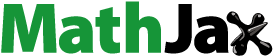
ABSTRACT
The post-heat treatment of laser powder bed fusion (LPBF) Sc-modified Al alloy is a remarkably necessary method to improve mechanical properties. In this work, the LPBF Al-Mn-Sc alloys with a high yield strength of 586 ± 4 MPa and excellent elongation of 14.5% were obtained by a novel and efficient non-isothermal aging (NIA) treatment at the heating rate of 2 °C/min from 30°C to 350°C. The precipitation behaviour and mechanical properties of the LPBF Al-Mn-Sc alloys after NIA treatment were investigated by differential scanning calorimetry (DSC), X-ray diffraction (XRD), transmission electron microscopy (TEM), hardness test as well as tensile test. The activation energy and Avrami exponent were calculated through DSC results with various heating rates. A non-isothermal aging kinetic model was applied to evaluate the NIA process referring to isothermal aging. The excellent mechanical property was analysed by revealing the strengthening mechanism of LPBF Al-Mn-Sc alloys after NIA.
1. Introduction
Aluminium alloys are widely used in automobiles, aerospace, weapons, electronics and energy on account of their lightweight, high strength-to-weight, excellent electrical and thermal conductivity and low cost [Citation1,Citation2]. However, the 3xxx series Al-Mn alloys have poor mechanical properties due to insufficient solid solution strengthening caused by the low solid solubility of Mn in the Al matrix and inferior precipitation strengthening resulted from the coarse and brittle Al-Mn phase after annealing [Citation3–5]. Laser powder bed fusion (LPBF) as an advanced additive manufacturing technology prepares components by melting the powder layer by layer using a high-energy laser beam based on computer-aided design (CAD) [Citation1,Citation6,Citation7]. The ultra-fast cooling rate of the LPBF process drastically improves the solid solubility of Mn in the Al matrix, and the addition of the transition elements or rare earth elements Sc and Zr refines the grains and reduces the hot cracking susceptibility [Citation8–11]. The incorporation of the transition or rare earth elements (Sc, Zr or Er) results in the formation of primary Al3X and serves as a heterogeneous nucleation site during the cooling of the melt pool due to the low lattice misfit with the matrix and the low energy barrier [Citation12,Citation13]. This favours the formation of a fine equiaxed grain structure that accommodates strain and restrains hot cracking susceptibility [Citation12]. As a result, Al-Mn-Sc alloys suitable for LPBF appear, which possess excellent mechanical properties much higher than those of the as-cast alloys. For additively manufactured alloys, subsequent heat treatment is performed as a necessary step in order to reduce internal stresses and further increase strength by utilising precipitation strengthening brought about by transition elements or rare earth elements [Citation14,Citation15].
The traditional isothermal aging (IA) is to keep the sample at a specific high temperature for a long time, which has many shortcomings, such as a long heat treatment process, low efficiency and high cost, and a new heat treatment of NIA has been developed [Citation16]. NIA heat-treats the sample through a continuous heating or cooling process, and the thermodynamic parameters will dynamically evolve under the changing temperature field [Citation17,Citation18]. The NIA regime was first introduced by Staley et al. and applied to aluminium alloys with a continuous linear heat treatment regime to increase strength [Citation19]. The application of NIA treatment on materials not only allows for adequate precipitation of precipitates by selecting the temperature range but also prevents coarsening of the precipitates by varying the heating rate [Citation20]. Therefore, the advantages of NIA are that the heat treatment time can be drastically reduced to lower the cost, and the intragranular and intercrystalline precipitates can be rationally regulated to obtain superior mechanical properties [Citation21–23]. However, for additively manufactured aluminium alloys with transition elements or rare earth elements such as Sc, Zr, Er, etc., most of them are isothermal heat treatment at about 300°C for aging up to 4–5 h [Citation7,Citation14,Citation15,Citation24,Citation25]. Long-term holding time ensures adequate precipitation of Al3X, but it also reduces the solid solution strengthening of LPBF brought about by extremely fast cooling. And prolonged holding time may also cause recrystallisation and growth of the microstructure. Therefore, the application of NIA to additive manufacturing alloys to avoid the shortcomings of isothermal aging while enhancing the strength and lowering the cost will provide a novel and advanced post-heat treatment path. However, there are few reports on NIA of additively manufactured alloys, especially Al-Mn-Sc alloys. Therefore, the objectives of this paper are to introduce efficient, low-cost non-isothermal aging for strength enhancement to avoid the inefficiency and high-cost problems of isothermal aging, and to elucidate the precipitation behaviour and strengthening mechanism of NIA in the additively manufactured Al-Mn-Sc alloys.
Furthermore, in this paper, the dense Al-Mn-Sc alloys were prepared using LPBF, and NIA was performed to obtain excellent mechanical properties. Based on the differential scanning calorimetry (DSC) results and an NIA kinetics model, the precipitation kinetics and equivalent aging time of Al-Mn-Sc alloys were investigated. The precipitation phases were analysed in detail by applying X-ray diffraction (XRD), transmission electron microscopy (TEM), and the strengthening mechanism was revealed.
