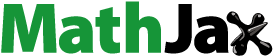
ABSTRACT
Similar to other cnidarians such as stony corals, the upside-down jellyfish (Cassiopea spp.) lives in endosymbiosis with dinoflagellates of the family Symbiodiniaceae. These jellyfish have been described as invasive species and are now found circumtropically in coastal marine environments. This study investigates the response of Cassiopea andromeda and its symbiotic algae to increased water temperature, further elucidating potential impacts of climate change on its populations in tropical ecosystems. It is demonstrated that water temperatures above 34°C are lethal for the C. andromeda medusae tested in this study. A non-lethal temperature rise triggers significant changes in the animals behaviour and physiology, including significant changes in bell pulsation rate and a significant decrease in photochemical efficiency, once 33°C is reached. Additionally, it is demonstrated that C. andromeda is capable of bioconcentrating significant amounts of cadmium from the surrounding water column. In the case of lead exposure, the jellyfish appear capable of regulating intake; no significant bioconcentration has been observed in this study. Our results do not indicate significant differences between the uptake patterns of anthropogenically complexed gadolinium chelates – emerging aquatic pollutants originating from hospital effluents – and the natural, non-chelated rare earth element terbium. This study supports the potential use of Cassiopea jellyfish as an attractive bioindicator species, serving to detect both continuous and pulse-contamination of coastal marine ecosystems. Cassiopea andromeda appears stable up until temperatures of about 34°C, suggesting that temperature increase concomitant with climate change might drive important range extensions of this tropical species into new environments – environments in which the holobiont will play significant roles in local pollutant dynamics.
Introduction
The epibenthic jellyfish Cassiopea spp. (class Scyphozoa, order Rhizostomeae) live in obligatory symbiosis with dinoflagellates of the family Symbiodiniaceae (zooxanthellae) and are distributed circumtropically from the Red Sea, over Australia and the Hawaiian Islands to Panama and Bermuda (Holland et al. Citation2004; Özgür and Öztürk Citation2008; Niggl and Wild Citation2009; Schembri et al. Citation2010; Karunarathne et al. Citation2020; Stampar et al. Citation2020). Restricted to the photic zone due to the dependence of their symbionts on photosynthetically active radiation, they inhabit shallow near-shore marine environments such as mangroves or seagrass-beds (Gohar and Eisawy Citation1960; Ohdera et al. Citation2018; Lyndby et al. Citation2020).
As a result of the coastal habitat, Cassiopea species are exposed to a variety of environmental stressors, including high light intensities and ultraviolet radiation, fluctuating water temperatures and salinities, as well as land-derived environmental pollutants such as heavy and trace metals or herbicides. A field study in Brazilian waters has observed Cassiopea thriving in a variety of environmental conditions, including large variations in salinity (0–36 PSU) and dissolved oxygen (7.01–15.56 mg/l) (Morandini et al. Citation2017). Though more research is required on the thermal tolerance of the organisms, it is clear that Cassiopea is a highly invasive tropical genus that may further extend its range with ongoing climate change and temperature increase. Cassiopea andromeda (Forskål, 1775) for example is native to the Red Sea and has been found to progressively invade the Mediterranean Sea (Schembri et al. Citation2010; Amor et al. Citation2016; Rubio Citation2017; Cillari et al. Citation2018; Maggio et al. Citation2019) which is attributed to high photosynthetic plasticity, thermal tolerance and mixotrophic behaviour (Mammone et al. Citation2021). Based on past developments, Cassiopea spp. range extension should be anticipated especially for anthropogenically impacted areas such as harbours or seafood farms. The biogeochemical impact that Cassiopea species populations might have in these systems – such as mobilization of sediment-locked pollutants including potential bioconcentration effects – is however unknown.
The question to what extent future temperature increase will drive further invasions mandates investigations of the holobionts’ thermal tolerance, key to which may be the zooxanthellae. Cassiopea’s symbiotic zooxanthellae significantly support the organism in meeting its carbon and nitrogen demands, complementing heterotrophic feeding on zooplankton and dissolved organic matter (Hofmann and Kremer Citation1981; Vodenichar Citation1995; Larson Citation1997; Freeman et al. Citation2017). Elevated temperatures have been shown to negatively affect Symbiodiniaceae’s photosynthesis both in and ex hospite, though thermotolerance widely differs between clades and strains of the dinoflagellate (Hoegh-Guldberg et al. Citation1998; McGinty et al. Citation2012; Karim et al. Citation2015; Díaz-Almeyda et al. Citation2017). Thermal stress and increased irradiance results in elevated production of reactive oxygen species (ROS), which can permanently damage both the symbionts and their cnidarian host and lead to expulsion of the endosymbionts, widely observed as coral bleaching (Tchernov et al. Citation2004; Smith et al. Citation2005).
Deploying Pulse-Amplitude-Modulation (PAM) fluorometry, numerous studies have demonstrated a decrease in the dinoflagellates’ dark-adapted maximum quantum yield (i.e. photochemical efficiency, Fv/Fm) of photosystem II under heat stress (Robinson and Warner Citation2006; Krämer et al. Citation2012; Díaz-Almeyda et al. Citation2017) both in culture and in hospite, under field and laboratory conditions (Fitt and Warner Citation1995; Bhagooli and Hidaka Citation2003), though the thermal-stress induced damage varies strongly between different Symbiodiniaceae (Karim et al. Citation2015).
Although the endosymbiotic supply of carbon and nitrogen are believed to meet most of Cassiopea’s energy requirements (Verde and McCloskey Citation1998; Freeman et al. Citation2017), medusae can actively perform heterotrophic feeding and external nutrient intake (Larson Citation1997). Given their relatively stagnant habitats, the jellyfish depend on bell pulsations for food capture processes, but also for oxygen exchange, incorporation of zooxanthellae, gamete dispersal and temperature regulation (Arai Citation1996; Welsh et al. Citation2009). The bell pulsation rate (BPR) vs. temperature relationships for warm-water jellyfish generally have a steep rise and fall on either side of a narrow temperature range in which BPR are highest (Gatz et al. Citation1973). To improve predictions on how climate change will impact species belonging to the genus Cassiopea, it is necessary to closer define the thermal window in which these cnidarians thrive.
A higher vulnerability to other environmental stressors can result once climate change moves the population closer to the upper boundary of its thermal window – or vice-versa. For example, Negri et al. have demonstrated that elevated sea surface temperatures significantly reduce the tolerance of coral larvae to water contamination of herbicides (Negri et al. Citation2011; Negri and Hoogenboom Citation2011), and a similar effect has been observed for reduced salinity (Klein et al. Citation2016). While such synergistic effects are yet to be described for Cassiopea species, they are indeed known to be adversely affected by herbicide exposure, which causes significant decreases in medusoid growth and symbiotic photosynthetic activity (Rowen et al. Citation2017).
While Cassiopea species are generally a relatively common sight in many tropical reef systems, they tend to occur in higher abundances in anthropogenically impacted waters such as shrimp farms, e.g. along the Brazilian coast, as has been reported by field studies and personal observations (Andreas Kunzmann, personal communication; Stoner et al. Citation2011; Thé et al. Citation2020). This is likely driven by high nutrient loadings or high availability of labile organic carbon (Rädecker et al. Citation2017). Field studies have suggested links between Cassiopea abundance and total phosphorus concentrations in waters of the Bahamas (Stoner et al. Citation2011) or with dissolved inorganic nitrogen in Pearl Harbor (Zarnoch et al. Citation2020). Motile, symbiont-bearing amoebocytes responsible for highly efficient nutrient uptake and transport have been singled out as a key adaptation rendering Cassiopea species successful in such waters (Lyndby et al. Citation2020).
The preponderance of the holobionts in anthropogenically impacted or polluted areas raises important questions with regard to the role of jellyfish in pollutant cycling, especially since Cassiopea is known to actively pump nutrients and interstitial waters from potentially contaminated sediments towards the surface (Jantzen et al. Citation2010; Zarnoch et al. Citation2020). If the Cassiopea thermal window is wide enough to allow for important range expansions into potentially contaminated areas with climate change, this might have significant impacts on local pollutant dynamics. Next to potentially mobilizing sediment-locked contaminants, jellyfish might transfer such compounds into the local food chain through bioconcentration and bioaccumulation. Templeman and Kingsford (Citation2015) have for example proven Cassiopea maremetens to be capable of bioconcentrating and bioaccumulating fine-scale pollutants such as copper and zinc with a bioconcentration factor (BCF) of 99 and 104 (Templeman and Kingsford Citation2010, Citation2015; Epstein et al. Citation2016). Bioconcentration dynamics of other relevant heavy metals such as cadmium (Cd) or lead (Pb) have not yet been investigated for Cassiopea species.
