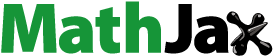
ABSTRACT
There is a huge need for energy storage devices due to the depletion of natural gas and the increasing requirement for portable electronic gadgets. Fused deposition modeling (FDM) 3D-printing has drawn tremendous interest for the fabrication of batteries and supercapacitors (SCs) due to its tabletop manufacturing technique, bespoke design, fast prototyping and user-friendly process. However, there are fewer available conductive filaments for FDM printing that are ideal from an energy storage standpoint. 2D transition metal dichalcogenide WS2 has been discovered to be a favourable material for electrochemical energy storage. As a result, in this work, we modified a carbon electrode that was 3D-printed by incorporating WS2 in order to enhance the capacitive performance of the SC electrode. The WS2-coated 3D-printed carbon electrode (WS2/3D-PCE) exhibits 2.8 times higher specific capacitance than the 3D-printed carbon electrode at 50 mV s−1. A solid-state symmetric supercapacitor (SS-SC) was fabricated with WS2/3D-PCE and polyvinyl alcohol (PVA)/Li2SO4 as gel electrolytes. Such modified 3D-PCE opens up the opportunities to design any custom-shaped electrode with tailored properties and pave a route for future research that will lead to more electrochemical devices for portable electronics.
Introduction
There has been a fast evolution toward designing and manufacturing complex structures using additive manufacturing or 3D printing techniques in recent years [Citation1, Citation2]. These open the possibility of fabricating inexpensive 3D components for various applications with ease [Citation3]. Advancements in the wearable and portable electronics industry have intensified the need for flexible and miniaturised electrochemical energy storage (EES) devices [Citation4, Citation5]. Besides scalability and size, superior power density, excellent energy density, and charging–discharging capability are essential considerations for EES design [Citation6–8]. In this regard, SCs are gaining popularity as an energy storage technology due to their good energy density, high power density, and excellent cyclic stability compared to Li-ion batteries [Citation9–11].
In order to accommodate in complex 3D electrical devices as power sources, SC parts, such as the anode, cathode, current collectors, and separators, should be tailored to a preferred structure and dimension [Citation12, Citation13]. The advancement of 3D printing processes opens up new avenues for tackling this significant difficulty for supercapacitors. Recently, researchers explored various 3D-printing technologies to fabricate 3D electrodes for SC applications [Citation12–18]. FDM, one of several 3D-printing methods, has been identified as a viable method for the manufacturing of SCs owing to its affordable and simple fabrication process [Citation1, Citation19]. Despite drawbacks, such as lower resolution than other technologies, its rapid printing speed, versatility in material options and ease of usage make it appropriate for applications, particularly in fields where these factors play a crucial role. In the FDM system, the 3D structure of the desired item is built layer by layer via extruding the filament through a nozzle [Citation20]. Thermoplastic materials, especially polylactic acid (PLA) or acrylonitrile–butadiene–styrene (ABS) polymers, are often used to make the filament used in FDM printing [Citation21, Citation22]. Foster et al. fabricated SC electrodes utilising a commercially available graphene-containing PLA filament. This filament has ∼92 wt% PLA and ∼8 wt% graphene and obtained a specific capacity of 15.8 mA h g−1 [Citation23]. However, the ease with which such a conductive filament opens up new possibilities for manufacturing electrode materials with the required design. Our group has recently conducted investigations to build electrodes for various electrochemical and sensing applications utilising commercial conductive graphene/PLA filament [Citation24–30]. One of the main challenges while using conductive graphene/PLA filament is their high polymer content affecting the electrochemical activity of the electrode. Deposition of electrochemically effective substance on the 3D-printed electrode can hasten the adoption of the FDM technology for the construction of energy storage devices [Citation31, Citation32].