2. Experimental procedure
2.1. Materials and preparation
The gas atomised Al-Mn-Sc powders were processed by LPBF on a commercial EOS M290 device in a protective atmosphere of argon. The previous studies showed that this Al-Mn-Sc alloy powder had a spherical morphology with an average particle size of about 20 μm [Citation26], which was conducive to laser preparation of dense high-strength alloys [Citation27]. The chemical components of the powders and LPBF alloys are shown in , indicating that almost no loss of the elements occurred during the laser melting process. The optimised process parameters were as follows: laser power of 370 W, scanning speed of 1100 mm/s, hatch space of 120 μm, layer thickness of 30 μm and scanning rotation of 67°. The subsequent non-isothermal aging (NIA) was performed at the heating rate of 1 °C/min, 2 °C/min, 5 °C/min and 8 °C/min from 30°C to 450°C. The hardness test was conducted on an HV hardness tester applying the load of 29.4 N for 15 s after each heat treatment. Based on the hardness results, the heating rate of 2 °C/min from 30°C to 350°C was chosen for NIA. The tensile specimens were obtained by cutting along perpendicular to the building direction of LPBF samples using wire cutting and the dimensions are shown in .
Table 1. The chemical components of the powders and the LBPF Al-Mn-Sc alloys (wt. %).
2.2. Structure and property characterisation
The samples with a thickness of about 30 μm were used to perform differential scanning calorimetry (DSC) to investigate the precipitation and stability of the phases on a DSC-700 device. The phases of as-built and NIA samples were examined using X-ray diffraction technique (XRD) (Smartlab SE, Japan, Cu-Kα radiation, 40 kV, 40 mA) at a scan rate of 2 °/min in the range of 10° to 90°. Transmission electron microscopy (TEM) and high-resolution TEM (HRTEM) were used to detect trace amounts of precipitates and the distribution of the precipitates, and TEM-EDS was used to probe the compositional content. The LPBF samples were prepared into thin slices with a thickness of 80 μm and 3 mm in diameter for TEM specimens. Then the specimens were electropolished with an electrolyte solution containing 30 vol% nitric acid and 70 vol% methanol at −25°C and 16 V using MTP-1 twinjet. The TEM and HRTEM were conducted on an FEI Talos F200X at 200 kV, and high angle annular dark field (HAADF) and TEM-EDS were carried out on an FEI Themis Z at 300 kV. The tensile test was conducted at a tensile speed of 1 mm/min on a Zwick Z2.5 TH universal tester equipped with a laser-Xtens laser extensometer. Three samples were tested for the as-built and NIA samples for tensile experiments respectively, and the average value was selected as the final tensile property.
3. Results
3.1. DSC and XRD analysis
shows the DSC curves of the as-built and non-isothermal aging (NIA) Al-Mn-Sc alloy at different heating rates. It could be found that each DSC curve had two exothermic peaks (Ⅰ and Ⅲ) and one endothermic peak (Ⅱ) at 50°C–600°C, and the increase of peak intensity and the shift of peak position from low temperature to high-temperature region occurred with the increase of heating rate, which might be related to the reduction of the precipitated Mn atoms due to the insufficient diffusion controlling the precipitation reactions at higher heating rates [Citation28–30], thus requiring higher temperatures and more heat to ensure adequate diffusion and precipitation reaction. The exothermic peak (Ⅰ) from 266°C to 350°C indicating the precipitation of phases could be identified as the precipitation of Al3(Sc,Zr) [Citation31]. The endothermic peak (Ⅱ) from 290°C to 372°C representing the dissolution of precipitates suggested a process of quasicrystal transition [Citation25], while the exothermic peak (Ⅲ) from 398°C to 476°C represented the formation of stable Al-Mn phases [Citation25,Citation31]. The abovementioned results were further confirmed by the XRD test conducted to detect precipitates for the as-built and NIA samples at the heating rate of 2 °C/min from 30°C to 350°C, as shown in (b). It was found that the as-built samples were dominated by icosahedral quasicrystal (QC) Al81Mn19 particles, while the main precipitated phases for NIA samples were QC-Al85(Mn0.72Fe0.28)14Si, Al4Mn and Al6Fe0.5Mn0.5 [Citation25,Citation32]. The phase transition occurred at peak Ⅱ of the DSC from 291°C to 350°C and the presence of Al81Mn19 in NIA samples indicated that a partial phase transition took place. It was shown that a stable Al-Mn phase could be formed at a temperature higher than 400℃.
3.2. Precipitation kinetics of non-isothermal
demonstrates the precipitation kinetics of Al-Sc and Al-Mn precipitates based on DSC curves with the heating rate of 1 °C/min, 5 °C/min, 10 °C/min and 20 °C/min. Based on equation (1), the activation energy (Q) of Al-Sc and Al-Mn was evaluated by Kissinger method [Citation33–35]. It was shown that the activation energy of Al-Sc precipitates was 111 ± 9 kJ/mol ((a)) and that of Al-Mn precipitates was 157 ± 8 kJ/mol ((b)). The transformed fraction (y) of Al-Sc and Al-Mn precipitates with the different heating rates was calculated by Equation (2) [Citation36], and by which the curves of integration of the peak Ⅰ and peak Ⅲ are plotted in (c,d). The transformed fraction decreased with the increase of heating rate at the same temperature. The Avrami exponent n of Al-Sc and Al-Mn precipitates was calculated by Equation (3) [Citation35], and acquired by the slope of the linear fit of three selected temperatures, as shown in (e,f). It could be found that the slope (n) varied with the change in temperature due to the influence of temperature [Citation37].
(1)
(1)
(2)
(2)
(3)
(3) where α is the heating rate, TP is the temperature of a peak in DSC curves, Q is the activation energy, R = 8.314 J/(mol·K) is the universal gas constant, CK is a constant, S and H are the total surface of the peak and the total enthalpy, respectively, ΔSj and ΔHj is the partial surface and enthalpy at the selected temperature, respectively.