While examining bioconcentration is important with regards to impacts on the local environment and the food chain, it also holds great potential in the context of bioindicator species. Detecting anthropogenic pollution in natural ecosystems and particularly seawater matrices can be challenging and time consuming – here the use of bioindicator species naturally preconcentrating environmental loads of relevant pollutants can be useful. However, concentrations of heavy metals such as copper or lead cannot unambiguously point to anthropogenic impacts in a system, given that these elements show variable environmental behaviours and have both anthropogenic and natural sources. An interesting tool to unambiguously point to anthropogenic pollution in a system is Rare Earth Element (REE) geochemistry, specifically anomalies in normalized REE spectra. Since all REE exhibit very similar chemical behaviour in environmental systems due to their peculiar valence electron configuration (Thielmann Citation2011), anomalies in such spectra can point to specific geochemical or anthropogenic inputs or processes. For example, a geochemical signal that has unambiguously been traced back to anthropogenic pollution is a gadolinium (Gd) anomaly in the REE spectrum of a water sample (Bau and Dulski Citation1996). Gd is deployed in the production of Magnetic Resonance Imaging (MRI) contrast media such as ‘Gadovist’ or ‘Dotarem’, where Gd is chelated with strong organic ligands. Since these complexes pass through the human organism and waste-water treatment plants largely un-metabolized, Gd contrast agents (GDCA) end up in waterbodies worldwide (Bau and Dulski Citation1996; Möller et al. Citation2000; Verplanck et al. Citation2005). Anthropogenic Gd contamination has been detected in different rivers, bays and lakes of Europe (e.g. Bau and Dulski Citation1996; Kulaksiz and Bau Citation2011b), North and South America (e.g. Verplanck et al. Citation2005; Bau et al. Citation2006; Merschel et al. Citation2015), Asia (Nozaki et al. Citation2000) and Australia (Lawrence et al. Citation2009). As the chelates behave conservatively during estuarine mixing, the presence of GDCA in marine waters can point to the presence of other waste-water derived pollutants, which are more challenging to detect in seawater matrices (Kulaksiz and Bau Citation2011b). If the Gd anomaly is bioconcentrated in potential bioindicator organisms such as Cassiopea, this can provide a simple tool for tracing anthropogenic impacts in coastal marine environments. Prior to applying this proxy in a biogeochemical setting, it is however necessary to verify whether the target organism – in this case Cassiopea species – takes up the chelated Gd complex without fractionation relative to unchelated REE.
The goals of this study are based on the hypothesis that warmer temperature might drive future range expansions of Cassiopea into new, coastal ecosystems where the benthic holobionts may play important roles in local pollutant dynamics by mobilizing and preconcentrating contaminants from sediments into the surrounding waters or the food chain. Firstly, we aim at identifying Cassiopea andromeda’s upper temperature limits which will improve future assessments of possible range extensions as well as population dynamics in current habitats. Secondly, we will provide first insights into the bioconcentration of the two heavy metals lead (Pb) and cadmium (Cd), investigating whether bioconcentration occurs in a similar fashion as has been observed for Zn and Cu (Templeman and Kingsford Citation2015). Lastly, we will explore the possibility of using C. andromeda as a bioindicator species for pinpointing anthropogenic contamination in coastal marine ecosystems through bioconcentration of the REE Gd anomaly. We expect that results will improve our understanding of both C. andromeda’s thermal tolerance and responses to heavy metal exposure, two abiotic stressors that could be tightly linked in future oceans.
Materials and methods
For all experiments in this study Cassiopea andromeda medusae were sourced from a larger pool of jellyfish, kept for years in the Centre for Tropical Marine Research (ZMT) marine experimental ecology laboratory (MAREE) and originally sourced from the Red Sea. The organisms in this pool have been raised from ephyrae collected from a strobilating polyp culture at the facility. The polyps are kept in small aquaria (50 l) at 24°C and 31 PSU artificial seawater (Red Sea Salt, Germany) at low light conditions (single 20 W fluorescence bulb) with a 12:12 h light/dark cycle. Strobilation is achieved by raising temperature to 28°C and increasing salinity to 34–35 PSU. For maintenance in the MAREE, jellyfish and ephyrae are kept in recirculation systems with salinity 34–35 PSU, temperature 25.9 ± 0.1°C (12:12 h light/dark cycle, 120 µmol photons m−2 s−1). Feeding occurs twice weekly with freshly hatched Artemia nauplii.
Temperature-stress
Setup
This experiment aimed at studying the response of C. andromeda jellyfish to a gradual increase in water temperature. Twelve organisms (six each for the experimental and control groups, respectively) of medium size (starting bell diameter 7–9 cm) were held in individual plastic aquaria, equipped with plastic lids and ball-airstones. The aquaria were filled with artificial seawater to a final volume of 1.8 l (salinity 35.2 ± 0.8 PSU). Water was changed once a week, care was taken to maintain water temperature throughout the water change. Two halogen flood lamps per tank provided a stable illumination of 120 µmol photons m−2 s−1 ± 10% for a 12 h photoperiod. In the temperature stress experiment, organisms were starved from heterotrophic feeding and only provided with 7 µM NH4Cl once per week, spiked directly after water changes. Cassiopea holobionts are known to efficiently assimilate NH4 from seawater (Jantzen et al. Citation2010; Lyndby et al. Citation2020).
The water temperature was regulated by a larger recirculating water bath and a controllable heating rod. After a 10 days acclimatization period at 26°C, the temperature was raised 2°C once per week over a 5-week period, up to final temperature of 36°C. The temperature in the control group stayed constant at 26.1 ± 0.1°C.
To increase the resolution of the obtained data, the experiment (experiment 1, duration 29 days) was repeated (experiment 2, duration 44 days) with a temperature gradient of 1°C instead of 2°C per week, with all other experimental parameters remaining the same. Time constraints however precluded us to apply the 1°C gradient throughout the whole temperature range tested (26–35°C). Since experiment 1 indicated no change in BPR prior to and including 28°C, experiment 2 was designed to include a temperature ramping phase in which temperature was increased in 10 days from 26°C to 29°C. Once 29°C were reached, temperature was stepwise increased with a gradient of 1°C per week. The control group – 4 organisms in experiment 2 – was again kept at 26°C. The inclusion of a 10-day temperature ramping phase results in experiment 1 and experiment 2 not being directly comparable; this limitation is addressed in the Results section.
Temperature stress indicators
During the experiment, three parameters were recorded:
Bell Pulsation Rate (BPR), defined as the number of times the outer bell margin contracts towards the centre of the organism per minute, recorded twice weekly (day 3 and day 7 after temperature increase). Triplicate measurements for each of the medusae were taken at each time point using a mechanical counter and a stopwatch. BPR was recorded between 14:00 and 15:00 in the afternoon, prior to eventual manipulations for weight and/or photochemical efficiency measurements. BPR for each individual organism throughout the experiment were normalized to the organism’s initial BPR under no temperature stress at 26°C. This was done to account for the inherently high inter-organism variability in bell pulsation rates unrelated to external stress factors.
Maximum quantum yield (Fv/Fm) was recorded prior to the experiment and henceforth once every week (day 7 after temperature increase), using a portable WALZ GmbH PAM-2500 field and laboratory chlorophyll fluorometer and the corresponding PAMWin-3 Software. All organisms were dark-adapted for 15 min in a separate room before the measurement. Every organism was probed four times in succession at each time point – once in the centre and three times in the outer part of the bell.
Wet body weight was recorded once a week. These records were obtained by carefully removing the organisms from the water using an acid-washed borosilicate ladle, removing excess surface water and placing them into pre-weighed beakers filled with small volumes of artificial seawater. Body weight was recorded using a balance (analytical precision ± 0.1 g, Mettler Toledo, Germany).
Heavy-metal exposure
Setup
This experiment was designed to investigate whether C. andromeda jellyfish bioconcentrate the heavy metals Pb or Cd, as well as the REE Gd. Further, we tested whether artificially chelated Gd in the form of ‘Gadovist’ is taken up in a similar manner to non-complexed natural REE, in our case represented by Tb. The experimental set-up consisted of one control and two treatment groups, with three replicate tanks each:
Treatment A: Artificial seawater + 20 µg l−1 Pb, 800 ng l−1 Tb, 800 ng l−1 Gd (as ‘Gadovist’)
Treatment B: Artificial seawater + 4 µg l−1 Cd, 800 ng l−1 Tb, 800 ng l−1 Gd (as ‘Gadovist’)
Control: Artificial seawater
Figure 1. Experimental overview of the temperature stress experiments (A) and the heavy metal bioconcentration experiment (B). Further details are found in the Materials and methods section.