Separately, 2D transition metal dichalcogenide, namely WS2, due to its unique electrical and structural features, has garnered interest as a possible material for energy storage devices [Citation33–36]. The layers of a multilayer WS2 are held together with van der Waals forces, allowing for facile ion intercalation. The tungsten also exhibits intriguing pseudocapacitive behaviour owing to its broad variety of valence states. Furthermore, the theoretical capacitance of WS2 material is high (433 mA h g−1) [Citation37, Citation38]. The benefits of WS2 as a prospective electrode material for supercapacitors include its low price, large-scale availability, and the possibility of synthesis by low-cost vacuum-free procedures such as hydrothermal method, chemical exfoliation, and so on [Citation39–41]. Electrodeposition has been proven to be an easier and more inexpensive procedure than most synthesis techniques that need intricate routes and expensive vacuum technology. Furthermore, compared to exfoliation and solution phase procedures, electrodeposition creates nano-to-micrometre films quickly and with high command over film deposition. Nonetheless, a WS2 deposition might be an excellent pseudocapacitive electrode material for efficient SC design. Pseudocapacitance and overall capacitive performance will be improved with a fine layer deposition of WS2 since the electrochemical energy storage process of SC is primarily restricted to the surface of the electrode.
Herein, we have designed a scalable and low-cost technique to fabricate a supercapacitor by ambient temperature electrodeposition of WS2 on chemically activated 3D-PCE. The WS2 electrodeposited 3D-PCE functions as a stand-alone electrode, eliminating the requirement for an external current collector. Thus, a low weight-to-volume ratio is attained by removing an extra component from SC. WS2 deposition on the surface of activated 3D-PCE improves the overall performance of the 3D electrode by pseudocapacitive reactions. Furthermore, SS-SCs have been constructed using PVA/Li2SO4 gel electrolyte. The solid-state electrolyte guarantees the secure usage of SS-SC in electrical devices, avoiding the electrolyte leakage issue that usually happens with liquid electrolytes. In a nutshell, our work illuminates a different path for ambient-temperature WS2 deposition on tailored free-standing 3D-PCE for supercapacitor electrodes.
Experimental methods
Materials
All materials used were of analytical grades such as ammonium tetrathiotungstate (NH4)2WS4, potassium chloride (KCl), lithium sulfate anhydrous (Li2SO4), and polyvinyl alcohol (MW 85,000–124,000 g mol−1) acquired from Sigma Aldrich, Germany. Sodium hydroxide (NaOH) was procured from Penta chemicals unlimited, Czech Republic. The conductive graphene/polylactic acid (PLA) filament (Blackmagic®) was acquired from Graphene Laboratories Inc, USA.
3D printing and activation of the electrodes
Autodesk Fusion 360 software was used to design the electrodes for the 3D printing. The electrodes were designed in the shape of a circular lollipop on a stick, with a 6 mm diameter circular disc electrode and a 15 × 2 mm rectangular stick attached to it as shown in Figure S7a. The printed parts had a thickness of 0.5 mm. This file is then sliced using PrusaSlicer 2.6.1 software keeping infill at 15%. A layer height of 0.2 mm was set for the initial layer and 0.1 mm for the consecutive layers. The default setting of the gyroid fill pattern was used keeping the top fill and bottom fill pattern monotonic. The sliced electrode file was used for the fused filament fabrication with conductive carbon/PLA filament. The electrodes were 3D-printed using a Prusa i3 MK3 printer (Prusa Research, Czech Republic) equipped with an Olsson Ruby ruby-tipped 0.6 mm nozzle (3DVerkstan, Sweden). The printing temperature was set at 215 °C for the nozzle and 60 °C for the bed. The 3D-printed carbon/PLA electrodes were then activated chemically by dipping for 5 h in a 4 M NaOH solution. Then electrochemical activation was carried out in a phosphate buffer solution (PBS) having pH 7.2 utilising a Pt wire as the counter electrode, and a constant potential of 2 V vs Ag/AgCl was applied for 150 s. Such activated electrodes are then denoted as 3D-PCE.
Preparation of WS2/3D-printed carbon electrodes
The electrodeposition of WS2 was carried out using previously optimised parameters from our group [Citation42]. For the electrodeposition, 10 mM of (NH4)2WS4 solution was prepared in 0.1 M KCl solution. Electrodeposition of WS2 on 3D-PCE was carried out by running CV from −1.3 to +1.1 V at 50 mV s–1 for 50 cycles. The WS2-coated 3D-PCE is represented as WS2/3D-PCE in the subsequent sections. An average mass deposition of ∼0.23 mg cm−2 of WS2 was obtained through gravimetric measurement.
