Abstract
The effects of four different ante-mortem stressors (exercise, emersion, starvation and a patent infection with the parasite Hematodinium sp.) on post-mortem processes have been investigated in the abdominal muscle of Norway lobster Nephrops norvegicus by measuring changes in the pH, the levels of glycogen, l-lactate, arginine phosphate, ATP, ADP, AMP, IMP, HxR, Hx and the adenylate energy charge (AEC) over a time course of 24 h with samples being taken at 0, 3, 6, 12 and 24 h. The acute stresses of intense exercise and 2 h emersion resulted in a premature onset of anaerobic glycolysis, leading both to an enhanced glycogen depletion rate and an early accumulation of l-lactate. The chronic stressors, starvation and parasite infection, resulted in a complete ante-mortem depletion of muscle glycogen and consequently the failure of post-mortem glycolytic fermentation. Post-mortem pH and ATP inter-conversion were significantly altered in chronically stressed animals. Ante-mortem, a rapid, almost complete depletion of arginine phosphate was observed in all stress groups. The AEC was altered significantly by all stresses, indicating a strong energy demand. The findings suggest that ante-mortem stressors strongly influence the post-mortem biochemical processes. The laboratory-based results are compared to ‘field’ data and effects on post-harvest product quality are discussed.
Published in collaboration with the University of Bergen and the Institute of Marine Research, Norway, and the Marine Biological Laboratory, University of Copenhagen, Denmark
Introduction
It has been shown in many studies on the muscle tissues of fish and mammals, which are used as a food source, that ante-mortem stress strongly influences the course of post-mortem events. Not only is the post-mortem biochemistry of these muscles changed, but consequently the overall quality, palatability and shelf life (use by date) of the meat is altered (Hendricks Citation1965; Fraser et al. Citation1966; Lee et al. Citation1976; Lewis et al. Citation1981; Sikorski et al. Citation1990; Lowe et al. Citation1993; Sigholt et al. Citation1997; Abdalla et al. Citation1999; Diouf & Rioux Citation1999). There are numerous studies on spoilage and storage effects in the muscles of crustaceans (Sidhu et al. Citation1974; Stroud et al. Citation1982; Ashie et al. Citation1996; Chinivasagam et al. Citation1996; Shimada et al. Citation2000; Mendes et al. Citation2001; Aubourg et al. Citation2007), but there is very little knowledge of the way in which ante-mortem stressors change the post-mortem biochemistry, and hence quality of related shellfisheries products.
The abdominal muscles of decapod crustaceans such as the Norway lobster Nephrops norvegicus (Linnaeus, 1758) that perform rapid tail-flip responses, e.g. during escape swimming, comprise mainly fast-type fibres that are biochemically equipped for rapid burst activity (Neil et al. Citation1993). These fast-type crustacean muscles are generally similar in both structure and metabolic properties to vertebrate fast-type muscles (Hoyle Citation1969; Beis & Newsholme Citation1975; Hooper & Thuma Citation2005; Gornik et al. 2008). To meet the energy demands of tail flipping and in a variety of other physiological circumstances, such fast-type muscles utilize anaerobic energy pathways (mainly glycolytic fermentation) (Ellington Citation1983). Reduced ATP production, l-lactate accumulation and glycogen depletion are the consequence of this fermentative glycolysis in both crustaceans (Paterson Citation1993; Hervant et al. Citation1999) and vertebrates (Bayliss Citation1995; Delbarre-Ladrat et al. Citation2006; Neath et al. Citation2007; Scheffler & Gerrard Citation2007).
The metabolic and respiratory responses of crustaceans to stress have been reported in many studies. In the prawns Palaemon elegans Rathke, 1837 and P. serratus (Pennant, 1777), for example, tissue l-lactate levels increase rapidly during acute anoxia, indicating anaerobic glycolysis (Taylor & Spicer Citation1987) and similar responses occur in the shore crab Carcinus maenas (Linnaeus, 1758) during environmental recovery from aerial exposure (Hill et al. Citation1991). Exercise has also been shown to induce anaerobic energy generation (e.g. Herreid & Full Citation1986). Morris & Adamczewska (Citation2002) showed that brief exercise in the terrestrial air-breathing crab Gecarcoidea natalis (Pocock, 1888) depletes arginine phosphate stores, consumes glycogen and results in the accumulation of l-lactate within the walking leg muscles. The tail-flip escape swimming of the spiny lobster Jasus edwardsii (Hutton, 1875) induces extensive anaerobic glycolysis in the abdominal muscles (Speed et al. Citation2001). In Nephrops norvegicus it was shown that not only anoxia (through aerial exposure) but also the hypoxic conditions (10–30% O2 saturation) in the bottom water of certain fishing grounds cause significant glycogen depletion, thus indicating anaerobic fermentation (Baden et al. Citation1994). Interestingly, some animals like Saduria entomon (Linnaeus, 1758) can also maintain their oxygen demand (aerobic ATP production) for some time by adaptation of ventilation and haemolymph circulation frequencies, before ultimately switching to anaerobic pathways (Hagerman & Szaniawska Citation1988).
During starvation, crustaceans, like all living organisms, must use body reserves (e.g. lipids, proteins and/or glycogen) to supply energy to maintain metabolism (Hervant et al. Citation1999). The relative importance of these metabolic reserves and their order of utilization vary with species (Hervant et al. Citation1999; Sanchez-Paz et al. Citation2006). However, in most species, glycogen (as the main carbohydrate source) is generally used first, then lipids and finally proteins (Hervant et al. Citation1999; Sanchez-Paz et al. Citation2006). During a 28-day starvation study in the shrimp Marsupenaeus (as Penaeus) japonicus (Bate, 1888) the glycogen stores were rapidly depleted, presumably being converted to glucose and used as an energy source (Cuzon et al. Citation1980). Starved individuals are thus deprived of glycogen reserves.
An infection with the parasitic dinoflagellates Hematodinium sp. results in dramatic pathological alterations to the organs, tissues and haemolymph of its decapod crustacean hosts. Physiological and biochemical disruptions to the muscles and other organs substantially alter the metabolism of infected hosts (Stentiford & Shields Citation2005). It was demonstrated by Stentiford et al. (Citation2001a; Citationb) that the presence of the Hematodinium parasite represents a major stressor in N. norvegicus. During patent infection, the concentration of glucose in the haemolymph was reduced significantly and significantly lower levels of hepatopancreatic glycogen accompanied this change; however, muscle glycogen was not investigated. As a consequence of the systemic infection, the normal feedback loops that control the release of CHH (crustacean hyperglycaemic hormone, a crustacean glucagon analog) were disrupted and plasma concentrations of CHH increased with the severity of infection. It was concluded that the parasite placed a heavy metabolic load on the host lobster.
We have recently shown that the sequence and characteristics of post-mortem changes in N. norvegicus are comparable to post-mortem changes known for most vertebrates (Gornik et al. Citation2008). The tissue reacts with a rapid response to meet ATP demands: the short-term energy buffer arginine phosphate is rapidly depleted, and anaerobic glycolysis results in glycogen depletion and l-lactate accumulation. In this way, ATP levels are maintained for an unusually long time post-mortem (~12 h at 10°C) before they eventually fall. This also has a major impact on rigor mortis development in the species (Gornik et al. Citation2009). It is also known that in response to trawling there is accumulation of l-lactate in the haemolymph and depletion of glycogen in the muscles and hepatopancreas of N. norvegicus (Ridgway et al. Citation2006a), and conversion of ATP to AMP and IMP (Mendes et al. Citation2001). Albalat et al. (Citation2009) showed that in rested and creel-caught animals the main nucleotide was ATP, while in trawled animals the main nucleotide was found to be AMP. Further, the AEC was lower in trawled animals compared with creel-caught animals, while trawling time did not significantly affect AEC values further. l-Lactate levels together with muscle pH indicated that trawled animals, even at the shortest time tested, were utilizing glycolytic fermentation (anaerobic metabolism).
It remains to be determined how the different stressors that may occur in vivo during the capture (trawling, creeling) and handling stages affect the post-mortem events in N. norvegicus. Furthermore, for commercial crustacean fisheries (such as the N. norvegicus fisheries), knowledge of how ante-mortem stressors affect the post-mortem processes and eventually quality is important in relation to the capture, handling and holding processes employed. Thus, during trawling extreme exercise is induced in the capture phase (Newland et al. Citation1992; Albalat et al. Citation2009) and handling on deck after trawling or after trapping in creels involves periods of emersion in the air (Schmitt & Uglow Citation1997; Harris & Andrews Citation2005a; Citationb; Ridgway et al. Citation2006b). Holding in pounds or cages may lead to prolonged starvation (Schirf et al. Citation1987), and seasonal effects causing changes in climatic factors such as water or air temperature (Giomi et al. Citation2008) and biological factors such as the moulting state, or infection (Stentiford et al. Citation2001a; Chang Citation2001) may also apply. Gaining an understanding of the relative severity of these stressors is important for recommending the most effective way to reduce physiological threats to the survival of these animals during holding and live transportation, and also helping to extend the shelf-life (use by date) of whole animals or tails, and improve their overall quality.