3.3. Hardness and tensile properties
illustrates the microhardness distribution of the non-isothermal aging (NIA) samples with the heating rate of 1–8°C/min at 30°C–450°C and stress–strain curves of as-built and NIA samples. The microhardness evolution of NIA in (a) shows that the microhardness increased first and then decreased with the increase of temperature from 25°C to 450°C. The lower the heating rate, the lower the temperature was required to reach the respective maximum hardness, that is, when the heating rate is 1 °C/min and 2 °C/min, the temperature at which the respective maximum hardness was obtained was 350°C, and when the heating rate is 5 °C/min and 8 °C/min, the temperature to reach the respective maximum hardness was 400°C. The previous work showed that a peak value of 190 HV was achieved at 300°C for 5 h for IA [Citation26]. The hardness of NIA samples with the heating rate of 2 °C/min reached a peak value of 192 HV at 350°C, which was used to perform the tensile tests. The temperature reaching peak value was 350°C, which might be mainly related to the precipitation temperature of Al-Sc particles, as shown in . The tensile test results shown in (b) demonstrated that the yield strength and ultimate tensile strength of the as-built samples were 460 ± 5 MPa and 493 ± 8 MPa, respectively, and the yield strength and ultimate tensile strength increased to 586 ± 4 MPa and 600 ± 7 MPa after NIA, respectively, while the elongation decreased from 16.3% to 14.5%. Compared to IA [Citation26,Citation38], the samples treated with NIA could efficiently obtain close to or even higher yield strength in a shorter period of time, and the elongation was significantly better than that of IA samples.
3.4. TEM analysis
It could be seen from the DSC and XRD results in that the icosahedral quasicrystal QC-Al81Mn19 particles in as-built Al-Mn-Sc alloy were transformed into QC-Al85(Mn0.72Fe0.28)14Si, Al4Mn and Al6Fe0.5Mn0.5 by NIA with the heating rate of 2 °C/min from 30°C to 350°C. However, the distribution of the precipitates and the Al3(Sc,Zr) particles could not be detected due to low content, and further characterisation of precipitates by TEM is shown in . It was found that QC-Al81Mn19 and Al3(Sc,Zr) precipitates mainly distributed on the grain boundaries for as-built Al-Mn-Sc alloy in (a,d). The structure of QC-Al81Mn19 was confirmed by the HRTEM and fast Fourier transform (FFT) image, as shown in (c). (b) illustrates that the morphology of QC-Al81Mn19 was an elongated shape in the as-built Al-Mn-Sc alloy. After NIA, coarser Al-Mn precipitates with a volume fraction of 5% were observed in the NIA samples, as shown in (e). (f,g) demonstrates that a quasicrystal transition from QC Al81Mn19 to QC Al85(Mn0.72Fe0.28)14Si occurred, which was identified by HRTEM and FFT in (g), while more nano-scale Al3(Sc,Zr) with the particle size of 1.6 nm precipitated within the grains, as shown in (h). The possible chemical composition of matrix and Al-Mn precipitates were detected by TEM-EDS, as shown in (i–l). It could be found that after NIA, no significant content reduction of Mn and Mg elements occurred in the NIA samples, and the possible chemical compositions of the two quasi-crystals almost coincided with the characterisation results of XRD.
Figure 5. TEM images of as-built and NIA Al-Mn-Sc alloys: (a) low magnification, (b) high magnification, (c) HRTEM and fast Fourier transform (FFT) of 2-fold quasicrystal Al81Mn19, (d) HAADF and FFT of Al3(Sc,Zr) of as-built Al-Mn-Sc alloys; (e) low magnification, (f) high magnification, (g) HRTEM and FFT of 3-fold quasicrystal Al85(Mn0.72Fe0.28)14Si, (h) inverse fast Fourier transform (IFFT) of Al3(Sc,Zr) of non-isothermal Al-Mn-Sc alloys, and EDS mappings of the EDS 1 (i), EDS 2 (j), EDS 3 (k) and EDS 4 (l).
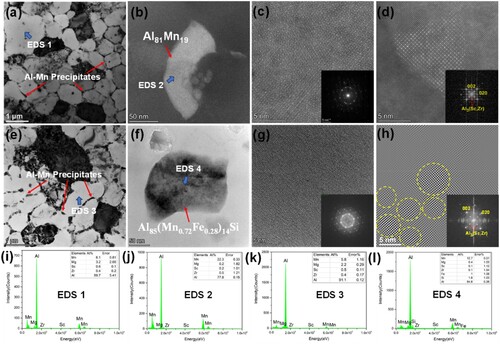
4. Discussion
4.1. Precipitation kinetics of LPBF Al-Mn-Sc alloy during NIA
DSC is considered to be an effective tool for studying precipitation kinetics and precipitation sequence of precipitates [Citation17]. The nano-scale Al3(Sc,Zr) precipitats first at 266°C–335°C, and then a phase transformation from QC Al81Mn19 to QC Al85(Mn0.72Fe0.28)14Si occurs from 291°C to 372°C, finally forming Al4Mn and Al6Fe0.5Mn0.5 at 398°C–476°C. For as-built Al-Mn-Sc alloy, the apparent activation energy Q associated with Al3(Sc,Zr) is 111 ± 9 kJ/mol, which is lower than the activation energy of diffusion of Sc [Citation39], but is in agreement with the activation energy of precipitation of Al3(Sc,Zr) precipitation in Al-Sc-Zr alloy and Al-Mn-Sc-Zr alloy within the limits of the experiment [Citation40,Citation41]. The average Avrami exponent n = 0.87 of the Al3(Sc,Zr) is close to 0.404 indicating that the intrinsically formed Sc-rich clusters exist as precursors for growth and no or less nucleation occurs [Citation24,Citation42]. The low activation energy of Al3(Sc, Zr) precipitation further suggests that the contribution of particle nucleation is not included, thus the growth of Al3(Sc,Zr) is dominated by interface-controlled growth through the reaction at the interface of matrix/particle [Citation41,Citation42]. The apparent activation energy Q of Al-Mn precipitation is determined as 157 ± 8 kJ/mol, which agrees with the apparent activation energy Q = 144 ± 16 kJ/mol of Al6(Fe, Mn) in AlMnFe-based alloy [Citation31,Citation43], and the average Avrami exponent n = 1.1 is close to the theory value 1.5 of three-dimensional diffusion-controlled growth, indicating that nucleation takes place in the pre-existing nucleation sites, and the phase transformation is controlled by diffusion [Citation30,Citation44]. Therefore, the precipitation of the Al-Mn phases may be related to a diffusion-driven phase transition during the NIA process. The shift to higher temperatures of Al-Sc and Al-Mn precipitation with the increase of the heating rate also indicates that the precipitation reaction of them is thermally activated and kinetically controlled [Citation17,Citation45].