Fabrication of solid-state supercapacitor cell
A PVA/Li2SO4 gel electrolyte was used to assemble two identical electrodes to create the SS-SC cell. There is no need for an external separator since the gel electrolyte acts as both an electrolyte and a separator in the cell. The preparation of the PVA/Li2SO4 gel electrolyte was carried out using an altered technique, as outlined in the literature [Citation29, Citation43]. The preparation process of the PVA/Li2SO4 gel electrolyte can be summarised as follows: A clear PVA solution was prepared by dissolving 1 g of PVA in 5 ml of water heating at 90 °C. Then, 4 mL of 1 M Li2SO4 was gradually added drop by drop. The solution was then cooled to ambient temperature and placed in a glass vial. The resulting gel was utilised for making SS-SCs.
Materials characterisation
Electrochemical and materials characterisations were carried out for 3D-PCE and WS2/3D-PCE. Scanning Electron Microscope (SEM, FEI VERIOS 460L) was used to visualise the surface of the electrodes. Energy-dispersive X-ray (EDX) was analyzed using a detector (EDAX SDD Octane Super) coupled with the SEM for the elemental mapping and chemical composition applying an electron beam accelerating voltage of 18 kV. AXIS Supra instrument (Kratos Analytical, Japan) was utilised for X-ray photoelectron spectroscopy (XPS) measurements using a monochromatic Al Kα (1486.7 eV) excitation source used to examine the bonding and atomic composition of the electrodes. The following spectra obtained from the XPS were analyzed using Casa XPS software. The Shirley algorithm was used for background correction of the XPS data.
Electrochemical performances were analyzed using cyclic voltammetry (CV), galvanostatic charge-discharge (GCD) and electrochemical impedance spectroscopy (EIS) using a potentiostat (Metrohm Autolab, Netherland) employing NOVA 2.1.5 software in a 20 mL cell at room temperature with Ag/AgCl (1 M KCl) and Pt as a reference and counter electrodes, respectively. The electrochemical tests were focused on the WS2-coated active surface of the printed electrodes. The electrochemical performance calculations such as capacitances, energy density, and power density have been determined using equations (S1-S5).
Results and discussion
The cost of producing energy storage units is a significant contributor to the overall cost of electronic devices. 3D printing has emerged as a low-cost and appealing option for prototyping, but a major challenge is the reduced electrochemical activity of 3D-printed electrodes due to high levels of polymer. The fabrication route of the WS2/3D-PCE symmetric supercapacitor is illustrated in . In this study, we addressed this issue by treating the 3D-printed electrodes in a NaOH solution and through electrochemical activation. The activation of the electrodes removed the surface PLA without disturbing the original form, as shown in the insight image of a. Along with the PLA removal, the overall mass of the electrode is reduced by ∼20%.
Figure 1. Schematic diagrams of fabrication of electrochemically coated WS2 modified 3D-printed carbon electrode for fabrication of symmetric supercapacitors.
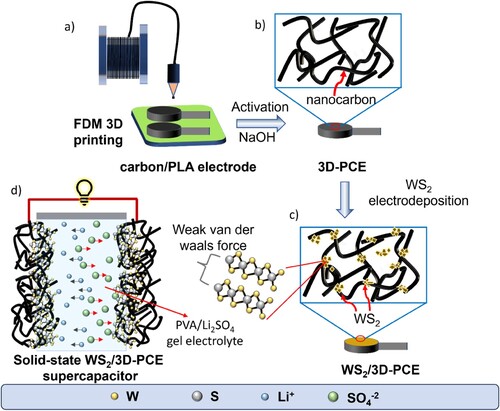
Figure 2. Scanning electron microscopy (SEM) image of (a, b) blank 3D-PCE (inset: digital image of the electrode after activation; scale 5 mm), (c, d) WS2/3D-PCE, (e) SEM-energy dispersive X-ray (EDX) spectroscopy mapping area and (f–j) elemental mapping of W, S, O, C and overlay respectively of WS2/3D-PCE, respectively.