In accordance with ‘field’ data, we expect that stressors such as exercise and emersion will result in an enhanced glycolysis as seen after trawling and to some extent after creeling (Ridgeway et al. 2006a,b; Albalat et al. Citation2009). Starvation and the systematic infection with Hematodinium sp., on the other hand, might not change the post-mortem response of N. norvegicus in the short-term, although long-term changes are expected as a result of these chronic stresses (e.g. lack of glycogen). The accumulation of by-products of glycolysis (such as l-lactate) and changes in the inter-conversion rate of ATP and related nucleotides will have effects on the post-harvest quality. l-Lactate, for example, reduces muscle pH and it is known for fish and red meat that such a pH change affects post-harvest quality and palatability (Sikorski et al. 1990; Huss Citation1995). The accumulation of IMP (an intermediate metabolite during ATP inter-conversion), on the other hand, is important for fish quality since it is a main component of the taste of fresh fish. Premature IMP accumulation, e.g. induced by ante-mortem and post-capture stressors, can lead to loss of the ‘fresh taste’ when the product is finally sold post-harvest. HxR and especially Hx are known to cause bitter off-flavours (Huss Citation1995).
This study was conducted to elucidate which changes take place in post-mortem muscle when different ante-mortem stressors such as exercise, emersion, starvation and a patent infection with the blood-borne parasite Hematodinium sp. have been applied. Changes have been assessed not only for their immediate effects, but also for long-term changes they might induce in the muscle tissue. From the results conclusions are drawn and assumptions are made as to how the observed changes could affect post-catch and post-process quality.
Materials and methods
Capture and transport of Nephrops norvegicus
Nephrops norvegicus were caught in the Clyde Sea Area (55.41°N, 4.56°W, Scotland, UK) between February and May of 2007 using baited traps (creels). Only healthy male individuals in the intermoult stage, as determined following the method of Aiken (Citation1980), and within a size range of 35–55 mm carapace length (distance from the posterior margin of the eye socket to the midline of the posterior carapace edge) were used. On board the fishing vessel the animals were kept in flow-through seawater holding tanks. Once landed, the animals were transferred to tube-sets (vertically subdivided containers), immersed in fresh seawater (without aeration) and transported to the University of Glasgow (1–1.5 h transport).
Aquarium maintenance
Animals were kept in separation at a temperature of 8°C and a salinity of 33–35 (Practical Salinity Scale) in holding tanks (6 animals per tank, approx. 26 litres per animal, tank dimensions: W 40 cm ×H 40 cm×L 100 cm), which were supplied by a closed circuit flow-through seawater system, equipped with a coarse sand filter, an active charcoal filter and a bio-filter unit. The lobsters were fed twice a week with ground fish paste.
Experimental design
Lobsters were kept for 4 weeks prior to the experimental procedure and only animals that presented themselves as healthy (lively and feeding) over this period were selected for experimentation. Great care was taken not to stress the lobsters prior to the actual experiment. Groups of animals were subjected to the different stressors (see below), or to none (control group of rested animals). Readings of muscle pH were carried out on three replicates (individual abdomen). Tissue sampling was carried out on nine replicates for exercised, emersed and parasitized animals and three replicates for starved animals.
Stressors were: (a) exercise – animals were exercised prior to sacrifice by inducing ~100–150 tail flips as described in Newland et al. (Citation1988; Citation1992) within a period of 5 min; (b) emersion – animals were emersed in air for 2 h at ~8°C prior to culling using a method described in Spicer et al. (Citation1990); (c) starvation – lobsters were starved for 4 weeks prior to experimentation; (d) Hematodinium sp. infection – patently infected animals were selected from the trawled catch on board the vessel by using a rapid method based on body colour and general appearance, as described by Stentiford et al. (Citation2001b) and used by Briggs & McAliskey (Citation2002). Moribund animals were discarded.
Immediately after application of the stress, animals were sacrificed by separating the carapace from the abdomen. An initial tissue sample (approx. 1–2 g) was taken from the abdominal muscle, frozen in liquid nitrogen and subsequently stored at –80°C. The remainder of the abdomen was incubated at 10°C in a tabletop incubator (ECHOtherm® IN30, Torrey Pines Scientific) in a humid-chamber and sampled periodically at 3, 6, 12 and 24 h post-mortem in much the same way. Simultaneously, a continuous pH reading was taken in situ from a different abdomen to record the change in the extracellular muscle pH during incubation at 10°C for 24 h.
Post-mortem extracellular muscle pH
The extracellular pH was measured using a flexible micro pH electrode (Model PEEK 9080-008, SENTRON, Roden, The Netherlands). To measure, the pointed tip of the electrode was inserted into the abdominal muscle. The pH probe was connected to a SENTRON pH meter (Model Titan), which in turn was connected to a 4-channel data acquisition system (PowerLab 4/26, ADInstruments, Oxfordshire, UK) via a single-channel, non-isolated bridge amplifier (Model ML221, ADInstruments). The readings were recorded on hard disk using the Chart5 software system (ADInstruments) on a WinXP operated machine.
Sample extraction for biochemical analysis
Approximately 1 g of muscle was taken from each sample, and the exact weight was recorded. The tissue was homogenized on ice with a 5× volume (w/v) of 0.6 M perchloric acid (PCA) using an Ultra Turrax® T25 (IKA) tabletop homogenizer. Half a millilitre of the homogenate was set aside and frozen at –20°C for subsequent glycogen analysis. The remainder of the homogenate was centrifuged for 10 min at 4°C and 16,000 g in a tabletop centrifuge (Heraeus, Biofuge fresco). Afterwards, a 0.5 ml fraction of the supernatant was removed and frozen at –20°C for l-lactate determination. The remaining supernatant was processed for HPLC analysis.
Glycogen determination
To measure the glycogen content in the abdominal muscle samples a two-step method was used as described by Bergmeyer et al. (Citation1985). In the first step, the glycogen in the unfiltered homogenate was hydrolysed by the action of amyloglucosidase. The amount of liberated glucose was measured enzymatically in a second step. Background glucose levels were measured before amyloglucosidase treatment and subtracted from the final glucose reading. A standard curve was prepared using d-glucose at concentrations of 0.5–10.0 mM.
l-lactate determination
The concentration of l-lactate was determined enzymatically using a spectrometric method described by Bergmeyer & Bernt (Citation1985) further modified by Hill et al. (Citation1991). The concentration of l-lactate in the sample was calculated by extrapolation from the ▵A of a standard. A standard curve was prepared using l-lactate at concentrations ranging from 0.5 to 10.0 mM.
Sample extraction for nucleotide and arginine phosphate analysis
Three millilitres of the supernatant of muscle homogenate was transferred to a glass vial and the pH was adjusted to 6.5–6.8 with 1.0 M KOH. The sample was then incubated for 30 min on ice and filtered with a 0.22 µm syringe filter (Sartorius, 16534k) into a new tube. An equal volume of phosphate buffer (0.06 M K2HPO4, 0.04 M KH2PO4, pH 7.0) was added to make up a 6 ml dilution. A sub-sample was finally carried over into a lightproof HPLC vial and stored at –20°C until HPLC analysis.
Nucleotide HPLC
To determine the individual nucleotide concentrations and the AEC values, ATP, ADP, AMP, IMP, HxR and Hx were analysed by HPLC following a protocol modified from Ryder (Citation1985). A SP8800 ternary HPLC pump was used and coupled to a PDA detector (Thermo Finnigan) set to monitor at 254 nm. The system was operated and controlled using the Xcalibur® systems software (Thermo Finnigan). Peaks were analysed using the same software package. Separations were carried out using a reverse-phase C18 SYNERGY MAX-RP 80 A column (250×4.60 mm, with an internal particle diameter of 4 mm, Phenomenex, Torrance, CA, USA) fitted with an C18 SecurityGuard cartridge (4×3.0 mm, I.D., Phenomenex) at 40°C. The mobile phase was composed of: solvent A (0.04 M KH2PO4, 0.06 M K2HPO4, pH 7.0) and solvent B (methanol (MeOH)). Standard curves were prepared from adenosine 5'-triphosphate (ATP), adenosine 5'-diphosphate (ADP), adenosine 5'-monophosphate (AMP), inosine 5'-monophosphate (IMP), inosine (HxR) and hypoxanthine (Hx) in concentrations ranging from 0.1 to 1.0 mM (all Sigma). The concentrations of different products of ATP breakdown were calculated by extrapolation from the ▵A of the standard.