The NIA process of LPBF Al-Mn-Sc alloys is evaluated using an NIA model with reference conditions of isothermal aging (IA) samples at 300°C for 5 h [Citation46], as shown in Equation (4–6). The curves of the evaluated equivalent aging degree of LPBF Al-Mn-Sc alloy for different heating rates are shown in . The aging efficiency is low at the beginning of NIA, which becomes longer with the decrease of heating rate, and then the aging efficiency increases exponentially with the increase of time and temperature. When the equivalent aging degree is 1, indicating that the NIA process has a similar structure and properties to the IA process, the equivalent aging time is evaluated. For the heating rate of 1 °C/min, it is comparable to the IA at 300°C for 5 h when the aging time reaches 5.61 h. As the heating rate increased from 1 °C/min to 2 °C/min, 5 °C/min and 8 °C/min, the equivalent aging time decreased from 5.61 to 2.98, 1.29 and 0.83 h. All estimated aging times are close to the time taken to reach respective maximum hardness at the corresponding heating rate are displayed in (a), as shown in , which means that the non-isothermal model is well suited for predicting non-isothermal processes of LPBF Al-Mn-Sc alloy.
(4)
(4)
(5)
(5)
(6)
(6) where T(t) is the instantaneous temperature at time t, T0 is the initial temperature.
Figure 6. The evolution of equivalent aging degree of LPBF Al-Mn-Sc alloy by NIA with the aging time referring to 300°C/5 h of IA samples.
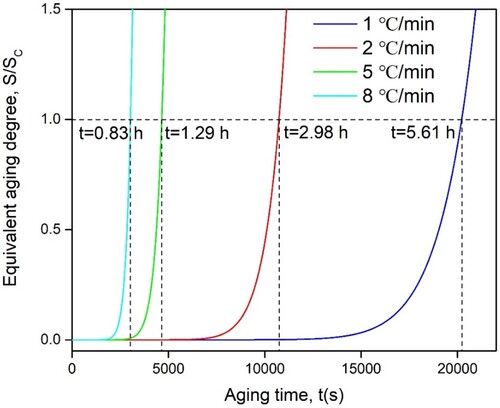
Table 2. The estimated and experimental value of aging time at different heating rate.
4.2. Precipitation of phases during the NIA process
The high yield strength of the as-built Al-Mn-Sc alloys is attributed to the precipitation of a large number of Al-Mn and Al-Sc particles due to the intrinsic heat treatment of LPBF. In the subsequent non-isothermal (NIA) heat treatment process, the phase transformation of Al-Mn and the precipitation of the nano-scale Al-Sc phase further improved its mechanical properties. The precipitation of Sc and Zr begins at about 250°C to be Al3Sc clusters (due to faster diffusion of Sc in Al), and gradually forms stable Al3(Sc,Zr) with the increase of temperature [Citation42]. The precipitation of Al3(Sc,Zr) has started at about 260°C–300°C at the heating rate of 1 °C/min, as shown in (a). The precipitation temperature of Al3(Sc,Zr) increased to about 280°C–350°C as the heating rate increased to 5 °C/min. Therefore, it can be inferred that at a heating rate of 1–5 °C/min, sufficient precipitation of Al3(Sc,Zr) can be obtained to enhance the strength before 350°C, which is further supported by the hardness distribution in (a). At a heating rate of 1 °C/min, the hardness increase is partially offset by reversion or recrystallisation due to the aging process that consumes more time when the maximum hardness is reached at 350°C. The increase in the precipitation temperature of Al3(Sc,Zr) at the heating rate of 5 and 8 °C/min makes it sufficient to precipitate at 400°C, but recrystallisation of LPBF Al-Mn-Sc occurs at this temperature resulting in a softening in microstructure [Citation26]. Thus, the heat treatment at a heating rate of 2 °C/min from 30°C to 350°C with a peak value of 192 HV at 350°C is used for non-isothermal aging (NIA). The Al3(Sc,Zr) precipitates in the as-built Al-Mn-Sc is mainly distributed at the grain boundaries and coherent with the Al matrix ((d)), and more nano-scale Al3(Sc,Zr) precipitates within the crystals after heating from 30°C to 350°C at a heating rate of 2 °C/min ((h)), which further strengthens the alloy.