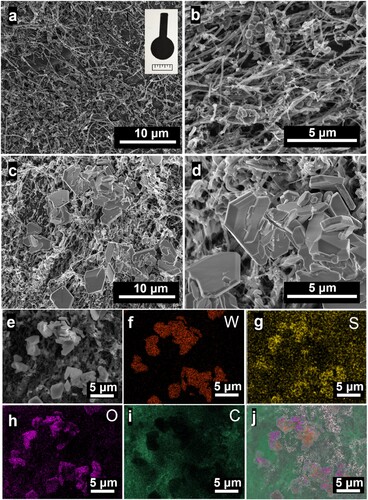
The SEM images of the activated electrode, as depicted in (a,b) reveals the morphology of carbon in the 3D-PCE, which has diameters of range from 70 to 130 nm, with lengths ranging between a few micrometres. The electrochemical deposition of WS2 deposition was carried out by following the previous optimisation published by our group [Citation42]. The corresponding CV of WS2 deposition is depicted in Figure S2b. Morphological analysis of 50 cycles of WS2 deposited 3D-PCE is shown in . After electrodeposition, WS2 flakes were observed around the nanocarbon fiber, as shown in SEM image (c,d). The elemental composition of the electrodeposited WS2 electrode was acquired from EDX spectroscopy analysis. The atomic percentage of the elements was attained from the EDX spectra point analysis shown in Figure S1. The atomic ratio of W and S was measured using EDX point analysis and observed to be ∼1:2. Furthermore, trace amounts of Ti and O were also observed in the WS2/3D-PCE. Ti is obtained from the intrinsic impurity of TiO2 in the nanocarbon filament [Citation29, Citation44]. The WS2 deposition on the nanocarbon electrode is endorsed from the EDX mapping of W and S, as shown in (f and g), respectively. (h–j) depict the mapping of EDX mapping O, C and overlay of individual elements on 3D-PCE, respectively.
Furthermore, high-resolution XPS analysis of the electrodes was conducted to confirm the bonding environment and chemical composition. For in-depth analysis of the bonding state, high-resolution XPS spectra of individual elements were carried out. Deconvolution of W 4f shows the peaks at 31.80 and 33.92 eV corresponding to the W4+ 4f7/2 and W4+ 4f5/2, respectively as shown in (a) [Citation45]. Besides, the peaks at 33.50 and 35.70 eV correspond to the W6+ 4f7/2 and W6+ 4f5/2, respectively. The deconvoluted peak at 37.60 eV is attributed to the W 3p3/2 [Citation46]. The deconvoluted high-resolution spectrum of S 2p is shown in (b). The peaks obtained at 163.64 and 164.80 eV have a spin–orbit peak separation of 1.16 eV attributed to S2- 2p3/2 and S2- 2p1/2, respectively. From the deconvoluted O 1s spectra, four peaks were obtained at 526.9, 528.4, 530.2, 531.4 and 533.3 eV (Figure S4). The peak at 528.4 and 530.2 eV corresponds to the W–O and W = O bonds, respectively due to the lattice oxygen [Citation47]. Other peaks are attributed to the C = O, C–OH and Ti–O bonds which are originated from the 3D-PCE [Citation48–51]. The oxidation states of W (W4+ and W6+), S and O support the formation of WS2 along with small quantity of WO3.
Figure 3. X-ray photoelectron spectroscopy (XPS). High-resolution core-level spectra of (a) W 4f and (b) S 2p of WS2/3D-PCE.
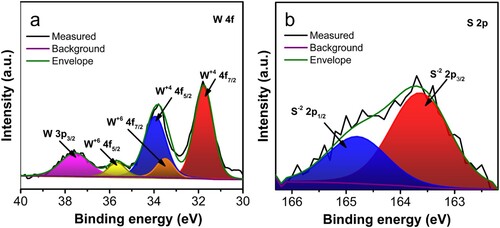
Electrochemical measurements were performed in 1 M Li2SO4 electrolyte in a three-electrode setup to explore the electrochemical behaviour of the 3D-PCE and WS2/3D-PCE. The electrodes required no additional current collector for electrochemical testing. The bare electrode exhibits strong current–potential responsiveness, with increasing scan rate scan speeds from 10 to 100 mV s−1, increasing current density as depicted in Figure S2a. The comparison of CV curves between 3D-PCE and WS2/3D-PCE at a scan rate of 50 mV s−1 reveals the difference in current density after the deposition of WS2, as presented in (a). The current–voltage area at 50 mV s−1, which is connected to the stored charge, increases after WS2 deposition compared to 3D-PCE. (b) shows that the specific capacitance increased by a factor of 2.8 times for the WS2/3D-PCE at the scan rate of 50 mV s−1. As given in (c), the CV was performed for WS2/3D-PCE in the potential window from −0.2 to +0.8 V at scan rates from 10 to 100 mV s−1. The CV patterns correspond to the Type A (pseudocapacitive) behaviour classification within the categorisation of SC electrodes [Citation52, Citation53]. The charge–discharge kinetics (diffusion-controlled and capacitive process) can be elucidated from the CV using the power law equation, i.e. [Citation54–58].
