue W, Chen S, et al. Genome editing with Cas9 in adult mice corrects a disease mutation and phenotype. Nat Biotechnol. 2014;32(6):551–553.
- Bi Y, Sun L, Gao D, et al. High-efficiency targeted editing of large viral genomes by RNA-guided nucleases. PLoS Pathog. 2014;10(5):e1004090.
- Ebina H, Misawa N, Kanemura Y, et al. Harnessing the CRISPR/Cas9 system to disrupt latent HIV-1 provirus. Sci Rep. 2013;3:2510.
- Lin S-R, Yang H-C, Kuo Y-T, et al. The CRISPR/Cas9 system facilitates clearance of the intrahepatic HBV templates in vivo. Mol Ther Nucl Acids. 2014;3(8):e186.
- Dominguez AA, Lim WA, Qi LS. Beyond editing: repurposing CRISPR-Cas9 for precision genome regulation and interrogation. Nat Rev Mol Cell Biol. 2016;17(1):5–15.
- Konermann S, Brigham MD, Trevino AE, et al. Optical control of mammalian endogenous transcription and epigenetic states. Nature. 2013;500(7463):472–476.
- Gilbert LA, Larson MH, Morsut L, et al. CRISPR-mediated modular RNA-guided regulation of transcription in eukaryotes. Cell. 2013;154(2):442–451.
- Gilbert LA, Horlbeck MA, Adamson B, et al. Genome-scale CRISPR-mediated control of gene repression and activation. Cell. 2014;159(3):647–661.
- Mali P, Aach J, Stranges PB, et al. CAS9 transcriptional activators for target specificity screening and paired nickases for cooperative genome engineering. Nat Biotech. 2013;31(9):833–838.
- Tanenbaum ME, Gilbert LA, Qi LS, et al. A protein-tagging system for signal amplification in gene expression and fluorescence imaging. Cell. 2014;159(3):635–646.
- Chavez A, Scheiman J, Vora S, et al. Highly efficient Cas9-mediated transcriptional programming. Nat Methods. 2015;12(4):326–328.
- Konermann S, Brigham MD, Trevino AE, et al. Genome-scale transcriptional activation by an engineered CRISPR-Cas9 complex. Nature. 2015;517(7536):583–588.
- Zalatan JG, Lee ME, Almeida R, et al. Engineering complex synthetic transcriptional programs with CRISPR RNA scaffolds. Cell. 2015;160(1–2):339–350.
- Shechner DM, Hacisuleyman E, Younger ST, et al. Multiplexable, locus-specific targeting of long RNAs with CRISPR-display. Nat Methods. 2015;12(7):664–670.
- Wang CX, Cannon PM. The clinical applications of genome editing in HIV. Blood. 2016;127(21):2546–2552.
- Chang CW, Lai YS, Westin E, et al. Modeling human severe combined immunodeficiency and correction by CRISPR/Cas9-enhanced gene targeting. Cell Rep. 2015;12(10):1668–1677.
- Kuo C, Hoban M, Joglekar A, et al. Site specific gene correction of defects in CD40 ligand using the Crispr/Cas9 genome editing platform. In: 2015 AAAAI Annual Meeting; Houston, TX; 2015, AB17.
- Cheong T-C, Compagno M, Chiarle R. Editing of mouse and human immunoglobulin genes by CRISPR-Cas9 system. Nat Commun. 2016;7:10934.
- Cyranoski D. Chinese scientists to pioneer first human CRISPR trial. Nature. 2016;535(7613):476–477.
- Chu HW, Rios C, Huang C, et al. CRISPR-Cas9-mediated gene knockout in primary human airway epithelial cells reveals a proinflammatory role for MUC18. Gene Ther. 2015;22(10):822–829.
- Cox DB, Platt RJ, Zhang F. Therapeutic genome editing: prospects and challenges. Nat Med. 2015;21(2):121–131.
- Balhara J, Gounni AS. The alveolar macrophages in asthma: a double-edged sword. Mucosal Immunol. 2012;5(6):605–609.
- Suzuki T, Arumugam P, Sakagami T, et al. Pulmonary macrophage transplantation therapy. Nature. 2014;514(7523):450–454.