Arginine phosphate HPLC
Arginine phosphate was determined following the method of Viant et al. (Citation2001). An UltiMaster 3000 LCi Series HPLC system (Dionex Corporation, Sunnyvale, USA) was used. The system was fitted with a low-pressure gradient quaternary analytical pump, an ACC-3000 auto-sampler column compartment and a variable wavelength detector set to monitor at 205 nm. The system was operated and controlled using the Chromeleon® chromatography management systems software (Dionex). Peaks were analysed using the same software package. Separation of arginine phosphate was achieved by using a reversed-phase SphereClone NH2 column (250×34.6 mm I.D., 5 mm particle diameter; Phenomenex, Torrance, CA, USA) fitted with an NH2 SecurityGuard cartridge (4×3.0 mm I.D.; Phenomenex), at room temperature. The isocratic mobile phase was composed of KH2PO4 buffer (20 mM, pH 2.6) and acetonitrile in a proportion of 72 to 28 (v/v) and was used at a flow rate of 1 ml min–1. The total analysis time per sample was 20 min. A 10 mM arginine phosphate standard (Sigma) stock solution was prepared in KH2PO4 buffer (20 mM, pH 7.0) and diluted in the same buffer to concentrations between 0.025 and 5 mM arginine phosphate. A calibration curve was generated by injecting 10 µl of each standard (in triplicate), and the result was subjected to linear regression analysis. The concentrations of arginine phosphate were calculated by extrapolation from the standard.
AEC calculation
The AEC was calculated from previously determined nucleotide concentrations (ATP, ADP, AMP) according to Atkinson (Citation1965) using the following formula:
Statistical analysis
After the homogeneity of variance of a data set was assured using the Levene statistic (p>0.05), multivariate F statistics (ANOVA) were used to test the hypothesis and a Tukey's t-test was used to determine statistical differences between sample means. The software package SPSS v.15.0 was used for statistical analysis. Regressions and curve fittings were evaluated using the 'Regression Wizard' function in SigmaPlot 9.0 (SYSTAT Inc.).
Results
Arginine phosphate
When compared with values from rested animals, the concentration of arginine phosphate in the abdominal muscle of animals subjected to each of the applied stressors was significantly lower () with the emersed animals showing the most pronounced depletion. By 3 h post-mortem, arginine phosphate was no longer detectable in significant amounts in any of the stressed muscle tissues while the metabolite remained detectable for 12 h in muscle of rested animals ().
Figure 1. Post-mortem arginine phosphate depletion in the abdominal muscle of rested Nephrops norvegicus muscle and after ante-mortem exercise, emersion, starvation and a patent parasite infection with Hematodinium sp. Each point is the mean±SEM of nine samples in rested, exercised, infected and emersed animals and the mean±SEM of three samples in starved animals; • = rested; ▾= exercised; ○ = emersed; ▵ = parasitized; ▪ = starved; means of the different treatments are compared at a given sampling time. Different letters indicate statistical differences between means, p<0.05.
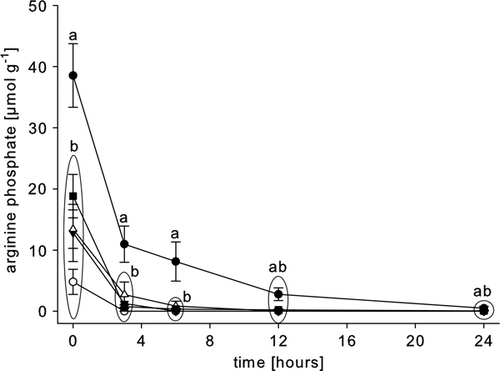
Table I. The in vivo metabolite concetrations in Nephrops norvegicus abdominal muscle immediately after the application of various stresses.
Glycogen
After a 5-min period of intense exercise (>100 tail flips) the glycogen concentration in the abdominal muscle was found not to be significantly different from the value in rested animals (, ), although a trend towards a premature depletion was indicated. Over the 24 h period, glycogen was depleted from the abdominal muscle of both the rested and exercised animals at the same rate post-mortem (). After hypoxia (2 h emersion), the muscle glycogen concentration () was found to be similar to that of the rested and exercised group, and over the post-mortem period it was depleted at a similar rate (). In starved animals, in contrast to the above results, only minimal amounts of glycogen () were found in the abdominal muscle, and no further significant depletion was observed post-mortem (). The same was found in animals carrying Hematodinium sp. parasites (, ).
Figure 2. Post-mortem glycogen depletion (as glycosyl units) in the abdominal muscle of rested Nephrops norvegicus muscle and after ante-mortem exercise, emersion, starvation and a patent parasite infection with Hematodinium sp. Each point is the mean±SEM of nine samples in rested, exercised, infected and emersed animals and the mean±SEM of three samples in starved animals; glycogen was measured as glucose subsequent to treatment with glucoamylase; • = rested; ▾= exercised; ○ = emersed; ▵ = parasitized; ▪ = starved; Different letters indicate significant differences between means, p<0.05.
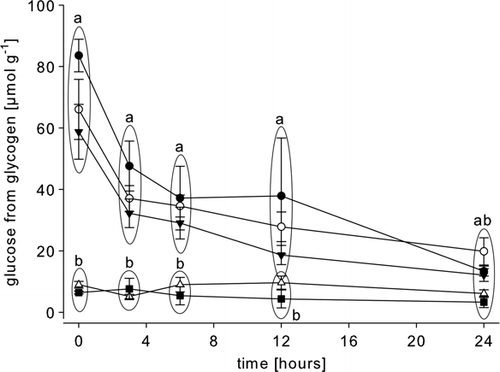
l-lactate
In animals subjected to a 5-min period of intense exercise, a significantly elevated concentration of l-lactate was found in the abdominal muscle, when compared to the value found in rested animals (, ). Later, at 3, 6, and 24 h post-mortem, the muscle l-lactate concentrations in the exercised and rested groups increased to similar values (), although at 12 h a significant difference was found between the two groups. In hypoxic animals after 2 h of emersion a significantly elevated concentration of l-lactate was also found in the abdominal muscle () and during the subsequent post-mortem period this value increased at the same rate as found in the exercised and rested animals (). In starved animals, only minimal amounts of l-lactate were detected initially in the abdominal muscle tissues (), and post-mortem the mean value never rose significantly (). The same was observed in the abdominal muscle of animals carrying a patent Hematodinium sp. parasite infection ( and ).
Figure 3. Post-mortem l-lactate accumulation in the abdominal muscle of rested Nephrops norvegicus muscle and after ante-mortem exercise, emersion, starvation and a patent parasite infection with Hematodinium sp. Each point is the mean±SEM of nine samples in rested, exercised, infected and emersed animals and the mean±SEM of three samples in starved animals; • = rested; ▾= exercised; ○ = emersed; ▵ = parasitized; ▪ = starved; means of the different treatments are compared at a given sampling time. Different letters indicate statistical differences between means, p<0.05.
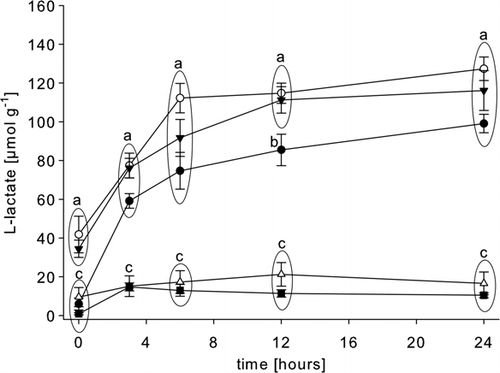
ATP and related nucleotides
The concentrations of ATP, ADP, AMP, IMP, HxR and Hx in the abdominal muscle of animals subjected to the various stressors, and the subsequent post-mortem changes are presented in and , respectively. The results from rested animals, presented in Gornik et al. (Citation2008), are also included for comparison.
Figure 4. Post-mortem changes in the concentrations of (a) ATP, (b) ADP, (c) AMP, (d) IMP), (e) inosine and (f) hypoxanthine in the abdominal muscle of rested Nephrops norvegicus muscle and after ante-mortem exercise, emersion, starvation and a patent parasite infection with Hematodinium sp. Each point is the mean±SEM of nine samples in rested, exercised, infected and emersed animals and the mean±SEM of three samples in starved animals; symbols: • = rested; ○= exercised; ▾= emersion; ▵ = parasite infection; ▪ = starvation.