(1)
(1) where, i denotes peak current, v represents scan rate, a is a constant and b is the signifies power-law exponent, which varies in the range of 0.5–1. When b = 0.5, it defines the kinetics is governed by diffusion-controlled pseudocapacitive process, while b = 1 states that the kinetics is dominated by surface-controlled capacitive process. For convenience, EquationEquation (1)
(1)
(1) is rearranged to EquationEquation (2)
(2)
(2) , here the value of b is determined by the slope of log i against log v.
(2)
(2) The plot of log i against log ν for WS2/3D-PCE is depicted in Figure S3. The value of b determined from the slope of the graph is 0.74. This shows that the reaction kinetics of WS2/3D-PCE is not constrained to the surface of the electrodes but also influenced by the diffusion process. According to the CV investigations of the 3D-PCE and WS2/3D-PCE, the WS2 coating significantly boosts the current density. The fast faradic redox reaction occurs at the electrode surface by the Li+ adsorption and desorption is stated by EquationEquation 3
(3)
(3) [Citation59, Citation60].
(3)
(3) Furthermore, the GCD analysis of WS2/3D-PCE was performed at the potential window of −0.2 to +0.8 V at different current densities, as shown in (d). The charge–discharge curve exhibits similar behaviour, consistent with the CV results. At constant current densities of 0.178, 0.255 and 0.764 mA cm−2, the electrode exhibits specific capacitances of 30.62, 6.40, and 4.58 mF cm−2, respectively. The capacitance was increased with a decrease in current density. This is due to the sluggish ion adsorption–desorption process at the lower current density, which allows for greater access to the active region of the WS2/3D-PCE and boosts capacitance [Citation61, Citation62]. At higher current densities, however, the diffusion and migration of electrolyte ions are restricted. While the presence of inherent metal oxide impurity in Blackmagic® filament can contribute to the electrochemical performance of 3D-PCE [Citation24, Citation63, Citation64]. This influence of TiO2 impurity is present in both 3D-PCE and WS2/3D-PCE which evens out. So, the performance enhancement after WS2 deposition can be solely attributed to the presence of WS2.
Figure 4. Electrochemical study in the three-electrode system (a) comparison of WS2/3D-PCE and 3D-PCE at 50 mV s−1, (b) specific capacitance comparison, (c) cyclic voltammogram of WS2/3D-PCE at different scan rates, (d) galvanostatic charge-discharge curves of WS2/3D-PCE at different current densities 0.178, 0.255 and 0.764 mA cm−2 which is labelled as I, II, and III, respectively.
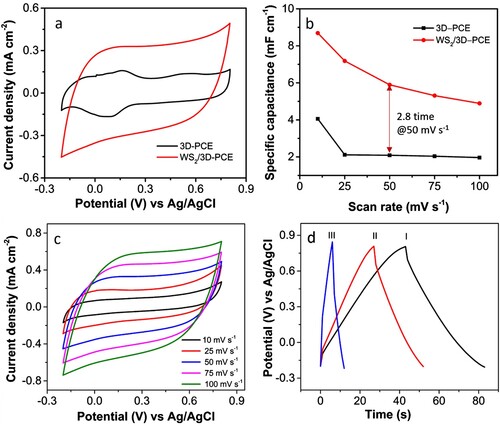
The improvement in charge storage properties of WS2/3D-PCE can be correlated with the conductivity and electrochemical reactivity. EIS was carried out at 0.3 V in the frequency range from 100 kHz to 10 mHz. From the Nyquist plots (Figure S5a) of 3D-PCE and WS2/3D-PCE it is observed that upon WS2 deposition equivalent series resistance (Rs) has decreased from 76 to 49 Ω. The Rs consists of the inherent resistance of the electrode material, solution resistance, interfacial resistance between electrode and electrolyte, and electrode and current collector. The decrease of Rs after WS2 deposition on 3D-PCE can be attributed to the 2D structure and inherent semiconductor nature of WS2 which lowered the inherent resistance of WS2/3D-PCE [Citation65]. Consequently, it is leading to faster electron and ion transfer mobility that improves the overall charge/discharge kinetics.