The QC-Al81Mn19 is formed in the as-built Al-Mn-Sc alloy because of the extremely fast cooling rate during the LPBF process [Citation47,Citation48]. It can be inferred from peak Ⅱ in (a) that the phase transformation of Al81Mn19 to Al85(Mn0.72Fe0.28)14Si occurred at a heating rate of 2 °C/min up to 350°C because the Al-(Mn,Fe)Si phases have already begun to precipitate at a temperature above 300°C [Citation32,Citation49], and partially stabilised Al-Mn precipitates are formed in this process. The presence of QC-Al81Mn19 and the formation of QC-Al85(Mn0.72Fe0.28)14Si, Al4Mn and Al6Mn in the NIA sample shown in (b) and further clearly demonstrate that the phase transition of Al-Mn takes place. The strip-shaped QC-Al81Mn19 is mainly distributed at the grain boundary ((a,b)), and coarser Al-Mn precipitates are formed after NIA ((e)).
4.3. Strengthening mechanisms
The yield strength of as-built Al-Mn-Sc alloy is 460 MPa, which increases to 586 MPa after NIA, which may be enhanced by solid solution strengthening (σSSS), grain boundary strengthening (σGB) and precipitation strengthening (σPS) [Citation47]. Due to the extremely fast cooling rate in the LPBF process, high amounts of alloy elements can be supersaturated in the Al matrix, which causes localised strain field arising from a deformed lattice, hindering dislocation movement to achieve the solid solution strengthening [Citation38]. The estimated solid solution strengthening (mainly Mn and Mg) can be expressed as [Citation50]:
(7)
(7) where ω is an experimental constant, G = 25.4 GPa is the shear modulus of Al at room temperature [Citation50], c is the solute concentration in at.% which is obtained from (k). The solute concentration of Mn and Mg would present a reduction due to the transformation and precipitation of new Al-Mn phases in the NIA process [Citation47]. The estimated strength increment from solid solution strengthening after NIA is calculated as 103 MPa for Mn and 67 MPa for Mg. The strength contribution of solid solution strengthening suggests that NIA with a nearly doubled reduction in aging time well retains the solid solution strengthening of as-built Al-Mn-Sc alloys due to supersaturated solid solution.
Due to the inherent heat treatment of LPBF, a large amount of Al3(Sc,Zr) precipitates into heterogeneous nucleation sites, which substantially refine the grains and create high-density grain boundaries. The highly efficient heat treatment process of NIA does not result in significant structure evolution on account of the excellent thermal stability of LPBF Al-Mn-Sc alloys [Citation51]. The high amount of grain boundaries prevents the movement of dislocations and leads to grain boundary strengthening, which follows the Hall-Petch equation [Citation52]:
(8)
(8) where k = 0.17 MN m−3/2 describes the relative strengthening contribution from grain boundaries [Citation50], σ0 is the intrinsic resistance of the lattice to dislocation motion, d is the average grain diameter. Therefore, the estimated strength increment from grain boundaries strength after NIA is 197 MPa. Due to the effective aging process of NIA, there will be no significant recrystallisation as well as the growth of the grains. The average grain size is maintained at 1.1 ± 0.5 μm, providing better grain boundary strengthening than IA [Citation7,Citation38].
After performing NIA, the diffused Al-Mn particles formed by diffusion and nanoscale Al3(Sc,Zr) phases precipitate within the crystal ((e,h)), which plays a significant role in strength enhancement through interaction with dislocations, called precipitated strengthening (σPS). However, since the Al-Mn and Al-Sc precipitates have significantly distinct particle sizes, the dislocations interact with the two types of particles in different ways, and thus the respective strengthening mechanisms are different. For the Al-Mn precipitated phases with an average particle size of 39 nm, the particle bypassing occurs via the Orowan mechanism, whereas for the Al3X particles with an average size of only 1.6 nm, the modulus strengthening is determined to be the predominant mechanism due to the difference in modulus between the particles and the surrounding Al matrix. The Orowan mechanism (σOr) and modulus hardening (σms) can be given by [Citation38,Citation53]:
(9)
(9)
(10)
(10) where M = 3.06 is Taylor factor, λ is the inter-precipitate spacing [Citation53], R and Vv is the average particle size and volume fraction of precipitated particles obtained by TEM, as shown in . b = 0.286 nm is the Burgers vector for Al [Citation52]. ν = 0.345 is Poisson’s ratio of Al, ΔG = 42.5 GPa is the shear modulus mismatch between Al3(Sc, Zr) and Al matrix [Citation50]. m = 0.85 is a constant [Citation54]. The estimated strength increment of Al-Mn particles by the Orowan mechanism is 71 MPa, and the strength contribution of Al-Sc by modulus hardening is 141 MPa, which was lower compared to the precipitation strengthening contributed by isothermal aging (IA) [Citation26,Citation38,Citation47]. The total of the estimated strength increment is approximately in agreement with the experimental results.
Table 3. The parameters obtained from TEM.
Therefore, the strength increment contributed by solid solution strengthening is 170 MPa, accounting for about 29%, and the strength increment contributed by grain boundary strengthening is 197 MPa, accounting for about 34%, while the strength contribution by precipitation strengthening is 212 MPa, accounting for about 37%. It can be seen that the strength contribution of Al3(Sc,Zr) and Al-Mn precipitates caused by precipitation strengthening is the largest. For the precipitation strengthening, the strength increment provided by Al-Mn precipitates through the Orowan mechanism is 71 MPa, and the strength increment brought by modulus strengthening of Al3(Sc,Zr) is 141 MPa. Therefore, the precipitations Al3(Sc,Zr) have a more important effect on the mechanical behaviour. By revealing the strengthening mechanism of Al-Mn-Sc alloys after NIA, it can be found that the efficient heat treatment in non-isothermal aging retains both solid solution strengthening and fine grain size, and enhances strength through precipitation strengthening of precipitates Al81Mn19, Al85(Mn0.72Fe0.28)14Si, Al4Mn and Al6Mn and Al3(Sc,Zr), resulting in LPBF Al-Mn-Sc alloys with excellent mechanical properties.