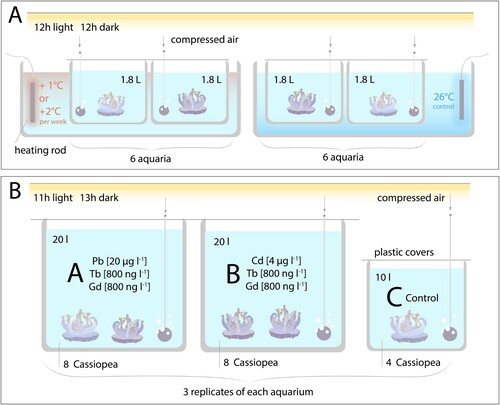
During the bioconcentration experiment, the organisms were held in large borosilicate aquaria containing 20 l of artificial seawater. Every aquarium was equipped with a ball-airstone and closed with an acrylic lid. All material was acid-washed and rinsed several times with artificial seawater before use. The aquaria were kept at room temperature (25°C) with illumination provided by halogen flood lamps from 08:00 am to 20:00 pm. Sixty small (mean bell diameter 3.2 ± 0.5 cm) organisms were randomly selected from the MAREE upbringing basin and allocated into the 9 test basins (8 per experimental tank, and 4 per control tank). These organisms were fed twice a week with freshly hatched Artemia nauplii (24 h). Prior to the beginning of the experiment, overall fitness of all medusae was assessed by a photochemical efficiency measurement (Fv/Fm), see above for details.
In order to minimize accumulation of jellyfish-derived organic matter that could interfere with the bioconcentration process and to continuously provide the organisms with the specified trace element concentrations, we performed complete water exchanges every two days. This was done by carefully introducing all organisms into aquaria with freshly spiked seawater medium using a borosilicate ladle.
Concentrated metal spike solutions were prepared in 0.5 M HNO3 using ‘Gadovist®’ originally purchased from Bayer HealthCare Pharmaceuticals (containing the macrocyclic complex Gadobutrol) and Pb, Cd or Tb 1000 mg l−1 single element standards (Inorganic Ventures, Germany).
Sample analysis for metal bioconcentration
Samples (water and organisms) were collected after 0, 4 and 7 days of exposure in acid-cleaned 50 ml polyethylene bottles. At every time point, all replicate tanks of both exposed and control treatments were randomly sampled, yielding a total of 27 jellyfish and 27 water samples (1 ml) over the course of the experiment. Jellyfish samples were collected using a borosilicate ladle. Before being sealed into their sampling bottles, jellyfish were carefully rinsed with deionized water, in order to avoid any bias from adhered contaminant water. After sampling, organisms were immediately frozen at −20°C and subsequently freeze-dried; dry weights (in g) were determined to the fourth decimal point using a microbalance (Mettler Toledo, Germany). The dried jellyfish samples were then subjected to an acid digestion that consisted of heating on a hotplate in PTFE beakers with 10 ml Suprapur grade 69% HNO3 for 2–3 h. Once incipient dryness had been reached, 5 ml Suprapur 30% HCl were added and again left for evaporation. The final residue was taken up in 10 ml Suprapur 0.5 M HNO3. Trace metal analysis was performed with a PerkinElmer Elan 6000 ICP-MS with mounted APEX Q inlet system, monitoring isotopes 206, 207 and 208 for Pb, 110, 111 and 112 for Cd, 158 and 160 for Gd and 159 for Tb. For the water samples, a dilution factor of 1:80 was set. An internal rhenium (Re), ruthenium (Ru), rhodium (Rh) and bismuth (Bi) standard was used in order to correct for matrix-induced changes in sensitivity. The data were corrected using Bi for Pb, Ru for Cd, and Re for Gd and Tb, respectively. Concentrations are reported as ng g−1 (dry weight). Reporting based on dry weight was chosen to allow for comparability with other studies in the literature. Metal concentrations in control jellyfish have been averaged to construct ‘baseline’ concentrations of medusa grown in artificial seawater without metal spike. These baseline concentrations from non-exposed organisms have been subtracted from all concentrations in the metal-exposed group. Bioconcentration factors (BCF) were calculated according to the standard formula:
Statistical analysis
All data treatment and statistical analysis was performed using R version 4.0.3. Repeated measurements (i.e. triplicate recording of BPR) were averaged for every medusa and sampling point before data analysis to preclude pseudoreplication. After normality testing by Shapiro–Wilk test, data from each of the two temperature stress experiments was analysed by two-way mixed analysis of variance (ANOVA) (including repeated measures for predictor time and non-repeated measures for predictor treatment), testing for sphericity using Mauchly’s test. In case of significant interaction effects, post-hoc unpaired two-sided t-tests were performed comparing stressed and control organisms on each measurement day. In the bioconcentration experiment, uptake patterns of Gd and Tb were compared using unpaired t-tests.
Results
Organism viability under temperature stress
The C. andromeda holobiont was significantly affected by the temperature stress, induced by stepping up the temperature from 26°C to 36°C by either 1 or 2°C once per week (). We used the bell pulsation rate as a marker for the viability of C. andromeda. The mean initial BPR of all 22 medusae used in the temperature stress experiments was 34 ± 12 SE, representing a standard variance of 34%, hence data are presented and discussed normalized to each medusa’s initial BPR for clarity. With temperature increase steps of 2°C every seven days (first experiment, A), maximum BPR (2.0 ± 0.2 SE relative to starting conditions) was attained after three days of 30°C exposure (day 11 of the experiment). With a further increase in temperature, BPR declined again, returning to the starting value (1.1 relative to starting conditions; ± 0.2 SE). A two-way mixed ANOVA for this first experiment suggested a significant interaction between temperature treatment and time, F(7, 70) = 3.54, P < 0.001. Unpaired two-tailed post-hoc t-tests revealed significant effects of temperature treatment on Cassiopea medusae on days 11 (3 days at 30°C) and 14 (7 days at 30°C), with significantly higher BPR than control organisms (P < 0.05).
Figure 2. A and B: Normalized bell pulsation rates (BPR) for temperature-stressed Cassiopea andromeda medusae (A, C and E: temperature gradient: 2°C/week, B, D and F: temperature gradient: 1°C/week) in orange and control (const. temperature 26°C) in grey. C and D: Symbiont maximum quantum yield (Fv /Fm) of C. andromeda medusae. Points represent average of triplicate (panels A and B) or quadruplicate (C and D) measurements for six replicate medusae at each time point with error bars representing the standard error. Shaded areas denote temperature recordings for both conditions. Measurement days on which the experimental group was significantly different from the control (unpaired one-sided t-test P < 0.05) are marked with an asterisk. E and F: C. andromeda wet weight normalized to initial weight, temperature stressed medusae (E: 2°C/week, F: 1°C/week) in orange and control (const. temperature 26°C) in grey. In all panels the vertical orange dashed line indicates the day of final ‘observation’ on which all 6 (experiment 1) or 3/6 (experiment 2) were found dead in the aquaria.
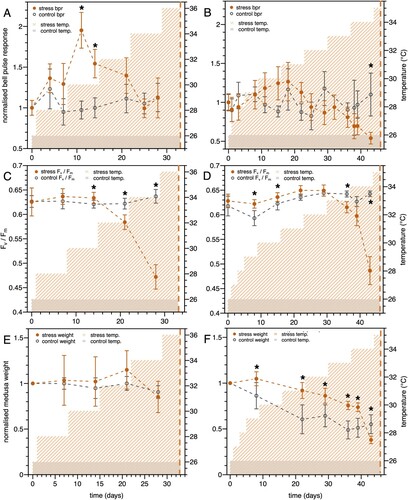
Using temperature increase steps of 1°C per seven days (second experiment, B) resulted in a similar trend, but lower BPR values than with 2°C per week. Maximum BPR was 1.3 ± 0.3 SE relative to starting values, 18 days into the experiment (three days of 31°C exposure), however this increase in BPR relative to the control was not statistically significant (P > 0.05). Nevertheless, a two-way mixed ANOVA did show significant interaction effects between temperature treatment and time, F(14, 112) = 2.53, P < 0.05. Post-hoc t-tests revealed this effect to be attributable to the last data point, day 43 of the experiment, where temperature-stressed medusae clearly showed significantly lower BPR than the control group (P < 0.05). A further increase in temperature to 35°C killed three of six temperature stress organisms (B and D); this marked the end of the experiment. Similar observations were made in experiment 1: when the temperature was increased by steps of 2°C per week, the final temperature increase from 34°C to 36°C (A and C) killed all six organisms within 1.5 days. In both experiments at 34°C and beyond, the jellyfish appeared to melt with oral arms drastically reduced in size, also leading to a significant loss of zooxanthellae. Bells were partially inverted, with very weak bell pulsations that were in some replicates one-sided only. Photos of an example temperature-stressed organism are shown in .
Figure 3. The same individual of Cassiopea andromeda after three days exposure to 33°C (1st row) and 34°C (2nd row).