Considering these encouraging results of the WS2/3D-PCE electrode, a free-standing SS-SC cell is designed with two similar WS2/3D-PCEs and PVA/Li2SO4 gel electrolytes, as described in the experimental section. It is important to note that no external current collector was employed in the fabrication of the SS-SC cell. CV and GCD were measured to assess the electrochemical performance of the SS-SC cell. (a) shows the CV at various scan rates ranging from 0 to 1 V. The current density raises with the increase of scan rate from 10 to 100 mV s−1, demonstrating the good capacitive behaviour by the solid-state device.
Figure 5. Electrochemical study in two electrode system. (a) Cyclic voltammogram of WS2/3D-PCE solid-state symmetric supercapacitor at different scan rates (b) galvanostatic charge-discharge (GCD) curves of WS2/3D-PCE solid-state symmetric supercapacitor at different current densities 56.62, 169.87, and 283.09 µA cm−2, denoted as I, II, and III, respectively. GCD curves of (c) series and (d) parallel connection of two and three-cells at the current density of 0.11 mA cm−2, respectively.
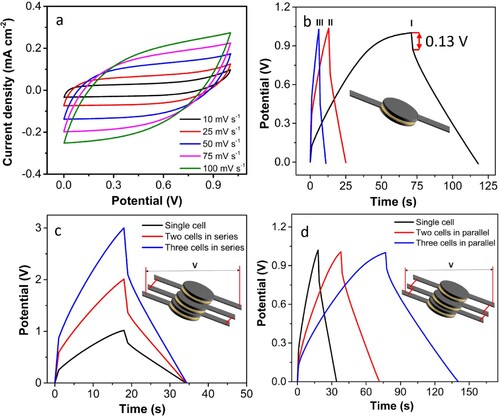
(b) depicts the GCD study at various current densities. The GCD curves show a symmetrical charge–discharge profile is observed. From the discharge curve, an IR drop of 0.13 V is found at the current density of 56.62 µA cm−2. The SS-SC exhibits the areal capacitances of 2.66, 2.04, and 1.42 mF cm−2 and areal energy density of 0.37, 0.28, and 0.20 µWh cm−2 and areal power density 28.31, 84.94, and 141.56 µW cm−2 at the current densities of 56.62, 169.87, and 283.09 µA cm−2, respectively. The cyclic stability of the cell is an important evaluating factor in the lifetime assessment of supercapacitors [Citation66]. The cyclic stability test was carried out at 226.50 µA cm−2 that revealed excellent capacitance retention of 98% of its initial capacitance of 1.12 mF cm−2 even after 40,000 charge–discharge cycles (Figure S6).
In real-world applications, a single supercapacitor cannot provide all the energy and power required. To determine if multiple supercapacitors can meet practical requirements, the supercapacitors are connected either in series or in parallel. (c, d) depicts that two and three cells are connected in parallel and series, respectively. The two and three series-connected cells attain potential difference of 2 and 3 V, respectively at the current density of 56.62 µA cm−2. Thus, the series connection of supercapacitors is an effective method to provide the energy demand for higher output voltage. These series-connected cells can deliver enough potential difference to power LEDs and small portable devices. Additionally, a parallel connection of three supercapacitors showed three times longer output than a single supercapacitor cell operating at the same current density. The 3D printed structure facilitates easy assembling of the series and parallel connected cells in the same foot-print area as shown in the inset of (c,d). Thus, the serial and parallel-connected 3D-printed cells can be used for obtaining the desired operating voltage and current.
Conclusion
In this work, an easy and scalable manufacturing of free-standing electrodes of WS2 electrodeposited 3D-printed carbon electrodes are fabricated for supercapacitor application. The WS2 deposition on a 3D-printed carbon electrode improves the capacitive performance of 3D-printed carbon electrode by several folds. With the customised electrode, solid-state supercapacitor cells can be prepared without the need for an additional current collector or separator, which results in higher capacitive performance. Towards future direction, various electrochemically active transition metal dichalcogenides can be deposited onto the surface of the 3D-printed carbon electrode, allowing for the easy production of supercapacitor cells of any desired shape to meet the growing demand in the modern electronics market.
Conflicts of interest
There are no conflicts to declare.
Authors contribution
S. M. carried out materials synthesis and fabrication, characterisations, and analysis. K. G. conceptualised the project and mentored in fabrications, characterisations and analysing data. S. M. wrote the first draft and K. G reviewed and edited. M. P. supervised the project. All authors contributed to the writing.