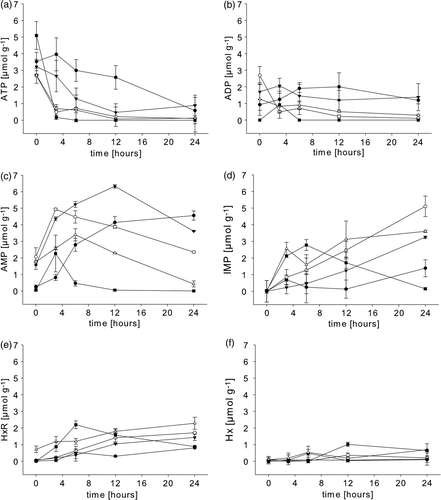
(a) Exercise
After intense exercise, the ATP concentration in the abdominal muscle was not significantly different from the value found in rested animals (). However, whereas in rested animals ATP was maintained around its initial value for up to 12 h post-mortem, in exercised animals it was rapidly depleted after 3 h (a). The initial concentration of ADP in the abdominal muscle of exercised animals decreased over the first 3 h post-mortem, and remained at this value thereafter (b). The AMP concentration in the abdominal muscle was elevated by exercise (). In rested animals AMP concentrations increased continuously for 24 h. In the exercised group the concentration of AMP increased from its initial elevated value for the first 3 h post-mortem, and then decreased (c). The IMP value was initially very low (, d) and increased in a linear manner during the sampling period (d). In both rested and exercised animals neither HxR nor Hx were detectable () at 0 h. Later, HxR was detected in both groups after 24 (e) and Hx was not detected at all (f). An effect of the ante-mortem exercise on the rate and extent of nucleotide inter-conversion is conveyed effectively by the AEC value. The period of intense exercise reduced the AEC significantly (). Differences were also apparent post-mortem. The AEC of rested animals fell steadily over the 24-h post-mortem period. In contrast, in the exercised group the AEC fell more rapidly in the first 3 h, to then remained stable ().
Figure 5. Post-mortem changes in the adenylate energy charge (AEC) values in the abdominal muscle of (a) rested Nephrops norvegicus muscle and after ante-mortem (a) exercise, (b) emersion, (c) starvation and (d) a patent parasite infection with Hematodinium sp. Each point is the mean±SEM of nine samples in rested, exercised, infected and emersed animals and the mean±SEM of three samples in starved animals; in (a): ○ = rested animals; • = exercised animals. Different letters indicate significant differences between means, p<0.05.
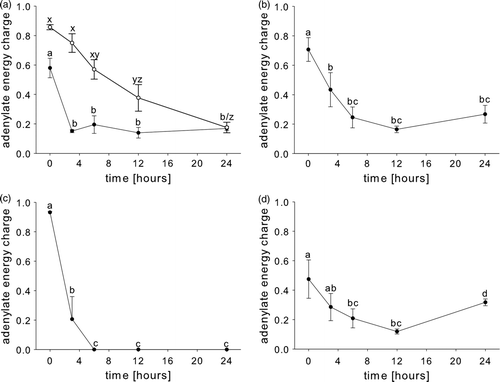
(b) Emersion
A general similarity was found between animals emersed for 2 h and animals subjected to intense exercise, and many features of the post-mortem changes in nucleotides were similar. However, the rates of change in the ‘emersion’ group were somewhat intermediate if compared to the conversion rates of rested and exercised animals. From its initial concentration ATP was depleted over the first 12 h post-mortem (a) with a more rapid rate than the one found for rested animals but less rapid if compared to ‘exercise’ individuals. The concentration of ADP did not change significantly for the whole sampling period (b). AMP increased initially for 12 h and then decreased between 12 and 24 h post-mortem (c). This was a similar pattern to that seen in the ‘exercise’ group, although the AMP peak was reached at a later time point. IMP was not detectable after emersion, but as for the ‘exercise’ group it increased in concentration in a linear manner over the whole post-mortem period (d). This rate of increase (0.12 µmol g–1 h–1) was lower than that for the ‘exercise’ group (0.20 µmol g–1 h–1). HxR was detected initially at 12 h post-mortem and increased over the remaining sampling period (e). Hx was not detected at all (f). The AEC fell immediately after the period of emersion () and reached a minimum at 12 h post-mortem, and thereafter showed no further significant change (b). The rate of fall was intermediate between those for the rested and exercised animals.
(c) Starvation
In the abdominal muscle of animals subjected to a 4-week starvation, the ATP concentration was not significantly different from that found in rested animals (). However, ATP was completely depleted over the first 3 h post-mortem, and was not detected thereafter (a). ADP was not detectable at any time (b). No AMP was measurable in the abdominal muscle directly after sacrifice, but post-mortem its concentration increased to peak at 3 h, and subsequently decreased until it was undetectable at 12 h and thereafter (c). IMP was initially at a very low concentration, but a premature peak of IMP was indicated at 6 h post-mortem. Values depleted gradually thereafter to reach a very low value at 24 h (d). This pattern is clearly different for those of the other groups, not only in its lower maximum value, but also particularly in terms of its early peak during the post-mortem period, and its subsequent reduction. HxR became detectable after 3 h, peaked at 6 h and then decreased (e). Hx was detectable after 12 h and thereafter (f). The AEC decreased significantly within 3 h (c) and thereafter it could not be calculated since ATP, ADP and AMP were at undetectable levels.
(d) Infection with Hematodinium sp
In the abdominal muscle of Nephrops norvegicus carrying a patent Hematodinium sp. infection, the ATP concentration was rapidly depleted post-mortem during the first 12 h (a). The ADP concentration was low initially and decreased further (b). AMP increased during the first 6 h and then decreased for the remainder of the sampling period (c). Interestingly, IMP values in Hematodinium sp. infected individuals showed a very small peak at 3 h and seemed not to increase significantly until between 12 and 24 h post-mortem, although the increase was never significant and infected animals seem to lack the ability to produce IMP post-mortem in significant amounts (d). HxR increased gradually over the whole sampling period (e). Hx was detectable only at the 6 and 24 h sample points (f). The AEC in parasitized animals decreased from an initially low value for 12 h, before rising for the remainder of the sampling period (d).
Post-mortem changes in the extracellular pH in the abdominal muscle of rested and starved animals
shows a comparison of the post-mortem changes in the extracellular pH of abdominal muscle of rested animals (which had been fed normally) and of starved Nephrops norvegicus. From an initial value of 7.57 immediately after sacrifice, the pH in the abdominal muscle of normally fed animals fell rapidly at first (20 min), and then more slowly for 5 h. It then remained steady for the next 15 h at low levels. The pH change in the muscles of starved animals was markedly different. The pH was lower initially (7.05) and increased to over the next 2 h (rather than decreasing as in the fed animals). The increase was followed by a rapid decrease over the next hour to 6.80, and then a linear increase at a rate of ~0.06 pH units h–1, so that after 20 h a value of approximately 7.92 was reached.
Figure 6. Post-mortem pH changes in the abdominal muscle of well-fed, rested Nephrops norvegicus and in the abdominal muscle of animals after 4 weeks of starvation. (•) pH changes in muscle of well-fed, rested animals; (○) pH changes in muscle of starved animals; the asterisk (*) indicates pH means found in the starved muscle which are significantly different from corresponding values found in the rested muscle (p<0.05); each point is the mean±SEM of three samples.
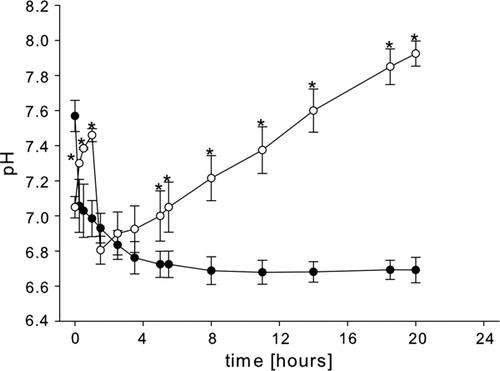
Discussion
Immediate effects of stress on stress-related metabolite values
The main immediate effect observed in Nephrops norvegicus muscle after the application of acute stresses such as exercise and emersion was a rapid progression towards anaerobic glycolysis. At the point of sacrifice, related metabolites revealed that an advanced glycolysis had taken place even before death occurred and haemolymph circulation ceased. This is consistent with the fact that exercise and emersion are both known to trigger anaerobic glycolysis in crustaceans (Taylor & Spicer Citation1987; Spicer et al. Citation1990; Morris & Adamczewska Citation2002). In N. norvegicus it has been shown by several authors that during high stress events (e.g. during trawl capture), where exercise and emersion are unavoidably combined, l-lactate levels in the haemolymph are elevated (Spicer et al. Citation1990; Ridgway et al. Citation2006a; Citationb; Albalat et al. Citation2009). The present study confirms this and also links exercise and emersion. Both deplete the glycogen stores by approximately 25%, and cause l-lactate to be produced prematurely in significant amounts, compared to rested muscle. Exercise and emersion are interchangeable in terms of their immediate effects on the animal. However, the results imply that exercise is a much more energy-demanding process than emersion, since during a 5-min period of intense exercise virtually the same amount of energy reserve was consumed as in a 2-h period of emersion.