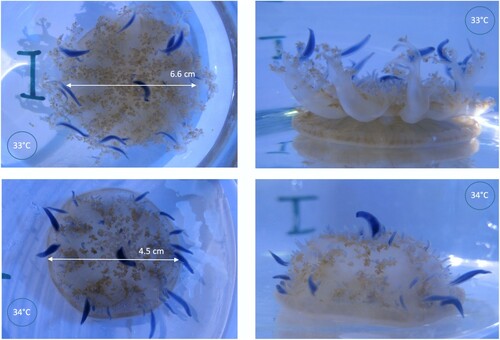
To determine the viability of symbionts associated with C. andromeda, we measured their photochemical efficiency (Fv/Fm; C and D). In both experiments, ANOVA analyses showed significant two-way interactions between temperature treatment and time (experiment 1: F(4, 40) = 46.3, P < 0.001, experiment 2: F(7, 56) = 21.0, P < 0.001). Post-hoc t-tests confirm the trends apparent in C and D: in both experiments, increased temperatures led to significant (P < 0.05) reductions of Fv/Fm in the symbionts. Up until 30°C, Fv/Fm is in both experimental rounds either similar or even significantly higher than in the control jellyfish.
With temperature increase steps of 2°C every week (C), further warming beyond 30°C resulted in a significant drop (P < 0.05) in photochemical efficiency of temperature-stressed symbionts, decreasing to a minimum of 0.47 ± 0.03 SE on day 28 of the experiment at 34°C. With temperature increase steps of 1°C per week, Fv/Fm values significantly dropped only at higher temperatures (≥ 33°C) to 0.49 ± 0.03 SE at 34°C.
Medusa weight developed differently in the two experiments. In experiment 1 (gradient 2°C/week), normalized medusa wet weight was in no case significantly different from that in the control group (E). Over the course of 28 days, the initial weight did not change significantly. In experiment 2 however (gradient 1°C/week), weights of both the control and the experimental group decreased over time. Jellyfish in experiment 2 were smaller than those in experiment 1 (experiment 1: control 35.3 ± 12.5 g, stress 37.1 ± 13.3 g vs. experiment 2: control 25.3 ± 8.7 g, stress: 30.0 ± 6.2 g), implying a lower contribution of zooxanthellae to animal respiration (CZAR) and hence stronger dependence on heterotrophic food sources. In both experiments a temperature increase to 34°C strongly decreased medusa weight in the treatment group (though significantly so only in experiment 2), as aforementioned jellyfish appeared to melt and lost oral arm structures. This melting precluded us from recording a final, decreased weight at 35°C/36°C.
We note that experiment 2 included a temperature ramping phase where temperature was increased from 26°C to 29°C within 10 days. This faster increase did not lead to any significant changes in the holobionts’ BPR, Fv/Fm or wet weight.
Bioconcentration of heavy and rare-earth metals
At the beginning of the metal-exposure experiment, medusae did not show any sign of impaired viability; their symbiotic algae showed a mean Fv/Fm value of 0.65 (± 0.04 SD).
To determine the bioconcentration factors of the different metals, we measured tissue and bulk water concentrations for metal-exposed and non-exposed (control) samples at 0, 4 and 7 days after exposure (). Mean tissue concentrations (± SD) of control organisms were 290 ± 73 ng g−1 dry weight (DW) for Pb, 433 ± 94 ng g−1 DW for Cd, 2 ± 1 ng g−1 DW for Gd and 0.3 ± 0.1 ng g−1 DW for Tb. A bulk water sample was analysed corresponding to every tissue sample. The mean water concentration of Pb was measured to be 20.3 ± 0.9 µg l−1, of Cd 3.0 ± 0.3 µg l−1, of Gd 0.60 ± 0.01 and 0.58 ± 0.04 µg l−1 (added with Pb or Cd, respectively), and of Tb 0.79 ± 0.03 and 0.76 ± 0.05 µg l−1 (added with Pb or Cd, respectively).
Figure 4. Bioconcentration of C. andromeda exposed to heavy and rare-earth metals. A: cadmium (Cd; green circles) or lead (Pb; purple circles); B: terbium (Tb; squares) and gadolinium (Gd; triangles) added with either Pb (purple) or Cd (green) in Cassiopea andromeda over time following exposure at day 0. Points and error bars represent average tissue concentrations and their standard deviations measured for triplicate organisms, respectively. Bioconcentration factors (BCF) refer to increases in concentrations in jellyfish divided by the bulk water concentrations after 7 days.
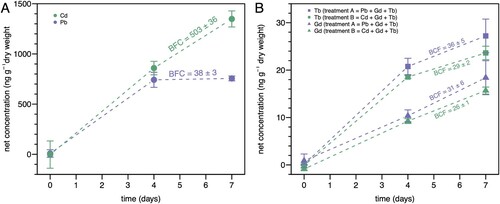
It is important to note that jellyfish are characterized by a particularly high water content when considering bioconcentration factors. In our study, we found the average water content of C. andromeda medusae to equal 96.2 (± 0.2) weight %. Hence in theory, a dry weight BCF of 26.3 ( = 100 g total weight/3.8 g dry weight) would be recorded if the organism did not actually concentrate any of the metal, i.e. if the metal concentration in the jellyfish total (dry + water) weight equalled the concentration in the bulk water.
Bioconcentration of lead (Pb) and cadmium (Cd)
In the tissues of Pb-exposed C. andromeda, we measured a mean (± SD) Pb net concentration of 742 ± 75 ng g−1 DW after four days of exposure; after three more days of exposure (seven total days) we measured a mean concentration of 756 ± 25 ng g−1 DW. This final tissue concentration, along with the initial tissue and final bulk water concentrations, yielded a BCF of 38 ± 3. Considering that the tissue concentrations are based on dry weight, this value represents a weak bioconcentration of Pb by C. andromeda under the given conditions (see above).
In contrast, we measured mean (± SD) Cd net concentrations of 860 ± 67 and 1349 ± 80 ng g−1 DW after 4 and 7 days of exposure, respectively, in the tissues of Cd-exposed C. andromeda. This final concentration corresponded to a BCF of 503 ± 36 (), suggesting significant bioconcentration of Cd by C. andromeda in this experiment.
Table I. Bioconcentration factors of spiked metals in Cassiopea andromeda.Table Footnotea
Bioconcentration of gadolinium (Gd) and terbium (Tb)
We exposed C. andromeda to both Gd and Tb, along with either Pb (‘treatment A’) or Cd (‘treatment B’). Tissue net concentrations (mean ± SD) of Gd added with Pb were 10 ± 1 and 18 ± 4 ng g−1 DW after 4 and 7 days, respectively. When added with Cd, the Gd tissue net concentrations were 9.2 ± 0.5 and 15.7 ± 0.7 ng g−1 DW after 4 and 7 days, respectively. These final concentrations corresponded to BCFs of 31 ± 6 and 26 ± 1 when added with Pb and Cd, respectively.
For Tb added with Pb, we measured tissue net concentrations of 21 ± 2 and 27 ± 4 ng g−1 after 4 and 7 days, respectively. The tissue net concentrations were 18.6 ± 0.3 and 24 ± 1 ng g−1 after 4 and 7 days, respectively, when added with Cd. Using these concentrations after 7 days, we calculated BCFs of 36 ± 5 and 29 ± 2 when added with Pb and Cd, respectively.
Overall, the BCFs for both Gd and Tb (irrespective of co-exposure with either Pb or Cd) were low, considering that tissue net metal concentrations are based on dry weight (see above). While the BCF for Tb was significantly higher (unpaired two-sided t-test: P < 0.05) than the one for Gd on day 4 of the uptake, this difference was not significant on day 7 anymore (unpaired two-sided t-test: P > 0.05). Generally, Gd and Tb accumulation was found to be small and no significant change in uptake pattern upon the presence of Cd or Pb in the water (B) was observed (both on day 4 and day 7 P > 0.05, unpaired one-tailed t-test).
Discussion
This study aimed at providing new insights on the role and fate of C. andromeda jellyfish in coastal, shallow-water environments. These environments are important Cassiopea species habitats and are disproportionally affected by human-induced stressors, including temperature increase as well as exposure to environmental pollutants. Depending on how it reacts to temperature increase, climate change might drive future range expansions of this holobiont, introducing it to new coastal environments, with different local biogeochemical cycles, including those of anthropogenic contaminants.