5. Conclusions
In this paper, the LPBF Al-Mn-Sc alloys with excellent mechanical properties were obtained by using a novel non-isothermal thermal treatment at a heating rate of 2 °C/min from 30°C to 350°C. By analysing precipitation behaviour and mechanical properties, the conclusions are as follows:
By analysing the activation energies and Avrami exponent of Al-Sc and Al-Mn precipitates, it was found that the precipitation of Al3(Sc,Zr) after non-isothermal aging was mainly dominated by interface-controlled growth through the reaction at the interface of matrix/particle, whereas the phase transformation of Al-Mn precipitates was mainly dominated by diffusion-driven phase transition. The non-isothermal model proposed was well suited for predicting non-isothermal processes of LPBF Al-Mn-Sc alloy.
More nanoscale Al3(Sc,Zr) precipitated within the grain after non-isothermal aging. The precipitates QC-Al81Mn19 precipitated along the grain boundaries for as-built samples, whereas they transformed into coarser precipitates QC Al85(Mn0.72Fe0.28)14Si, Al4Mn and Al6Fe0.5Mn0.5 after non-isothermal aging.
The excellent mechanical properties of LPBF Al-Mn-Sc alloy are obtained by non-isothermal aging, which is equivalent to or even better than those obtained by isothermal aging. By revealing the strengthening mechanism, it is found that the excellent mechanical properties of the non-isothermally aged Al-Mn-Sc alloys are mainly attributed to the retention of the solid solution strengthening and fine grains by the highly efficient heat treatment, and the precipitation strengthening of the precipitates Al3(Sc,Zr), Al81Mn19, Al85(Mn0.72Fe0.28)14Si, Al4Mn and Al6Fe0.5Mn0.5.
Acknowledgements
The authors gratefully acknowledge the National Building Project of Application Demonstration Platform on New Materials Products (Project No. TC190H3ZV/2) for their supportive effect on finance. Wei Jiang would like to thank the support of the China Scholarship Council.
Disclosure statement
No potential conflict of interest was reported by the authors.
Data availability statement
The raw/processed data required to reproduce these findings cannot be shared at this time as the data also forms part of an ongoing study.
Additional information
Funding
References
- Zhang J, Song B, Wei Q, et al. A review of selective laser melting of aluminum alloys: processing, microstructure, property and developing trends. J Mater Sci Technol. 2019;35:270–284. doi:10.1016/j.jmst.2018.09.004
- Liu M, Wei K, Zhou R, et al. Microstructure and mechanical property of high power laser powder bed fusion AlSi10Mg alloy before and after T6 heat treatment. Virtual Phys Prototyp. 2022;17:749–767. doi:10.1080/17452759.2022.2068294
- Grushko B, Balanetskyy S. A study of phase equilibria in the Al-rich part of the Al–Mn alloy system. Int J Mater Res. 2008;99:1319–1323. doi:10.3139/146.101768
- Mair P, Letofsky-Papst I, Leichtfried G. Microstructural features and mechanical properties of a novel Ti- and Zr-modified Al-Mn alloy processed by laser powder bed fusion. J Alloys Compd. 2022;897:1–10. doi:10.1016/j.jallcom.2021.163156
- Mochugovskiy AG, Tabachkova NY, Mikhaylovskaya AV. Nanodispersoids of the quasicrystalline I-phase in Mn- and Mg-bearing aluminum-based alloys. Mater Lett. 2021;284:1–5. doi:10.1016/j.matlet.2020.128945
- DebRoy T, Wei HL, Zuback JS, et al. Additive manufacturing of metallic components – process, structure and properties. Prog Mater Sci. 2018;92:112–224. doi:10.1016/j.pmatsci.2017.10.001
- Zhao D, Han C, Peng B, et al. Corrosion fatigue behavior and anti-fatigue mechanisms of an additively manufactured biodegradable zinc-magnesium gyroid scaffold. Acta Biomater. 2022;153:614–629. doi:10.1016/j.actbio.2022.09.047
- Yu W, Xiao Z, Zhang X, et al. Processing and characterization of crack-free 7075 aluminum alloys with elemental Zr modification by laser powder bed fusion. Mater Sci Addit Manuf. 2022;1:1–11. doi:10.18063/msam.v1i1.4
- Kuo CN, Chua CK, Peng PC, et al. Microstructure evolution and mechanical property response via 3D printing parameter development of Al–Sc alloy. Virtual Phys Prototyp. 2020;15:120–129. doi:10.1080/17452759.2019.1698967
- Zhang H, Gu D, Dai D. Laser printing path and its influence on molten pool configuration, microstructure and mechanical properties of laser powder bed fusion processed rare earth element modified Al-Mg alloy. Virtual Phys Prototyp. 2022;17:308–328. doi:10.1080/17452759.2022.2036530