Initial ATP levels were not affected by acute stresses. Moreover, they were not affected by any stress applied to the animals. This is not surprising, since stable ATP levels are essential for almost all cellular functions in all organisms and are rigidly maintained at physiological relevant levels. However, significant changes occur in ADP and AMP levels during acute stress. As a result, the AEC value calculated at the time of sacrifice is significantly reduced by both exercise and emersion, compared with that of the rested muscle, indicating a very low energy status. In this respect, a review from Atkinson (Citation1977) pointed out that a normal AEC values range from 0.87 to 0.94, while animals with AEC values lower than 0.5 are considered to be in irrecoverable physiological collapse (Sylvestre & Le Gal Citation1987). In the present study, AEC values for rested animals were in the normal range at the point of sacrifice. However, AEC values in the exercised and emersed animals were significantly reduced. This is analogous to observations in other crustaceans following exercise, e.g. in the prawn Palaemon serratus tail muscle (Thébault et al. 1997) and the crab Gecarcoidea natalis (Morris & Adamczewska Citation2002) or during hypoxia in the prawns Palaemon elegans and P. serratus (Taylor & Spicer Citation1987). Interestingly, the effect of emersion on the AEC seems to be species-dependent, as Carlsson & Gäde (1986) could show that hypoxia in the horseshoe crab Limulus polyphemus (Linnaeus, 1758) (Chelicerata, Xiphosura) did not affect the AEC in muscle over 48 h.
A striking feature of the results obtained by exposing the animals to the chronic stress of a four-week starvation or a patent systemic parasite infection is that no glycogen remains in the muscles and thus no anaerobic glycolysis can be observed post-mortem. This demonstrates, in consistency with results reported by Baden et al. (Citation1994) in a study of long-term starvation in Nephrops norvegicus, that glycogen represents an important metabolic storage molecule for N. norvegicus. Unfortunately, since tissue protein and lipid content were not investigated in the present study, glycogen cannot be referred to as the sole or most important storage metabolite utilized during starvation. It could also be that N. norvegicus switches from one storage molecule type to another as starvation progresses, or that several different storage molecules are in use at the same time.
The depletion of carbohydrate stores (mainly glycogen) during Hematodinium sp. infection as observed in the present study has been described previously. Stentiford et al. (Citation2001b) demonstrated that the parasite intervenes in the carbohydrate metabolism of the animal by breaking the feedback control loop involving crustacean hyperglycaemic hormone (CHH), which has a glucagon-like action (Chang Citation2001). When N. norvegicus is infected with Hematodinium sp. the CHH titre is persistently elevated in response to the glucose depletion by the parasite, and glycogen stores cannot be accumulated or maintained (Stentiford et al. Citation2001b). The authors suggest that this parasite imposes a functional starvation on its host, and the results of the present study would support this interpretation.
All stressors significantly depleted arginine phosphate (APi). In contrast to this, the depletion in the rested muscle was much slower. This demonstrates that arginine phosphate represents an important short-term phosphagen buffer in N. norvegicus and is depleted rapidly during times of high energy demand, e.g. during exercise. Albalat et al. (Citation2009) indirectly showed that N. norvegicus restores ATP by hydrolysis of APi when using rapid swimming movements (tail-flips) in response to threatening situations such as trawling. During escape from the trawl net, energy requirements may exceed the aerobic capacity of the muscle to produce ATP. In this situation, ATP is firstly formed from the hydrolysis of the muscle phosphagens before switching to glycolytic fermentation. The same has been observed in other species of arthropods. The horseshoe crab Limulus polyphemus, the terrestrial red crabs Gecarcoidea natalis, and the Australian yabby (Cherax destructor Clark, 1936), for example, use APi during exercise and tail-flipping (Carlsson & Gäde Citation1986; Morris & Adamczewska Citation2002).
The results of the present study show further that N. norvegicus uses APi for ATP reconstitution during emersion. In accordance with this, Abe et al. (Citation2007) found that the kuruma prawn Marsupenaeus japonicus utilizes APi during severe hypoxia. Starvation, whether systemic or induced by parasitism, also seems to reduce the APi levels, but the reasons for this can only be speculated. It is possible that, since the energy reserves of the animal are severely depleted during starvation, the muscle merely lacks the ability to recharge APi once it has been depleted during an earlier stress event, and thus APi levels remain low.
Do the different ante-mortem stressors predispose the abdominal muscle to different patterns of post-mortem change?
In animals stressed by exercise and emersion the glycogen stores are depleted and l-lactate is produced in significant amounts in vivo, but by 3 h post-mortem the values for l-lactate accumulation in these stressed animals converge with those found in rested muscle post-mortem (Gornik et al. Citation2008), and then follow the same trends. Thus, l-lactate values for exercised, emersed and rested muscle level out at a maximum plateau. It is possible that when the anaerobic glycolysis is triggered by ante-mortem exercise or emersion, its activation is initially enhanced and will therefore progress at a higher rate post-mortem, compared with rested individuals, but slows again when the majority of the stored glycogen is metabolized. Since one molecule of glucose is metabolized to form two molecules of l-lactate during glycolysis, the overall availability of glycogen dictates the maximum value reached. The post-mortem l-lactate in rested and acutely stressed animals only differs in the rate of accumulation not in the levels accumulated. Consistent with this suggestion that the rate of glycolysis is enhanced by metabolic stress, data obtained from vertebrates indicate that exercise induces and elevates the rate of glycolysis (Shen et al. Citation2006). However, no data for crustaceans are available to date and therefore, to confirm this theory, a more detailed study of the kinetics of the conversion of glycogen to lactate would be required. Also, this can only be true if a significant amount of glycogen is present in the animal before the stressor (e.g. exercise) is applied. If an animal is, for example, starved or infected with the blood-borne parasite Hematodinium sp. no such elevated lactate production can be observed. With regard to the post-mortem depletion of glycogen, the rested, exercised and hypoxic animals can be considered to display a common post-mortem response pattern, while starved and parasitized animals display a distinctly different response, presumably due to a lack of muscle glycogen store.
ATP is the near-exclusive nucleotide at the time of sacrifice under all conditions, and starting from this point a wave of rapid nucleotide inter-conversions (ATP→ADP→AMP→IMP→HxR) takes place post-mortem. The ability of rested Nephrops norvegicus to maintain ATP levels in muscle post-mortem is powerful (Gornik et al. Citation2008), but in the face of the both the acute and chronic stressors post-mortem ATP production cannot be maintained for long, and 3 h after sacrifice (or 12 h in the case of hypoxia) an almost complete depletion of ATP is observed. ADP seems to act as a transitional intermediate, leading to the formation of AMP, which peaks at distinct times under different conditions: 3 h after exercise and starvation, 6 h after infection and 12 h after hypoxia, compared to more than 24 h for rested animals. The wave of inter-conversion passes on most rapidly for the starved group, with both IMP and HxR peaking by 6 h, while IMP continues to accumulate for up to 24 h after exercise and emersion. In the parasitized animals, IMP is detected in much lower concentrations, although following a similar pattern compared to the starvation group. IMP is barely detected in rested animals in the first 24 h post-mortem.
It is somewhat surprising that the IMP-stage is reached so early post-mortem following ante-mortem stresses if compared to non-stressed values. In vertebrates IMP is established as a marker for muscular fatigue (Heller et al. Citation1986; Baldwin et al. Citation1999) and it is suggested that the early activation of anaerobic glycolysis causes an increased activity of the enzyme AMP deaminase (ADA), which converts AMP to IMP. It is known from studies in mammalian muscle and to some extent in fish muscle that exercise and stress activate the ADA (Rahim et al. Citation1979; Thébault et al. Citation2005). Current knowledge suggests that some alterations in the glycolytic pathway activate the AMP deaminase, which is otherwise inhibited in replete, rested muscle (see above). It is known that AMP induces the AMPK, which in turn activates the AMPKK, which activates phosphofructokinase, an important glycolytic enzyme. If no or a slow glycolytic response is taking place (due to the absence or slow release of glucose) the system becomes over-activated, and it is suggested that as a consequence IMP is accumulated in order to remove adenosine nucleotides (in the form of AMP) from the adenosine system to reduce the AMP signal strength and down-regulate the AMPK. However, the feedback loop involved is not yet fully explained and further research is needed. Also, it has been shown that a decrease in the ATP/ADP ratio, together with a decrease in the tissue pH, can be the stimulus for the activation of AMP deaminase in periods of intense muscular activity (Ranieri-Raggi et al. Citation1995). Furthermore, ADA is very likely to play a significant role in regulating the relative concentration of adenine nucleotides by stabilizing the AEC of contracting muscle (Rahim et al. Citation1979; Ranieri-Raggi et al. Citation1995). In starved animals, both pH and the ATP/ADP ratios behave in a way that allows us to assume ADA activation as described above. It is therefore fair to assume that that ADA activity is responsible for reaching the high IMP values prematurely if compared to non-stressed samples.