Aljbour et al. (Citation2017) found that a temperature increase up to 32°C appeared beneficial for acutely heat-treated C. andromeda, with the medusae acclimating well, including an increase in body mass and decrease in aerobic energy consumption. The present study found no increase in body mass, but significant changes in bell pulsation rates (BPR) and photochemical efficiency, when temperature was raised from the control temperature (26°C) up to 32°C. In this study jellyfish were not provided with a heterotrophic food source, but only supplied with ammonia for zooxanthellae. We observed an overall decrease in jellyfish weight in both the control and the experimental treatment in the second but not the first experiment. It is possible that this can be attributed to (i) the second experiment lasting longer than the first and (ii) the initial weight of organisms in the second experiment being lower than in the first, implying lower CZAR values and higher dependence on external nutrient input. Both experiments yielded significant reactions of jellyfish to temperature increase in terms of BPR and photochemical efficiency. We recorded maximum BPR at temperatures of 30°C–32°C. Faster temperature increase (2°C per week) resulted in rapid and pronounced increase of BPR, while more gradual temperature increase (1°C per week) resulted in a less pronounced increase of BPR. Other studies on different jellyfish species found either no correlation of BPR with temperature (Colin and Costello Citation2003) or correlation of BPR with salinity and bell diameter (Mayer Citation1914; Dillon Citation1977). When exposing the medusae in our study to further temperature increase beyond 32°C, BPR decreased. Temperatures of more than 34°C were lethal for the C. andromeda organisms in this study. With 39°C, the temperature threshold identified by Klein et al. for C. andromeda is higher than the one observed in this study (Klein et al. Citation2019). Comparability is however hindered by the fact that Klein et al. increased temperature by 2°C per day (instead of 2°C per 7 or 14 days as in our experiment), further the C. andromeda in this study had been raised and kept in aquaria prior to the experiment while the organisms in Klein et al., were sourced from a natural environment.
There is a considerable body of work investigating fluid dynamics of jellyfish pulsation (Hamlet et al. Citation2011; Hamlet and Miller Citation2012). In the common jellyfish Aurelia aurita, an increase in pulsation rate with water temperature is largely attributed to a growing metabolic rate (Avian et al. Citation1991). Verde and McCloskey (Citation1998) indeed found higher metabolic rates in Cassiopea xamachana at higher water temperatures (24.2°C vs. 29.9°C), however this has not been linked to external food intake. Numerical flow analyses have confirmed that Cassiopea bell pulsation generates feeding currents, though being distinct from those known in pelagic jellyfish (Hamlet and Miller Citation2012; Santhanakrishnan et al. Citation2012).
When BPR is moderate and pauses are present between contractions, vortices dissipate and move around the oral arms. In the case of continuous contraction (high BPR), vortices are stronger and are advected vertically away from the medusa, precluding potential refiltration by the oral arms (Hamlet et al. Citation2011), apparently contradicting the idea that enhanced BPR at high temperatures is due to external feeding. A similar phenomenon has been observed in soft corals, where tentacle pulsation effectively hinders foraging, but increases photosynthesis by lowering oxygen concentrations in coral tissues and hence maintaining the affinity of RuBisCo to CO2, which would be lowered under high oxygen concentrations (Kremien et al. Citation2013). Symbiotic zooxanthellae harboured in Cassiopea jellyfish are known to produce high amounts of intracellular ROS at increased temperature, accumulating ROS such as superoxide radicals (O2−) and hydrogen peroxide (H2O2) (Lesser Citation1996; McGill and Pomory Citation2008; McGinty et al. Citation2012). McGill and Pomory (Citation2008) suggest that this could lead to oxidative stress both within the zooxanthellae and the host, given the membrane permeability of certain ROS. The hypothesis of Cassiopea jellyfish increasing its pulsation frequency not only to increase food intake, but in order to maintain high photosynthesis in its symbionts is supported by our observation that the photosynthetic system of the zooxanthellae remains unaffected by the temperature stress up to about 32°C, i.e. when significant decreases in BPR set in. Future research could corroborate this hypothesis, e.g. by monitoring dissolved oxygen concentrations in the microenvironment of Cassiopea medusae under oxidative stress.
In this study, we have defined 34°C as the upper limit of the thermal window for the adult C. andromeda jellyfish in our laboratory. Near this temperature limit, a decrease in bell pulsation coincides with a drastic reduction in photochemical efficiency (C and D). This particular strong decrease in Fv/Fm upon 34°C exposure in Symbiodiniaceae (both in hospite and isolated) is widely confirmed by the literature, albeit dependent on clade (Iglesias-Prieto et al. Citation1992; Fitt and Warner Citation1995; Hoegh-Guldberg et al. Citation1998; Robinson and Warner Citation2006). Though it has been demonstrated that Cassiopea can establish symbioses with non-native, more thermo-tolerant Symbiodiniaceae strains, such pairings appear more sensitive to bleaching events than wild types (Newkirk et al. Citation2020). Matching our results, adult C. xamanchana have been observed to bleach after 1 week of 34°C exposure (McGill and Pomory Citation2008), though it has been noted that lower night-time temperatures can extend this bleaching threshold by 4°C (Klein et al. Citation2019). To what extent jellyfish pulsation is contributing towards this threshold remains to be elucidated. Certainly however, the thermal window of C. andromeda appears relatively wide, which may allow the holobiont to further extend its range with ongoing temperature increases and invade new coastal environments. This may have important consequences to local ecosystems, including cycling of nutrients, trace elements or pollutants, given the conspicuous behaviour of pumping interstitial waters towards the surface which may simply be released or bioconcentrated. Indeed, various studies have demonstrated that gelatinous plankton and cnidarians can bioconcentrate substances, including toxic contaminants (Roméo et al. Citation1992; Fowler et al. Citation2004; Templeman and Kingsford Citation2010; Howe et al. Citation2014; Muñoz-Vera et al. Citation2015). Cassiopea spp. specifically were observed actively regulating intake of some metals (e.g. Li), while bioconcentrating other fine-scale pollutants such as Cu and Zn (Templeman and Kingsford Citation2010, Citation2012, Citation2015). This study indicates that Pb is only moderately taken up by the medusae, which is in contrast to Munoz-Vera et al. (Citation2015) but in line with Templeman and Kingsford (Citation2010), who have found Pb concentrations of wild Cassiopea always below the limit of detection and max. 10 µg kg−1 (wet weight). However, while no strong Pb bioconcentration was observed, the medusae were found capable of bioconcentrating significant amounts of Cd from the surrounding water. For Cd, C. andromeda showed BCFs of roughly 250 and 500 after 4 and 7 exposure days, respectively (), representing a linear increase through the 7 days of exposure. We therefore expect that Cd tissue concentrations, and therefore BCF, did not reach steady-state and would likely have continued to increase after this time. Pb concentrations, on the other hand, reached a plateau at or before 4 days of exposure, suggesting that the BCF is expected to remain more constant over longer exposure times. The calculated BCF of 38 ± 3 SD after 7 exposure days () is largely attributed to the high water content (mean 96%) of the organisms. Thus, C. andromeda appears capable of regulating Pb intake from its surrounding waters.
An important habitat for Cassiopea jellyfish is the Red Sea with the Gulf of Suez. The Gulf has a comparably high load of heavy metals. During winter 2003, mean concentrations of 2 μg l−1 Pb and 0.2 μg l−1 Cd were recorded (Hamed and Emara Citation2006). In the Mar Menor habitat in Spain, another ecosystem that is showing first signs of invasion by the species (Rubio Citation2017) that may expand with further temperature increase, free Pb water concentrations between 19 and 21 µg l−1 have been recorded (Auernheimer et al. Citation1996). Since C. andromeda actively pumps interstitial waters towards the sediment surface (Jantzen et al. Citation2010), also sedimentary heavy metal concentrations should be considered when assessing potential bioconcentration effects. In Red Sea sediments for example, concentrations between 42.15–18.54 μg g−1 for Pb and 3.98–1.98 μg g−1 for Cd have been measured (Hamed and Emara Citation2006).
While both Pb and Cd are highly toxic heavy metals with prominent industrial sources, they are not unambiguous tracers of anthropogenic pollution since both have natural sources in the marine environment as well. Studying gadolinium chelates (GDCA) and the associated rare earth element (REE) anomalies on the other hand has proven to be an effective tool to trace human-derived waste-water influxes (e.g. Hatje et al. Citation2016).
When calculating an average for the co-exposure experiments with Pb and Cd, we end up with an overall BCF for Tb of 25.7 ± 1.6 SD after 4, and 32.7 ± 4.6 SD after 7 days of exposure. These values slightly exceeded those of Gd with an overall calculated BCF of 16.9 ± 1.4 SD and 28.5 ± 0.9 SD after 4 and 7 days, respectively (see ). Although they differ after 4 days, these REE BCFs do not differ significantly after 7 exposure days. We suggest a kinetic effect such as stearic hindrance for explaining the slight difference in uptake, with Tb being taken up faster than the substantially larger Gd complex.
A GDCA uptake laboratory experiment with the freshwater bivalve Dreissena rostriformis bugensis (Perrat et al. Citation2017) allows for some degree of comparison with our experiments carried out on C. andromeda, although not ‘Gadovist’, but ‘Dotarem’, another common macrocyclic GDCA, was used in this case. After 7 days exposure to 1 µg l−1 chelated gadolinium, the bivalves’ digestive gland tissue contained 6 ± 5.8 SD ng g−1 Gd (DW), which is lower than the 17.04 ng g−1 (DW) in C. andromeda in our study. The bivalve concentrations increased to 77.8 ± 18 SD ng g−1 (DW) after 14 additional exposure days (Perrat et al. Citation2017). On the other hand, water content in C. andromeda is significantly higher than in the bivalve, which artificially inflates concentrations that are calculated based on dry weight.