Supplemental Material
Download MS Word (3.1 MB)Acknowledgements
The work was supported by ERDF/ESF project TECHSCALE (No. CZ.02.01.01/00/22_008/0004587). This research was co-funded by the European Union under the REFRESH – Research Excellence For REgion Sustainability and High-tech Industries project number CZ.10.03.01/00/22_003/0000048 via the Operational Programme Just Transition. CzechNanoLab project LM2018110 funded by MEYS CR is gratefully acknowledged for the financial support of the measurements/sample fabrication at CEITEC Nano Research Infrastructure.
Disclosure statement
No potential conflict of interest was reported by the author(s).
Data availability statement
The data that support the findings of this study are available from the corresponding author, M. P., upon reasonable request.
References
- Tan HW, Choong YYC, Kuo CN, et al. 3D printed electronics: processes, materials and future trends. Prog Mater Sci. 2022;127:1–28.
- Jandyal A, Chaturvedi I, Wazir I, et al. 3D printing – a review of processes, materials and applications in industry 4.0. Sustainable Operat Comput. 2022;3:33–42.
- Shahrubudin N, Lee TC, Ramlan R. An overview on 3D printing technology: technological, materials, and applications. Procedia Manuf. 2019;35:1286–1296.
- Zhang S, Liu Y, Hao J, et al. 3D-printed wearable electrochemical energy devices. Adv Funct Mater. 2022;32:1–33.
- Kwon S, Kim H, Yeo WH. Recent advances in wearable sensors and portable electronics for sleep monitoring. iScience. 2021;24(5):1–16.
- Sumboja A, Liu J, Zheng WG, et al. Electrochemical energy storage devices for wearable technology: a rationale for materials selection and cell design. Chem Soc Rev. 2018;47:5919–5945.
- Kasprzak D, Mayorga-Martinez CC, Pumera M. Sustainable and flexible energy storage devices: a review. Energy Fuels. 2023;37(1):74–97.
- Zhou R, Wang H, Chang J, et al. Ammonium intercalation induced expanded 1T-rich molybdenum diselenides for improved lithium ion storage. ACS Appl Mater Interfaces. 2021;13(15):17459–17466.
- Zhai Z, Zhang L, Du T, et al. A review of carbon materials for supercapacitors. Mater Des. 2022;221:1–19.
- Chatterjee DP, Nandi AK. A review on the recent advances in hybrid supercapacitors. J Mater Chem A. 2021;9:15880–15918.
- Yao Z, Yu C, Dai H, et al. Hybrid fibers assembled from MoSe2/graphene heterostructures endow improved supercapacitive performance. Carbon N Y. 2022;187:165–172.
- Zhu C, Liu T, Qian F, et al. 3D printed functional nanomaterials for electrochemical energy storage. Nano Today. 2017;15:107–120.
- Wei M, Zhang F, Wang W, et al. 3D direct writing fabrication of electrodes for electrochemical storage devices. J Power Sources. 2017;354:134–147.
- Jha S, Velhal M, Stewart W, et al. Additively manufactured electrodes for supercapacitors: a review. Appl. Mater. Today. 2022;26:1–26.
- Gulzar U, Glynn C, O’Dwyer C. Additive manufacturing for energy storage: methods, designs and material selection for customizable 3D printed batteries and supercapacitors. Curr Opin Electrochem. 2020;20:46–53.
- Liu Y, Zheng S, Ma J, et al. Aqueous high-voltage all 3D-printed micro-supercapacitors with ultrahigh areal capacitance and energy density. J Energy Chem. 2021;63:514–520.
- Zong W, Ouyang Y, Miao YE, et al. Recent advances and perspectives of 3D printed micro-supercapacitors: from design to smart integrated devices. Chem Comm. 2022;58(13):2075–2095.
- Huang Q, Liu X, Wang J. 3D printed pure carbon-based electrodes for zinc-ion hybrid supercapacitor. Carbon Trends. 2022;9:1–9.
- Gao H, An J, Chua CK, et al. 3D printed optics and photonics: processes, materials and applications. Materials Today. 2023;69:107–132.
- Browne MP, Redondo E, Pumera M. 3D printing for electrochemical energy applications. Chem Rev. 2020;120(5):2783–2810.