The changes in the post-mortem pH observed in the muscle of starved animals, when compared with that of normally fed animals, probably reflect the absence of anaerobic glycolysis in the former. The shape of the pH curve recorded from the muscle of starved animals is significantly altered: the initial pH is lower and increases, rather than decreases, during the first 3 h post-mortem. Only then does it decrease, at a rapid rate for 20 min, before increasing again for the remainder of the time. At this point, without further experiments, the sudden drop in the pH value of the starved group after approximately 3 h of increase cannot be explained easily. However, it is likely that the observed changes reflect changes in the biochemistry of the muscle induced by starvation. Usually the post-mortem accumulation of l-lactate lowers the pH significantly and causes it to remain at low values (as seen in the rested group). The increase of the pH after approximately 2.5 h in the starved group can be explained as follows: other biochemical processes (e.g. ATP breakdown or TMAO reduction) release ammonia and other nitrogenous compounds (Sikorski et al. 1990), which are known to increase the pH. It is, for example, known that NH4 + is released when AMP is transformed to IMP (Huss Citation1995). This liberation of NH4 + will, for its part, increase the pH, if in the absence of glycolysis no l-lactate is accumulated to oppose it.
The fact that APi levels were depleted to near baseline values within 3 h post-mortem by all four stressors, in contrast to the rested muscle in which the depletion was much slower, highlights that all applied stressors result in an increased energy demand of the muscle.
Implications for the product quality
From the results presented here certain predictions can be made about what effects different capture methods and post-harvest handling procedures will have on the post-mortem metabolism of Nephrops norvegicus and later product quality. Trawl capture, which involves both extensive escape swimming (Newland et al. Citation1992) and a degree of asphyxia (Harris & Andrews Citation2005a) represents a combination of two powerful acute stresses that would be expected to induce both strong glycolytic activity and an extensive depletion of energy reserves. In contrast, the creel capture method would be expected to be less stressful, since neither of these stresses is involved (although some tail flipping may occur during removal from the creel). A direct demonstration of these expectations has in fact recently been made by sampling N. norvegicus immediately after landing from trawl and creel capture; the former results in high tissue and haemolymph lactate levels, and a more rapid inter-conversion of nucleotides (Albalat et al. Citation2009).
Gornik et al. (Citation2008) demonstrated that post-mortem the abdominal muscle of N. norvegicus always exhibits an extensive glycolytic response, which leads to an unavoidable and rapid depletion of the energy. The present study now demonstrates that under artificially induced stress (mimicking capture stress and post-harvest handling) the consequences are an even further enhanced post-mortem energy depletion. If extrapolated, this result demonstrates that an animal, when subsequently sacrificed (by tailing or emersion in air) following trawling or creeling, will respond with an even more significant biochemical response compared with rested non-stressed (recovered) individuals during handling. The data in this study also show that the initial differences are later overcome and values for e.g. l-lactate converge 12–24 h post-mortem. The effects of this late convergence on product quality are hard to judge and sensory testing would be needed to elucidate if the early differences have a long-term effect on taste or palatability.
The enhanced stress-induced glycolytic fermentation results in an early accumulation of l-lactate in the muscle, and the l-lactate in turn lowers the pH more rapidly. In fish and red meat this post-mortem reduction in the pH of the muscle has an effect on its physical properties. As the pH drops, the net surface charge on the muscle proteins is reduced, which causes them to partially denature and lose some of their water-holding capacity. Muscle tissue in this state loses its moisture when cooked and is particularly unsuitable for further processing, since heat denaturation enhances the water loss (Huss Citation1995). It has been shown, e.g. by Love (Citation1979), that there is an inverse relationship between muscle toughness and pH, with unacceptable levels of toughness (and water-loss on cooking) occurring at lower pH levels. Hence, it is likely that N. norvegicus meat exhibiting a low pH (or high l-lactate) after catch and post-mortem will have an increased toughness when cooked. Following this it can be assumed that meat from exercised or emersed individuals (e.g. trawled animals) will be of lower quality when processed soon after catch than from rested animals (e.g. creel-caught). However, this is just an assumption and again a thorough study involving a professional sensory panel is required to clarify this.
A low pH does not necessarily mean a lower-quality product, since this depends on the time scale. If the pH is actually decreasing slowly post-mortem, a beneficial effect is observed: bacteria growth is reduced and hence bacterial spoiling delayed (Sikorski et al. 1996). Taking this into account one could argue that the enhanced post-mortem response (increased l-lactate values) induced by ante-mortem stress (e.g. during exercise/trawling) could result in a better product from a spoilage point of view. However, this is only speculation, since thorough bacteriological experiments would be needed to determine such an effect. Further, since this enhanced l-lactate accumulation is brought about rapidly through stress, as discussed above, it is likely to be generally more detrimental for the overall quality, even if a beneficial effect towards a longer shelf life would be indicated. It is long known for red meat and fish that ante-mortem stress causes quality loss and that in general great care is taken not to stress animals prior to slaughter. The same should be true for N. norvegicus lobsters.
We have shown that body condition, in terms of both the starvation level (which may vary with habitat and/or season), and a seasonal parasite infection, predisposes animals to a significantly altered post-mortem autolysis. Prolonged vivier-transport and/or tank recovery will lead to starvation, which in turn will lead to the already discussed predisposition. The common factor in these cases is the incapability of the animal to generate ATP through anaerobic glycolysis. Here, the l-lactate accumulation is much less pronounced compared to that in the muscle of starved or parasitized animals and its effects on quality, both beneficial or detrimental, can be neglected.
Starved and parasitized individuals showed a significantly different nucleotide inter-conversion pattern, with a much more rapid IMP accumulation compared with the ‘acute stress’ groups. Interestingly, IMP is now widely accepted as being responsible for the desirable fresh-fish flavour, which is present in high-quality seafood (Huss Citation1995). Starved animals will pass through the IMP maximum much more rapidly than others (within 6–12 h) and hence will be at their prime of taste earlier. This creates a problem for the processor since the good tasting meat is likely not to reach the consumer in time. Further, the levels of IMP are much reduced and the overall taste might hence be bland. Judging from the results of this study it can be assumed that starved and parasitized animals are likely to be of lower quality and palatability. Effects of HxR and Hx, of which especially Hx is known to induce bitter off-flavours (Huss Citation1995), can be neglected since they were not present at significant levels in any of the investigated post-mortem groups.
Conclusions
In conclusion, it can be said that this study has shown that different types of stress affect Nephrops norvegicus lobsters in different ways. The experimental animals in this study can be separated into two groups according to their stress responses: those subjected to the acute stresses of exercise and emersion and those experiencing the chronic stresses of starvation and infection. The acute stresses resulted in a rapid onset of glycolytic fermentation (glycolysis), leading to an early accumulation of its by-products (e.g. l-lactate) and an almost complete depletion of arginine phosphate. The chronic stressors resulted in a complete ante-mortem depletion of abdominal muscle glycogen and consequently the failure of post-mortem glycolytic fermentation. When the results of the laboratory-based experiments are extrapolated it has to be assumed that non-stressed Norway lobsters will generally be of a better quality than others. Exercised and emersed animals are likely to have tough meat and lower water-holding capacity due to enhanced l-lactate production and low pH values. At the same time, the low pH might be beneficial for the overall shelf life by inhibiting bacteria growth, but at a cost of a lower quality product. Starved individuals will pose a problem to the processor in general, since they are likely to be of inferior taste due to premature loss of IMP. Parasitized animals will be of similarly lower quality, although the presence of the parasite will also produce other detrimental effects, which have not been the subject of this study.
If the aim is to keep the animals alive for vivier-transport, the present study raises relevant questions about the relationship between fishing procedures and the metabolic responses of N. norvegicus. For example, what is the possibility that a trawl-caught animal, which can present an AEC values as low as 0.2 (Albalat et al. Citation2009), can recover to a normal state if restored to seawater? If these animals are also in poor body condition, due to starvation or infection, is there any likelihood that they can recover?
A study is now required in which the experimental results reported here are tested under ‘field’ conditions, and are also extended to examine the direct effects of ante-mortem stressors on product quality following long-term post-mortem storage by the utilization of, for example, a professional sensory panel and bacteriological assessments.
A more general conclusion of this study is that investigations of the post-harvest physiology of N. norvegicus and other crustaceans should always take into account the interplay between ante-mortem stresses and post-mortem changes, and the fact that the former can strongly influence the latter. Studies that do not minimize the ante-mortem stresses, or at least quantify them, can lead to erroneous conclusions being drawn about the effects of subsequent post-capture handling procedures.
Editorial responsibility: Alan Taylor
Acknowledgements
This work was supported by a grant from the EU Financial Instrument for Fisheries Guidance (FIFG) Scheme through the Scottish Executive, and by Young's Seafood Ltd.