It remains to be elucidated whether the organisms are capable of eliminating the metals from their body structures upon transfer to clear water – this could be investigated in future studies by adding a clearance phase to the experiment.
Conclusions and outlook
Temperature increase beyond 34°C is lethal to the Cassiopea andromeda jellyfish in this study, coming along with changes in bell pulsation rate and a strong reduction in zooxanthellae photochemical efficiency. Although stepwise 1°C or 2°C increases once per week lead to the same upper threshold of tolerance, a slower increase results in slower and less dramatic changes in BPR and photochemical efficiency. Hence, given the relatively wide thermal window of this tropical species, global temperature increase might be expected to drive further invasions of jellyfish of the genus Cassiopea into new coastal habitats. The results of this study further suggest that such an introduction of the holobiont into new environments might have significant impacts on local pollutant dynamics. We confirm the results of Templeman and Kingsford (Citation2010) showing that C. andromeda is able to bioconcentrate considerable amounts of Cd in their medusoid body. This is particularly relevant for coastal areas that receive runoff from agricultural or mining activity, since Cd pollution is often linked to zinc extraction and phosphate fertilizers (Loganathan and Hedley Citation1997; Howe et al. Citation2014). Bioconcentration of Pb has been shown to be much less severe. For some species, metal pollution toxicity has further been shown to increase with water temperature (Chapman et al. Citation2006). Temperature rise and increased metal contamination in near-shore habitats might thus act synergistically on C. andromeda.
Additionally, results indicate that jellyfish of the genus Cassiopea might not only be interesting in their role of an emerging model for cnidarian-zooxanthellae interactions, but also hold considerable interest as an environmental bioindicator species. We show that anthropogenic Gd complexes from MRI contrast media are taken up by the jellyfish in comparable amounts as uncomplexed, natural Tb. Although a small kinetic difference in the uptake pattern was observed, the data do not suggest a significant biological fractionation between the periodic system neighbours. We therefore conclude that C. andromeda could be a suitable bioindicator for tracing anthropogenic wastewater inputs in the natural environment.
Performing best at water temperatures between 26°C and 32°C, C. andromeda can be anticipated to expand its geographic range with climate change, as can already be observed with the ongoing invasion of the Mediterranean Sea (Schembri et al. Citation2010; Amor et al. Citation2016; Rubio Citation2017; Cillari et al. Citation2018; Maggio et al. Citation2019). Potential consequences for the environment could be the bioconcentration of waterborne pollutants and the mobilization of otherwise sediment-locked nutrients and material into the water column to become part of the food web (Jantzen et al. Citation2010; Stoner and Layman Citation2015). Especially in the case of a jellyfish population crash, the very rapid turnover of biomass can lead to high amounts of material being transferred to the pelagic and/or benthic food webs (Titelman et al. Citation2006; Yamamoto et al. Citation2008).
Acknowledgements
We thank Jule Mawick and Dr Katja Schmidt for their invaluable help in sample analysis at the geochemistry laboratory of Jacobs University. Thanks also to Nina Paul, Dr Karin Springer, Diane Enkelmann and Samir Aljbour for their support in experimental/measurement setup and animal handling in the ZMT MAREE. Thank you to Prof. Dr Andrea Koschinsky, for providing constructive feedback throughout the project.
Data availability
The authors confirm that the data supporting the findings of this study are available within the article, or are available from the corresponding author, P.B., upon reasonable request.
Disclosure statement
No potential conflict of interest was reported by the author(s).
Additional information
Funding
References
- Aljbour S, Zimmer M, Kunzmann A. 2017. Cellular respiration, oxygen consumption, and trade-offs of the jellyfish Cassiopea sp. in response to temperature change. Journal of Sea Research. 128:92–97. doi:10.1016/j.seares.2017.08.006
- Amor KOB, Rifi M, Ghanem R, Draief I, Zaouali J, Ben Souissi J. 2016. Update of alien fauna and new records from Tunisian marine waters. Mediterranean Marine Science. 17:124–143. doi:10.12681/mms.1371
- Arai MN. 1996. A functional biology of Scyphozoa. London: Chapman & Hall.
- Auernheimer C, Servando C, Jose AP. 1996. Lead pollution in bivalve shells: Mar Menor, Spain. Arch des Sci compte rendu des séances la Société. 49:87–98. doi:10.5169/seals-740414
- Avian M, Rottini Sandrini L, Stravisi F. 1991. The effect of seawater temperature on the swimming activity of Pelagia noctiluca (Forsskal). Bolletino di Zoologia. 58:135–143. doi:10.1080/11250009109355744
- Bau M, Dulski P. 1996. Anthropogenic origin of positive gadolinium anomalies in river waters. Earth and Planetary Science Letters. 143:245–255. doi:10.1016/0012-821X(96)00127-6
- Bau M, Knappe A, Dulski P. 2006. Anthropogenic gadolinium as a micropollutant in river waters in Pennsylvania and in Lake Erie, northeastern United States. Geochemistry. 66:143–152. doi:10.1016/j.chemer.2006.01.002
- Bhagooli R, Hidaka M. 2003. Comparison of stress susceptibility of in hospite and isolated zooxanthellae among five coral species. Journal of Experimental Marine Biology and Ecology. 291:181–197. doi:10.1016/S0022-0981(03)00121-7
- Chapman PM, Mcdonald BG, Kickham PE, Mckinnon S. 2006. Global geographic differences in marine metals toxicity. Marine Pollution Bulletin. 52:1081–1084. doi:10.1016/j.marpolbul.2006.05.004
- Cillari T, Andaloro E, Castriota L. 2018. First documented record of Cassiopea andromeda (Cnidaria: Scyphozoa) in Italian waters. Cahiers de Biologie Marine. 59:193–195. doi:10.21411/CBM.A.1037AA
- Colin SP, Costello JH. 2003. In situ swimming and feeding behavior of eight co-occurring hydromedusae. Marine Ecology Progress Series. 253:305–309. doi:10.3354/meps253305
- Díaz-Almeyda EM, Prada C, Ohdera AH, Moran H, Civitello DJ, Iglesias-Prieto R, Carlo TA, Lajeunesse TC, Medina M. 2017. Intraspecific and interspecific variation in thermotolerance and photoacclimation in Symbiodinium dinoflagellates. Proceedings of the Royal Society B: Biological Sciences. 284. doi:10.1098/rspb.2017.1767
- Dillon TM. 1977. Effects of acute changes in temperature and salinity on pulsation rates in ephyrae of the scyphozoan Aurelia aurita. Marine Biology. 42:31–35. doi:10.1007/BF00392011
- Epstein HE, Templeman MA, Kingsford MJ. 2016. Fine-scale detection of pollutants by a benthic marine jelly fish. Marine Pollution Bulletin. 107:340–346. doi:10.1016/j.marpolbul.2016.03.027
- Fitt WK, Warner ME. 1995. Bleaching patterns of four species of Caribbean Reef Corals. The Biological Bulletin. 189:298–307. doi:10.2307/1542147
- Fowler SW, Danis B, Fowler SW, Teyssié J, Cotret O, Danis B, Rouleau C. 2004. Applied radiotracer techniques for studying pollutant bioaccumulation in selected marine organisms (jellyfish, crabs and sea stars). Nukleonika. 49:97–100.
- Freeman CJ, Stoner EW, Easson CG, Matterson KO, Baker DM. 2017. Variation in δ13C and δ15N values suggests a coupling of host and symbiont metabolism in the Symbiodinium−Cassiopea mutualism. Marine Ecology Progress Series. 571:245–251. doi:10.3354/meps12138
- Gatz JA, Kennedy VS, Mihursky JA. 1973. Effects of temperature on activity and mortality of the scyphozoan medusa, Chrysaora quinquecirrha. Chesapeake Science. 14:171–180. doi:10.2307/1350603.
- Gohar HAF, Eisawy AM. 1960. The biology of Cassiopea andromeda (from the Red Sea). Publications of the Marine Biological Station, Ghardaqa. 11:48–190.