- Qi Y, Zhang H, Yang X, et al. Achieving superior high-temperature mechanical properties in Al-Cu-Li-Sc-Zr alloy with nano-scale microstructure via laser additive manufacturing. Materials Research Letters. 2024;12:17–25. doi:10.1080/21663831.2023.2285388
- Martin JH, Yahata BD, Hundley JM, et al. 3D printing of high-strength aluminium alloys. Nature. 2017;549:365–369. doi:10.1038/nature23894
- Zhang X, Xiao Z, Yu W, et al. Influence of erbium addition on the defects of selective laser-melted 7075 aluminium alloy. Virtual Phys Prototyp. 2022;17:406–418. doi:10.1080/17452759.2021.1990358
- Sun Y, Wang J, Shi Y, et al. An SLM-processed Er- and Zr-modified Al–Mg alloy: microstructure and mechanical properties at room and elevated temperatures. Mater Sci Eng A. 2023;883:1–12. doi:10.1016/j.msea.2023.145485
- Spierings AB, Dawson K, Kern K, et al. SLM-processed Sc- and Zr- modified Al-Mg alloy: mechanical properties and microstructural effects of heat treatment. Mater Sci Eng A. 2017;701:264–273. doi:10.1016/j.msea.2017.06.089
- Yuan S, Wu M, Yin X, et al. Synchronous improvement of strength and corrosion resistance by an improved variable-rate non-isothermal aging process in Al-Zn-Mg-Cu alloy. Mater Charact. 2024;207:1–15. doi:10.1016/j.matchar.2023.113491
- Wang J, Xie J, Mao Z, et al. Microstructure evolution and mechanical properties of the Al-Cu-Mg-Ag alloy during non-isothermal aging. J Alloys Compd. 2023;942:1–12. doi:10.1016/j.jallcom.2023.169031
- Nicolas M, Deschamps A. Characterisation and modelling of precipitate evolution in an Al–Zn–Mg alloy during non-isothermal heat treatments. Acta Mater. 2003;51:6077–6094. doi:10.1016/S1359-6454(03)00429-4
- Staley JT, Durham N. (2007). “Method and process of non-isothermal aging for aluminum alloys.” Us Patent 20070267113A1.
- Zang C, Xiao W, Fu Y, et al. Enhanced properties and homogeneity of Al-Zn-Mg-Cu alloy thick plate by non-isothermal aging. J Alloys Compd. 2023;952:1–16. doi:10.1016/j.jallcom.2023.170023
- Jiang JT, Xiao WQ, Yang L, et al. Ageing behavior and stress corrosion cracking resistance of a non-isothermally aged Al–Zn–Mg–Cu alloy. Mater Sci Eng A. 2014;605:167–175. doi:10.1016/j.msea.2014.03.023
- Liu Y, Jiang D, Li B, et al. Effect of cooling aging on microstructure and mechanical properties of an Al–Zn–Mg–Cu alloy. Mater Des. 2014;57:79–86. doi:10.1016/j.matdes.2013.12.024
- Peng X, Guo Q, Liang X, et al. Mechanical properties, corrosion behavior and microstructures of a non-isothermal ageing treated Al-Zn-Mg-Cu alloy. Mater Sci Eng A. 2017;688:146–154. doi:10.1016/j.msea.2017.01.086
- Jia Q, Zhang F, Rometsch P, et al. Precipitation kinetics, microstructure evolution and mechanical behavior of a developed Al–Mn–Sc alloy fabricated by selective laser melting. Acta Mater. 2020;193:239–251. doi:10.1016/j.actamat.2020.04.015
- Wang M, Li R, Yuan T, et al. The evolution of quasicrystal during additive manufacturing and aging treatment of a Si-modified Al–Mn-Sc alloy. Mater Sci Eng A. 2022;859:1–7. doi:10.1016/j.msea.2022.144206
- Jiang W, Deng Y, Guo X. Effect of heat treatment on microstructure and mechanical anisotropy of selective laser melted Al–Mn-Sc alloy. Mater Sci Eng A. 2023;887:1–11. doi:10.1016/j.msea.2023.145743
- Yu WH, Sing SL, Chua CK, et al. Particle-reinforced metal matrix nanocomposites fabricated by selective laser melting: A state of the art review. Prog Mater Sci. 2019;104:330–379. doi:10.1016/j.pmatsci.2019.04.006
- Kongthep J, Juijerm P. Kinetics of precipitation hardening phase in aluminium alloy AA 6110. Mater Sci Technol. 2014;30:1815–1819. doi:10.1179/1743284713Y.0000000488
- Varschavsky A, Donoso E. A differential scanning calorimetrie study of precipitation in Cu-2Be. Thermochim Acta. 1995;266:257–275. doi:10.1016/0040-6031(95)02338-0
- Fatmi M, Ghebouli B, Ghebouli MA, et al. The kinetics of precipitation in Al-2.4wt% Cu alloy by kissinger, ozawa, bosswel and matusita methods. Phys B. 2011;406:2277–2280. doi:10.1016/j.physb.2011.03.053
- Vlach M, Stulikova I, Smola B, et al. Precipitation in cold-rolled Al–Sc–Zr and Al–Mn–Sc–Zr alloys prepared by powder metallurgy. Mater Charact. 2013;86:59–68. doi:10.1016/j.matchar.2013.09.010
- Pan S, Qian F, Li C, et al. Synergistic strengthening by nano-sized α-Al(Mn,Fe)Si and Al3Zr dispersoids in a heat-resistant Al–Mn–Fe–Si–Zr alloy. Mater Sci Eng A. 2021;819:1–12. doi:10.1016/j.msea.2021.141460