Notes
Published in collaboration with the University of Bergen and the Institute of Marine Research, Norway, and the Marine Biological Laboratory, University of Copenhagen, Denmark
References
- Abe , H , Hirai , S and Okada , S. 2007 . Metabolic responses and arginine kinase expression under hypoxic stress of the kuruma prawn Marsupenaeus japonicus . Comparative Biochemistry and Physiology – Part A , 146 : 40 – 46 .
- Abdalla , ASA , Harrison , AP and Jensen , JF. 1999 . Effect of some ante-mortem stressors on peri-mortem and post-mortem biochemical changes and tenderness in broiler breast muscle: A review. World's . Poultry Science Journal , 55 : 403 – 14 .
- Aiken , DE. 1980 . “ Moulting and growth ” . In The Biology and Management of Lobsters , Edited by: Cobb , JS and Phillips , BF . 91 – 164 . New York : Academic Press .
- Albalat , A , Gornik , SG , Atkinson , RJA , Coombs , GH and Neil , DM. 2009 . Effect of capture method on the physiology and nucleotide breakdown products in the Norway lobster (Nephrops norvegicus) . Marine Biology Research , 5 : 441 – 50 .
- Ashie , INA , Smith , JP and Simpson , BK. 1996 . Spoilage and shelf-life extension of fresh fish and shellfish . Critical Reviews in Food Science and Nutrition , 36 : 87 – 121 .
- Atkinson , DE. 1965 . Biological feedback control at the molecular level . Science , 150 : 851 – 75 .
- Atkinson , DE. 1977 . Cellular Energy Metabolism and its Regulation , New York : Academic Press. 293 pages .
- Aubourg , S , Losada , V , Prado , M , Miranda , JM and Velázquez , JB. 2007 . Improvement of the commercial quality of chilled Norway lobster (Nephrops norvegicus) stored in slurry ice: Effects of a preliminary treatment with an antimelanosic agent on enzymatic browning . Food Chemistry , 103 : 741 – 48 .
- Baden , SP , Depledge , MH and Hagerman , L. 1994 . Glycogen depletion and altered copper and manganese handling in Nephrops norvegicus following starvation and exposure to hypoxia . Marine Ecology Progress Series , 103 : 65 – 72 .
- Baldwin , J , Snow , RJ , Carey , MF and Febbraio , MA. 1999 . Muscle IMP accumulation during fatiguing submaximal exercise in endurance trained and untrained men . American Journal of Physiology , 277 : 295 – 300 .
- Bayliss , P. 1995 . Chemistry in the kitchen: The chemistry of flesh foods I . Nutrition and Food Science , 1 : 31 – 35 .
- Beis , I and Newsholme , EA. 1975 . The contents of adenine nucleotides phosphagens and some glycolytic intermediates in resting muscle from vertebrates and invertebrates . Biochemical Journal , 152 : 23 – 32 .
- Bergmeyer , HU and Bernt , E. 1985 . “ Lactate dehydrogenase ” . In Methods of Enzymatic Analysis , Edited by: Bergmeyer , HU , Bergmeyer , J and Graβl , M . Vol. II , 574 – 79 . New York : Academic Press .
- Bergmeyer , HU , Bernt , E , Schmidt , E and Stork , H. 1985 . “ d-Glucose: Determination with hexokinase and glucose-6-phophate dehydrogenase ” . In Methods of Enzymatic Analysis , Edited by: Bergmeyer , HU , Bergmeyer , J and Graβ(l , M . Vol. III , 1196 – 201 . New York : Academic Press .
- Briggs , RP and McAliskey , A. 2002 . The prevalence of Hematodinium in Nephrops norvegicus from the western Irish Sea . Journal of the Marine Biological Association of the United Kingdom , 82 : 427 – 433 .
- Carlsson , KH and Gäde , G. 1986 . Metabolic adaptation of the horseshoe crab, Limulus polyphemus, during exercise and environmental hypoxia and subsequent recovery . Biological Bulletin , 171 : 217 – 35 .
- Chang , ES. 2001 . Crustacean hyperglycemic hormone family: Old paradigms and new perspectives . Integrative and Comparative Biology , 41 : 380 – 88 .
- Chinivasagam , HN , Bremner , HA , Thrower , SJ and Stephen , M. 1996 . Spoilage pattern of five species of Australian prawns: Deterioration is influenced by environment of capture and mode of storage . Journal of Aquatic Food Product Technology , 5 : 25 – 50 .
- Cuzon , G , Cahu , C , Aldrin , JF , Messager , JL , Stephan , G and Mevel , M. 1980 . Starvation effect on metabolism of Penaeus japonicus . Proceedings of the World Mariculture Society , 11 : 410 – 23 .
- Delbarre-Ladrat , C , Cheret , R , Taylor , R and Verrez-Bagnis , V. 2006 . Trends in post-mortem aging in fish: Understanding of proteolysis and disorganization of myofibrilliar structure . Critical Reviews in Food Science and Nutrition , 46 : 409 – 21 .
- Diouf , B and Rioux , P. 1999 . Use of the rigor mortis process as a tool for better understanding of skeletal muscle physiology: Effect of the ante-mortem stress on the progression of rigor mortis in brook charr (Salvelinus fontinalis) . American Biology Teacher , 61 : 376 – 79 .
- Ellington , WR. 1983 . The recovery from anaerobic metabolism in invertebrates . Journal of Experimental Zoology , 228 : 431 – 44 .
- Fraser , DI , Dyer , WJ , Weinstein , HM , Dingle , JR and Hines , JA. 1966 . Glycolytic metabolites and their distribution at death in the white and red muscle of cod following various degrees of antemortem muscular activity . Canadian Journal of Biochemistry , 44 : 1015 – 33 .
- Giomi , F , Raicevich , S , Giovanardi , O , Pranavi , F , Di Muro , P and Beltramini , M. 2008 . Catch me in winter! Seasonal variation in air temperature severely enhances physiological stress and mortality of species subjected to sorting operations and discarded during annual fishing activities . Hydrobiologia , 606 : 195 – 202 .
- Gornik , SG , Albalat , A , Atkinson , RJA , Coombs , GH and Neil , DM. 2008 . The time course of early post-mortem biochemical processes in the abdominal muscle of a commercially important decapod crustacean (Nephrops norvegicus): Implications for post-catch processing . Marine and Freshwater Behaviour and Physiology , 41 : 241 – 56 .
- Gornik , SG , Albalata , A , Atkinson , RJA and Neil , DM. 2009 . Biochemical investigations into the absence of rigor mortis in Norway lobster Nephrops norvegicus . Journal of Experimental Marine Biology and Ecology , 373 : 58 – 65 .
- Hagerman , L and Szaniawska , A. 1988 . Respiration, ventilation and circulation under hypoxia in the glacial relict Saduria (Mesidotea) entomon . Marine Ecology Progress Series , 47 : 55 – 63 .
- Harris , RR and Andrews , MB. 2005a . Physiological changes in the Norway lobster Nephrops norvegicus (L) escaping and discarded from commercial trawls on the West Coast of Scotland: I Body fluid volumes and haemolymph composition after capture and during recovery . Journal of Experimental Marine Biology and Ecology , 320 : 179 – 93 .
- Harris , RR and Andrews , MB. 2005b . Physiological changes in the Norway lobster Nephrops norvegicus (L) escaping and discarded from commercial trawls on the West Coast of Scotland: II Disturbances in haemolymph respiratory gases tissue metabolites and swimming performance after capture and during recovery . Journal of Experimental Marine Biology and Ecology , 320 : 195 – 210 .
- Heller , SL , Choksi , R and Brooke , MH. 1986 . IMP response: An indicator of metabolic stress in working muscle . Muscle Nerve , 9 : 515 – 18 .
- Hendricks , HB. 1965 . Influence of ante-mortem stress on meat palatability . Journal of Animal Science , 24 : 255 – 63 .
- Herreid , CF and Full , RJ. 1986 . Energetics of hermit crabs during locomotion: The cost of carrying a shell . Journal of Experimental Biology , 120 : 297 – 308 .
- Hervant , F , Garin , D , Mathieu , J and Freminet , A. 1999 . l-Lactate metabolism and glucose turnover in the subterranean crustacean Niphargus virei during post-hypoxia recovery . Journal of Experimental Biology , 202 : 579 – 92 .
- Hill , AD , Taylor , AC and Strang , RHC. 1991 . Physiological and metabolic responses of the shore crab Carcinus maenas (L) during environmental anoxia and subsequent recovery . Journal of Experimental Marine Biology and Ecology , 150 : 31 – 50 .
- Hooper , SL and Thuma , JB. 2005 . Invertebrate muscles: Muscle specific genes and proteins . Physiological Reviews , 85 : 1001 – 60 .
- Hoyle , G. 1969 . Comparative aspects of muscle . Annual Review of Physiology , 31 : 43 – 84 .