- Hamed MA, Emara AM. 2006. Marine molluscs as biomonitors for heavy metal levels in the Gulf of Suez, Red Sea. Journal of Marine Systems. 60:220–234. doi:10.1016/j.jmarsys.2005.09.007
- Hamlet CL, Miller LA. 2012. Feeding currents of the upside down jellyfish in the presence of background flow. Bulletin of Mathematical Biology. 74:2547–2569. doi:10.1007/s11538-012-9765-6
- Hamlet CL, Santhanakrishnan A, Miller LA. 2011. A numerical study of the effects of bell pulsation dynamics and oral arms on the exchange currents generated by the upside-down jellyfish Cassiopea xamachana. Journal of Experimental Biology. 214:1911–1921. doi:10.1242/jeb.052506
- Hatje V, Bruland KW, Flegal AR. 2016. Increases in anthropogenic gadolinium anomalies and rare earth element concentrations in San Francisco bay over a 20 year record. Environmental Science & Technology. 50:4159–4168. doi:10.1021/acs.est.5b04322
- Hoegh-Guldberg O, Jones RJ, Larkum AWD, Schreiber U. 1998. Temperature-induced bleaching of corals begins with impairment of the CO2 fixation mechanism in zooxanthellae. Plant, Cell and Environment. 21:1219–1230. doi:10.1046/j.1365-3040.1998.00345.x
- Hofmann DK, Kremer BP. 1981. Carbon metabolism and strobilation in Cassiopea andromedea (Cnidaria: Scyphozoa): significance of endosymbiotic dinoflagellates. Marine Biology. 65:25–33. doi:10.1007/BF00397064
- Holland BS, Dawson MN, Crow GL, Hofmann DK. 2004. Global phylogeography of Cassiopea (Scyphozoa: Rhizostomeae): molecular evidence for cryptic species and multiple invasions of the Hawaiian Islands. Marine Biology. 145:1119–1128. doi:10.1007/s00227-004-1409-4
- Howe PL, Reichelt-Brushett AJ, Clark MW. 2014. Investigating lethal and sublethal effects of the trace metals cadmium, cobalt, lead, nickel and zinc on the anemone Aiptasia pulchella, a cnidarian representative for ecotoxicology in tropical marine environments. Marine and Freshwater Research. 65:551–561. doi:10.1071/MF13195
- Iglesias-Prieto R, Matta JL, Robins WA, Trench RK. 1992. Photosynthetic response to elevated temperature in the symbiotic dinoflagellate Symbiodinium microadriaticum in culture. Proceedings of the National Academy of Sciences. 89:10302–10305. doi:10.1073/pnas.89.21.10302
- Jantzen C, Wild C, Rasheed M, El-zibdah M, Richter C. 2010. Enhanced pore-water nutrient fluxes by the upside-down jellyfish Cassiopea sp. in a Red Sea coral reef. Marine Ecology Progress Series. 411:117–125. doi:10.3354/meps08623
- Karim W, Nakaema S, Hidaka M. 2015. Temperature effects on the growth rates and photosynthetic activities of Symbiodinium cells. Journal of Marine Science and Engineering. 100:368–381. doi:10.3390/jmse3020368.
- Karunarathne KD, Liyanaarachchi SM, De Croos MDST. 2020. First record of upside-down jellyfish Cassiopea andromeda (Forskål, 1775) (Cnidaria: Scyphozoa: Rhizostomeae: Cassiopeidae) from Sri Lanka. Sri Lanka Journal of Aquatic Sciences. 25:57. doi:10.4038/sljas.v25i2.7577
- Klein SG, Pitt KA, Carroll AR. 2016. Reduced salinity increases susceptibility of zooxanthellate jellyfish to herbicide toxicity during a simulated rainfall event. Environmental Pollution. 209:79–86. doi:10.1016/j.envpol.2015.11.012
- Klein SG, Pitt KA, Lucas CH, Hung SH, Schmidt-Roac S, Aranda M, Duarte CM. 2019. Night-time temperature reprieves enhance the thermal tolerance of a symbiotic cnidarian. Frontiers in Marine Science. 6:1–16. doi:10.3389/fmars.2019.00001
- Krämer WE, Caamaño-Ricken I, Richter C, Bischof K. 2012. Dynamic regulation of photoprotection determines thermal tolerance of two phylotypes of Symbiodinium clade a at two photon fluence rates. Photochemistry and Photobiology. 88:398–413. doi:10.1111/j.1751-1097.2011.01048.x
- Kremien M, Shavit U, Mass T, Genin A. 2013. Benefit of pulsation in soft corals. Proceedings of the National Academy of Sciences. 110:8978–8983. doi:10.1073/pnas.1301826110
- Kulaksiz S, Bau M. 2011b. Anthropogenic gadolinium as a microcontaminant in tap water used as drinking water in urban areas and megacities. Applied Geochemistry. 26:1877–1885. doi:10.1016/j.apgeochem.2011.06.011
- Larson RJ. 1997. Feeding behaviour of Caribbean scyhomedusae: Cassiopea frondosa (Pallas) and Cassiopea xamachana. Studies on the Natural History of the Caribbean Region. 73.
- Lawrence MG, Ort C, Keller J. 2009. Detection of anthropogenic gadolinium in treated wastewater in South East Queensland, Australia. Water Research. 43:3534–3540. doi:10.1016/j.watres.2009.04.033
- Lesser MP. 1996. Elevated temperatures and ultraviolet radiation cause oxidative stress and inhibit photosynthesis in symbiotic dinoflagellates. Limnology and Oceanography. 41:271–283. doi:10.4319/lo.1996.41.2.0271
- Loganathan P, Hedley MJ. 1997. Downward movement of cadmium and phosphorus from phosphatic fertilisers in a pasture soil in New Zealand. Environmental Pollution. 95:319–324. doi:10.1016/S0269-7491(96)00142-X
- Lyndby NH, Rädecker N, Bessette S, Søgaard Jensen LH, Escrig S, Trampe E, Kühl M, Meibom A. 2020. Amoebocytes facilitate efficient carbon and nitrogen assimilation in the Cassiopea-Symbiodiniaceae symbiosis. Proceedings of the Royal Society B: Biological Sciences. 20202393. doi:10.1098/rspb.2020.2393
- Maggio T, Allegra A, Bosch-belmar M, Cillari T, Castriota L, Cuttitta A, Falautano M, Milisenda G, Nicosia A. 2019. Molecular identity of the non-indigenous Cassiopea sp. from Palermo Harbour (central Mediterranean Sea).
- Mammone M, Ferrier-Pages C, Lavorano S, Rizzo L, Piraino S, Rossi S. 2021. High photosynthetic plasticity may reinforce invasiveness of upside-down zooxanthellate jellyfish in Mediterranean coastal waters. PLoS One. 16:1–17. doi:10.1371/journal.pone.0248814
- Mayer AG. 1914. The effects of temperature on tropical marine animals. Pap Tortugas Lab 3.