- Khan IN, Starink MJ, Yan JL. A model for precipitation kinetics and strengthening in Al–Cu–Mg alloys. Mater Sci Eng A. 2008;472:66–74. doi:10.1016/j.msea.2007.03.033
- Djema O, Bouabdallah M, Badji R, et al. Isothermal and non-isothermal precipitation kinetics in Al–Mg–Si-(Ag) alloy. Mater Chem Phys. 2020;240:1–9. doi:10.1016/j.matchemphys.2019.122073
- Boumerzoug Z, Fatmi M. Effect of heat treatments on discontinuous precipitation kinetics in Al-30 wt.% Zn alloy. Mater Charact. 2009;60:768–774. doi:10.1016/j.matchar.2008.12.015
- Guía-Tello JC, Garay-Reyes CG, Ruiz-Esparza-Rodríguez MA, et al. Effect of plastic deformation on the precipitation reaction in 2024 alloys. Mater Chem Phys. 2021;271:1–5. doi:10.1016/j.matchemphys.2021.124927
- Eivani AR, Taheri AK. Modeling age hardening kinetics of an Al–Mg–Si–Cu aluminum alloy. J Mater Process Technol. 2008;205:388–393. doi:10.1016/j.jmatprotec.2007.11.195
- Jia Q, Rometsch P, Kürnsteiner P, et al. Selective laser melting of a high strength Al Mn Sc alloy: alloy design and strengthening mechanisms. Acta Mater. 2019;171:108–118. doi:10.1016/j.actamat.2019.04.014
- Jo H, Fujikawa S. Kinetics of precipitation in Al-Sc alloys and low temperature solid solubility of scandium in aluminium studied by electrical resistivity measurements. Mater Sci Eng A. 1993;171:151–161. doi:10.1016/0921-5093(93)90401-Y
- Vlach M, Stulikova I, Smola B, et al. Effect of cold rolling on precipitation processes in Al–Mn–Sc–Zr alloy. Mater Sci Eng A. 2012;548:27–32. doi:10.1016/j.msea.2012.03.063
- Vlach M, Stulíková I, Smola B, et al. Phase transformations in isochronally annealed mould-cast and cold-rolled Al–Sc–Zr-based alloy. J Alloys Compd. 2010;492:143–148. doi:10.1016/j.jallcom.2009.11.126
- Senkov ON, Shagiev MR, Senkova SV, et al. Precipitation of Al3(Sc,Zr) particles in an Al–Zn–Mg–Cu–Sc–Zr alloy during conventional solution heat treatment and its effect on tensile properties. Acta Mater. 2008;56:3723–3738. doi:10.1016/j.actamat.2008.04.005
- Luiggi NJ. Analysis of thermoelectric power measurements in the study of precipitation kinetics in 3003 Al alloy. Metall Mater Trans B. 1997;28:149–159. doi:10.1007/s11663-997-0137-9
- Wierszyłłowski I, Wieczorek S, Stankowiak A, et al. Kinetics of transformation during supersaturation and aging of the Al-4.7mass%Cu alloy: grain size, dilatometric, and differential thermal analysis studies. J Phase Equilibria Diffus. 2005;26:555–560. doi:10.1007/s11669-005-0050-3
- Ghosh KS, Gao N. Determination of kinetic parameters from calorimetric study of solid state reactions in 7150 Al-Zn-Mg alloy. Trans Nonferrous Met Soc China. 2011;21:1199–1209. doi:10.1016/S1003-6326(11)60843-1
- Feng D, Zhang X, Liu S, et al. Non-isothermal retrogression kinetics for grain boundary precipitate of 7A55 aluminum alloy. Trans Nonferrous Met Soc China. 2014;24:2122–2129. doi:10.1016/S1003-6326(14)63322-7
- Zhao J, Wang B, Liu T, et al. Study of in situ formed quasicrystals in Al-Mn based alloys fabricated by SLM. J Alloys Compd. 2022;909:164847. doi:10.1016/j.jallcom.2022.164847
- Gargarella P, Almeida A, Vilar R, et al. Microstructural characterization of a laser remelted coating of Al91Fe4Cr3Ti2 quasicrystalline alloy. Scr Mater. 2009;61:709–712. doi:10.1016/j.scriptamat.2009.06.010
- Qian F, Jin S, Sha G, et al. Enhanced dispersoid precipitation and dispersion strengthening in an Al alloy by microalloying with Cd. Acta Mater. 2018;157:114–125. doi:10.1016/j.actamat.2018.07.001
- Vo NQ, Dunand DC, Seidman DN. Atom probe tomographic study of a friction-stir-processed Al–Mg–Sc alloy. Acta Mater. 2012;60:7078–7089. doi:10.1016/j.actamat.2012.09.015
- Zhang Z, Sun J, Wu J, et al. Influence of heat treatment on corrosion behavior of Al–Mn–Mg–Sc–Zr alloy produced by laser powder bed fusion. J Mater Res Technol. 2023;23:4734–4746. doi:10.1016/j.jmrt.2023.02.029
- Li R, Wang M, Li Z, et al. Developing a high-strength Al-Mg-Si-Sc-Zr alloy for selective laser melting: crack-inhibiting and multiple strengthening mechanisms. Acta Mater. 2020;193:83–98. doi:10.1016/j.actamat.2020.03.060
- Fuller CB, Seidman DN, Dunand DC. Mechanical properties of Al(Sc,Zr) alloys at ambient and elevated temperatures. Acta Mater. 2003;51:4803–4814. doi:10.1016/S1359-6454(03)00320-3
- Krug ME, Mao Z, Seidman DN, et al. Comparison between dislocation dynamics model predictions and experiments in precipitation-strengthened Al–Li–Sc alloys. Acta Mater. 2014;79:382–395. doi:10.1016/j.actamat.2014.06.038