- Huss HH. 1995 Quality and quality changes in fresh fish. FAO Fisheries Technical Paper-348 http://wwwfaoorg/docrep/V7180E/V7180E00htm
- Lee , YB , Hargus , GL , Hagberg , EC and Forsythe , RH. 1976 . Effect of ante-mortem environmental temperatures on post-mortem glycolysis and tenderness in excised broiler breast muscle . Journal of Food Science , 42 : 1466
- Lewis , PK , Rakes , LY , Brown , CJ and Heck , MC. 1981 . Interaction between ante-mortem stress sampling time and pH found in certain pork muscle characteristics . Journal of Food Science , 46 : 1315 – 19 .
- Love , RM. 1979 . The post-mortem pH of cod and haddock muscle and its seasonal variation . Journal of the Science of Food and Agriculture , 30 : 433 – 37 .
- Lowe , TE , Ryder , JM , Carragher , JF and Wells , RMG. 1993 . Flesh quality in snapper Pagrus auratus affected by capture stress . Journal of Food Science , 58 : 770 – 73 .
- Mendes , R , Quinta , R and Nunes , ML. 2001 . Changes in baseline levels of nucleotides during ice storage of fish and crustaceans from the Portuguese coast . European Food Research and Technology , 212 : 141 – 46 .
- Morris , S and Adamczewska , AM. 2002 . Utilisation of glycogen ATP and arginine phosphate in exercise and recovery in terrestrial red crabs Gecarcoidea natalis . Comparative Biochemistry and Physiology – Part A , 133 : 813 – 25 .
- Neath , KE , Del Barrio , AN , Lapitan , RM , Herrera , JRV , Cruz , LC Fujihara , T . 2007 . Difference in tenderness and pH decline between water buffalo meat and beef during post-mortem ageing . Meat Science , 75 : 499 – 505 .
- Neil , DM , Fowler , WS and Tobasnick , G. 1993 . Myofibrillar protein composition correlates with histochemistry in fibres of the abdominal flexor muscle of the Norway lobster Nephrops norvegicus . Journal of Experimental Biology , 183 : 185 – 201 .
- Newland , PL , Chapman , CJ and Neil , DM. 1988 . Swimming performance and endurance of the Norway lobster Nephrops norvegicus . Marine Biology , 98 : 345 – 350 .
- Newland , PL , Neil , DM and Chapman , CJ. 1992 . Ecape swimming in the Norway lobster . Journal of Crustacean Biology , 12 : 342 – 53 .
- Paterson , BD. 1993 . The rise in inosine monophosphate and l-lactate concentrations in muscle of live penaeid prawns (Penaeus japonicus Penaeus monodon) stressed by storage out of water . Comparative Biochemistry and Physiology – Part B , 106 : 395 – 400 .
- Rahim , ZH , Lutaya , G and Griffiths , JR. 1979 . Activation of AMP aminohydrolase during skeletal–muscle contraction . Biochemical Journal , 184 : 173 – 76 .
- Ranieri-Raggi , M , Ronca , F , Sabbatini , A and Raggi , A. 1995 . Regulation of skeletal–muscle AMP deaminase: Involvement of histidine residues in the pH-dependent inhibition of the rabbit enzyme by ATP . Biochemical Journal , 309 : 845 – 52 .
- Ridgway , ID , Taylor , AC , Atkinson , RJA , Chang , ES and Neil , DM. 2006a . Impact of capture method and trawl duration on the health status of the Norway lobster Nephrops norvegicus . Journal of Experimental Marine Biology and Ecology , 339 : 135 – 47 .
- Ridgway , ID , Taylor , AC , Atkinson , RJA , Stentiford , GD , Chang , ES Chang , E . 2006b . Morbidity and mortality in Norway lobsters Nephrops norvegicus: Physiological immunological and pathological effects of aerial exposure . Journal of Experimental Marine Biology and Ecology , 328 : 251 – 64 .
- Ryder , JM. 1985 . Determination of adenosine triphosphate and its breakdown products in fish muscle by high-performance liquid chromatography . Journal of Agricultural and Food Chemistry , 33 : 678 – 80 .
- Sanchez-Paz , A , Garcia-Carreno , F , Muhlia-Almazan , A , Peregrino-Uriarte , AB , Hernandez-Lopez , H and Yepiz-Plascencia , G. 2006 . Usage of energy reserves in crustaceans during starvation: Status and future directions . Insect Biochemistry and Molecular Biology , 36 : 241 – 49 .
- Scheffler , TL and Gerrard , DE. 2007 . Mechanisms controlling pork quality development: The biochemistry controlling post-mortem energy metabolism . Meat Science , 77 : 7 – 16 .
- Schirf , VR , Turner , P , Selby , L , Hannapel , C , De La Cruz , P and Dehn , PF. 1987 . Nutritional status and energy metabolism of crayfish (Procambrus clarkii girard) muscle and hepatopancreas . Comparative Biochemistry and Physiology – Part A , 88 : 383 – 86 .
- Schmitt , ASC and Uglow , RF. 1997 . Effects of ambient ammonia levels on blood ammonia, ammonia excretion and heart and scaphognathite rates of Nephrops norvegicus . Marine Biology , 127 : 411 – 18 .
- Shen , QW , Means , WJ , Thompson , SA , Underwood , KR , Zhu , MJ McCormick , RJ . 2006 . Pre-slaughter transport AMP-activated protein kinase glycolysis and quality of pork loin . Meat Science , 74 : 388 – 95 .
- Shimada , R , Ushio , H and Yamanaka , H. 2000 . Initial changes in ATP equivalents in the post-mortem muscles of three species of lobster . Fisheries Science , 66 : 755 – 60 .
- Sidhu , GS , Montgomery , WA and Brown , MA. 1974 . Post-mortem changes and spoilage in Rock lobster muscle . Journal of Food Technology , 9 : 357 – 63 .
- Sigholt , T , Erikson , U , Rustad , T , Johansen , S , Nordtvedt , T and Seland , A. 1997 . Handling stress and storage temperature affect meat quality of farmed-raised Atlantic salmon (Salmo salar) . Journal of Food Science , 62 : 898 – 905 .
- Sikorski ZE Karlakowska A Burt JR. 1990 Postharvest biochemical and microbial changes Chapter 4 in Sikorski ZE Seafood: Resources, Nutritional Composition and Preservation Boca Raton, FL CRC Press Inc. 55 75
- Speed , SR , Baldwin , J and Wells , RMG. 2001 . Metabolic characteristics of muscles in the Spiny lobster Jasus edwardsii and responses to emersion during simulated live transport . Comparative Biochemistry and Physiology – Part B , 128 : 435 – 44 .
- Spicer , JI , Hill , AD , Taylor , AC and Strang , RHC. 1990 . Effect of aerial exposure on concentrations of selected metabolites in the blood of the Norway lobster Nephrops norvegicus (Crustacea: Nephropidae) . Marine Biology , 105 : 129 – 35 .
- Stentiford , GD , Neil , DM and Atkinson , RJA. 2001a . The relationship of Hematodinium infection prevalence in a Scottish Nephrops norvegicus population to season moulting and sex . ICES Journal of Marine Science , 58 : 814 – 23 .
- Stentiford , GD , Neil , DM and Coombs , GH. 2001b . Development and application of an immunoassay diagnostic technique for studying Hematodinium infections in Nephrops norvegicus populations . Diseases of Aquatic Organisms , 46 : 223 – 29 .
- Stentiford , GD and Shields , JD. 2005 . A review of the parasitic dinoflagellates Hematodinium species and Hematodinium-like infections in marine crustaceans . Diseases of Aquatic Organisms , 66 : 47 – 70 .
- Stroud , GD , Early , JC and Smith , GL. 1982 . Chemical and sensory changes in iced Nephrops norvegicus as indices of spoilage . International Journal of Food Science & Technology , 17 : 541
- Sylvestre , C and Le Gal , Y. 1987 . In situ measurements of adenylate energy charge and assessment of pollution . Marine Pollution Bulletin , 18 : 36 – 39 .
- Taylor , AC and Spicer , JI. 1987 . Metabolic responses of the prawns Palaemon elegans and P. serratus (Crustacean: Decapoda) to acute hypoxia and anoxia . Marine Biology , 95 : 521 – 30 .
- Thébault , MT , Izem , L , Leroy , JP , Gobin , E , Charrier , G and Raffin , JP. 2005 . AMP-deaminase in elasmobranch fish: A comparative histochemical and enzymatic study . Comparative Biochemistry and Physiology – Part B , 141 : 472 – 79 .
- Viant , MR , Rosenblum , ES and Tjeerdema , RS. 2001 . Optimized method for the determination of phosphoarginine in abalone tissue by high-performance liquid chromatography . Journal of Chromatography B , 765 : 107 – 11 .