- McGill CJ, Pomory CM. 2008. Effects of bleaching and nutrient supplementation on wet weight in the jellyfish Cassiopea xamachana (Bigelow) (Cnidaria: Scyphozoa). Marine and Freshwater Behaviour and Physiology. 41:179–189. doi:10.1080/10236240802369899
- McGinty ES, Pieczonka J, Mydlarz LD. 2012. Variations in reactive oxygen release and antioxidant activity in multiple Symbiodinium types in response to elevated temperature. Microbial Ecology. 64:1000–1007. doi:10.1007/s00248-012-0085-z
- Merschel G, Bau M, Baldewein L, Dantas EL, Walde D, Bühn B. 2015. Tracing and tracking wastewater-derived substances in freshwater lakes and reservoirs: anthropogenic gadolinium and geogenic REEs in Lake Paranoa. Comptes Rendus Geoscience. 347:284–293. doi:10.1016/j.crte.2015.01.004
- Möller P, Dulski P, Bau M, Knappe A, Pekdeger A, Mo P, Jarmersted CS. 2000. Anthropogenic gadolinium as a conservative tracer in hydrology. Journal of Geochemical Exploration. 69-70:409–414. doi:10.1016/S0375-6742(00)00083-2
- Morandini AC, Stampar SN, Maronna MM, Da Silveira FL. 2017. All non-indigenous species were introduced recently? The case study of Cassiopea (Cnidaria: Scyphozoa) in Brazilian waters. Journal of the Marine Biological Association of the United Kingdom. 97:321–328. doi:10.1017/S0025315416000400
- Muñoz-Vera A, García G, García-Sánchez A. 2015. Metal bioaccumulation pattern by Cotylorhiza tuberculata (Cnidaria, Scyphozoa) in the Mar Menor coastal lagoon (SE Spain). Environmental Science and Pollution Research. 22:19157–19169. doi:10.1007/s11356-015-5119-x
- Negri AP, Flores F, Röthig T, Uthicke S. 2011. Herbicides increase the vulnerability of corals to rising sea surface temperature. Limnology and Oceanography. 56:471–485. doi:10.4319/lo.2011.56.2.0471
- Negri AP, Hoogenboom MO. 2011. Water contamination reduces the tolerance of coral larvae to thermal stress. PLoS One. 6:e19703. doi:10.1371/journal.pone.0019703
- Newkirk CR, Frazer TK, Martindale MQ, Schnitzler CE. 2020. Adaptation to bleaching: are Thermotolerant Symbiodiniaceae strains more successful than other strains under elevated temperatures in a model symbiotic cnidarian? Frontiers in Microbiology. 11:1–12. doi:10.3389/fmicb.2020.00822
- Niggl W, Wild C. 2009. Spatial distribution of the upside-down jellyfish Cassiopea sp. within fringing coral reef environments of the Northern Red Sea: implications for its life cycle. Helgoland Marine Research. 64:281–287. doi:10.1007/s10152-009-0181-8
- Nozaki Y, Lerche D, Alibo DS, Tsutsumi M. 2000. Dissolved indium and rare earth elements in three Japanese rivers and Tokyo Bay: Evidence for anthropogenic Gd and In. Geochimica et Cosmochimica Acta. 64:3975–3982. doi:10.1016/S0016-7037(00)00472-5
- Ohdera AH, Abrams MJ, Ames CL, Baker DM, Suescún-Bolívar LP, Collins AG, Freeman CJ, Gamero-Mora E, Goulet TL, Hofmann DK, et al. 2018. Upside-down but headed in the right direction: review of the highly versatile Cassiopea xamachana system. Frontiers in Ecology and Evolution. 6:1–15. doi:10.3389/fevo.2018.00035
- Özgür E, Öztürk B. 2008. Aa population of the alien jellyfish, Cassiopea andromeda (Forsskål, 1775) (Cnidaria: Scyphozoa: Rhizostomea) in the Ölüdeniz Lagoon, Turkey. Aquatic Invasions. 3:423–428. doi:10.3391/ai.2008.3.4.8
- Perrat E, Parant M, Py J-S, Rosin C, Cossu-Leguille C. 2017. Bioaccumulation of gadolinium in freshwater bivalves. Environmental Science and Pollution Research. 24:12405–12415. doi:10.1007/s11356-017-8869-9
- Rädecker N, Pogoreutz C, Wild C, Voolstra CR. 2017. Stimulated respiration and net photosynthesis in Cassiopeia sp. during glucose enrichment suggests in hospite CO2 limitation of algal endosymbionts. Frontiers in Marine Science. 4:2–5. doi:10.3389/fmars.2017.00267
- Robinson JD, Warner ME. 2006. Differential impacts of photoacclimation and thermal stress on the photobiology of four different phylotopes of Symbiodinium. Journal of Phycology. 42:568–579. doi:10.1111/j.1529-8817.2006.00232.x
- Roméo M, Gnassia-Barelli M, Carré C. 1992. Importance of gelatinous plankton organisms in storage and transfer of trace metals in the northwestern Mediterranean. Marine Ecology Progress Series. 82:267–274. doi:10.3354/meps082267
- Rowen DJ, Templeman MA, Kingsford MJ. 2017. Chemosphere herbicide effects on the growth and photosynthetic efficiency of Cassiopea maremetens. Chemosphere. 182:143–148. doi:10.1016/j.chemosphere.2017.05.001
- Rubio M. 2017. Una medusa tropical, nueva amenaza para la biodiversidad del mar Menor [WWW Document]. [accessed 14 March 2020]. https://elclickverde.com/reportajes/una-medusa-tropical-nueva-amenaza-para-la-biodiversidad-del-mar-menor.
- Santhanakrishnan A, Dollinger M, Hamlet CL, Colin SP, Miller LA. 2012. Flow structure and transport characteristics of feeding and exchange currents generated by upside-down Cassiopea jellyfish. Journal of Experimental Biology. 215:2369–2381. doi:10.1242/jeb.053744
- Schembri PJ, Deidun A, Vella PJ. 2010. First record of Cassiopea andromeda (Scyphozoa: Rhizostomeae: Cassiopeidae) from the central Mediterranean Sea. Marine Biodiversity Records. 3:1–2. doi:10.1017/S1755267209990625
- Smith DJ, Suggett DJ, Baker NR. 2005. Is photoinhibition of zooxanthellae photosynthesis the primary cause of thermal bleaching in corals? Global Change Biology. 11:1–11. doi:10.1111/j.1529-8817.2003.00895.x
- Stampar SN, Gamero-mora E, Maronna MM, Fritscher JM, Oliveira BSP, Sampaio CLS, Morandini AC. 2020. The puzzling occurrence of the upside-down jellyfish Cassiopea (Cnidaria: Scyphozoa) along the Brazilian coast: a result of several invasion events? Sociedade Brasileira de Zoologia. 4689:1–10.
- Stoner EW, Layman CA. 2015. Bristle worms attack: benthic jellyfish are not trophic dead ends. Frontiers in Ecology and the Environment. 13:226–227. doi:10.1890/1540-9295-13.4.226
- Stoner EW, Layman CA, Yeager LA, Hassett HM. 2011. Effects of anthropogenic disturbance on the abundance and size of epibenthic jellyfish Cassiopea spp. Marine Pollution Bulletin. 62:1109–1114. doi:10.1016/j.marpolbul.2011.03.023
- Tchernov D, Gorbunov MY, de Vargas C, Narayan Yadav S, Milligan AJ, Häggblom M, Falkowski PG. 2004. Membrane lipids of symbiotic algae are diagnostic of sensitivity to thermal bleaching in corals. Proceedings of the National Academy of Sciences. 101:13531–13535. doi:10.1073/pnas.0402907101
- Templeman MA, Kingsford M. 2015. Predicting aqueous copper and zinc accumulation in the upside-down jellyfish Cassiopea maremetens through the use of biokinetic models. Environmental Monitoring and Assessment. 187:416. doi:10.1007/s10661-015-4657-5
- Templeman MA, Kingsford MJ. 2010. Trace element accumulation in Cassiopea sp. (Scyphozoa) from urban marine environments in Australia. Marine Environmental Research. 69:63–72. doi:10.1016/j.marenvres.2009.08.001
- Templeman MA, Kingsford MJ. 2012. Variation in soft tissue chemistry among scyphozoan and cubozoan jellyfishes from the Great Barrier Reef, Australia. Hydrobiologia. 690:279–290. doi:10.1007/s10750-012-1051-y
- Thé J, Barroso HS, Mammone M, Viana M, Batista Melo CS, Mies M, Banha TNS, Morandini AC, Rossi S, Soares MO. 2020. Aquaculture facilities promote populational stability throughout seasons and increase medusae size for the invasive jellyfish Cassiopea andromeda. Marine Environmental Research. 105161. doi:10.1016/j.marenvres.2020.105161
- Thielmann DT. 2011. Novel functionalized rare earth metal clusters and polymers. Göttingen: Karsruhe Institut für Technologie KIT.
- Titelman J, Riemann L, Sørnes TA, Nilsen T, Griekspoor P, Båmstedt U. 2006. Turnover of dead jellyfish: stimulation and retardation of microbial activity. Marine Ecology Progress Series. 325:43–58. doi:10.3354/meps325043
- Trapasso G, Chiesa S, Freitas R, Pereira E. 2021. What do we know about the ecotoxicological implications of the rare earth element gadolinium in aquatic ecosystems? Science of The Total Environment. 781:146273. doi:10.1016/j.scitotenv.2021.146273
- Verde EA, McCloskey LR. 1998. Production, respiration, and photophysiology of the mangrove jellyfish Cassiopea xamachana symbiotic with zooxanthellae: effect of jellyfish size and season. Marine Ecology Progress Series. 168:147–162. doi:10.3354/meps168147
- Verplanck PL, Taylor HE, Nordstrom DK, Barber LB. 2005. Aqueous stability of gadolinium in surface waters receiving sewage treatment plant effluent Boulder Creek, Colorado. Environmental Science & Technology. 39:6923–6929. doi:10.1021/es048456u
- Vodenichar JS. 1995. Ecophysiological physiology of the Scyphozoan Cassiopea xamachana. Athens: University of Georgia.
- Welsh DT, Dunn RJK, Meziane T. 2009. Oxygen and nutrient dynamics of the upside down jellyfish (Cassiopea sp.) and its influence on benthic nutrient exchanges and primary production. Hydrobiologia. 635:351–362. doi:10.1007/s10750-009-9928-0
- Yamamoto J, Hirose M, Ohtani T, Sugimoto K, Hirase K, Shimamoto N, Shimura T, Honda N, Fujimori Y, Mukai T. 2008. Transportation of organic matter to the sea floor by carrion falls of the giant jellyfish Nemopilema nomurai in the Sea of Japan. Marine Biology. 153:311–317. doi:10.1007/s00227-007-0807-9
- Zarnoch CB, Hossain N, Fusco E, Alldred M, Hoellein TJ, Perdikaris S. 2020. Size and density of upside-down jellyfish, Cassiopea sp., and their impact on benthic fluxes in a Caribbean lagoon. Marine Environmental Research. 154:104845. doi:10.1016/j.marenvres.2019.104845