- Muñoz J, Pumera M. Accounts in 3D-printed electrochemical sensors: towards monitoring of environmental pollutants. ChemElectroChem. 2020;7:3404–3413.
- Redondo E, Muñoz J, Pumera M. Green activation using reducing agents of carbon-based 3D printed electrodes: turning good electrodes to great. Carbon N Y. 2021;175:413–419.
- Foster CW, Down MP, Zhang Y, et al. 3D printed graphene based energy storage devices. Sci Rep. 2017;7:1–11.
- Browne PM, Novotný F, Sofer Z, et al. 3D printed graphene electrodes’ electrochemical activation. ACS Appl Mater Interfaces. 2018;10(46):40294–40301.
- Iffelsberger C, Ng S, Pumera M. Catalyst coating of 3D printed structures via electrochemical deposition: case of the transition metal chalcogenide MoSx for hydrogen evolution reaction. Appl Mater Today. 2020;20:1–7.
- Manzanares L, Palenzuela C, Novotný F, et al. 3D-printed graphene/polylactic acid electrodes promise high sensitivity in electroanalysis. Anal Chem. 2018;90(9):5753–5757.
- Gusmão R, Browne MP, Sofer Z, et al. The capacitance and electron transfer of 3D-printed graphene electrodes are dramatically influenced by the type of solvent used for pre-treatment. Electrochem Commun. 2019;102:83–88.
- Novotný F, Urbanová V, Plutnar J, et al. Preserving fine structure details and dramatically enhancing electron transfer rates in graphene 3D-printed electrodes via thermal annealing: toward nitroaromatic explosives sensing. ACS Appl Mater Interfaces. 2019;11(38):35371–35375.
- Ghosh K, Pumera M. Free-standing electrochemically coated MoSx based 3D-printed nanocarbon electrode for solid-state supercapacitor application †. Nanoscale. 2021;13:5744–5756.
- Ghosh K, Pumera M. MXene and MoS3−x coated 3D-printed hybrid electrode for solid-state asymmetric supercapacitor. Small Methods. 2021;5(8):1–15.
- Ng S, Zazpe R, Rodriguez-Pereira J, et al. Atomic layer deposition of photoelectrocatalytic material on 3D-printed nanocarbon structures. J Mater Chem A. 2021;9(18):11405–11414.
- Gao Y, Ding J. 3D printed thick reduced graphene oxide: manganese oxide/carbon nanotube hybrid electrode with highly ordered microstructures for supercapacitors. Adv Mater Technol. 2023;8:1–11.
- Mohan VV, Manuraj M, Anjana PM, et al. WS2 nanoflowers as efficient electrode materials for supercapacitors. Energy Technol. 2022;10(3):1–7.
- Sengupta S, Kundu M. Self-Assembled 2D WS2 interconnected nanosheets: an anode material with outstanding lithium-storage performance. Energy Technol. 2022;10(6):1–9.
- Dai H, Zhao Y, Zhang Z, et al. Ostwald ripening and sulfur escaping enabled chrysanthemum-like architectures composed of NiS2/NiS@C heterostructured petals with enhanced charge storage capacity and rate capability. J Electroanal Chem. 2022;921:1–7.
- Liu QY, Sun GW, Pan JL, et al. Metal ion cutting-assisted synthesis of defect-rich MoS2 nanosheets for high-rate and ultrastable Li2S catalytic deposition. ACS Appl Mater Interfaces. 2022;14(33):37771–37781.
- Mohan VV, Manuraj M, Anjana PM, et al. WS2 nanoflowers as efficient electrode materials for supercapacitors. Energy Technol. 2022;10(3):1–7.
- Wu C, Zeng X, He P, et al. Flexible WS2@CNFs membrane electrode with outstanding lithium storage performance derived from capacitive behavior. Adv Mater Interfaces. 2018;5(3):1–8.
- Venkata Guru Raghavendra K, Sreekanth TVM, Kim J, et al. Novel hydrothermal synthesis of jasmine petal-like nanoflower WS2/ZnCo2O4 as efficient electrode material for high-performance supercapacitors. Mater Lett. 2021;285:1–5.
- Kim JY, Chae S, Jang W, et al. Antioxidant triggered metallic 1T’ phase transformations of chemically exfoliated tungsten disulfide (WS2) nanosheets. Small. 2022;18(12):1–9.
- Lan C, Li C, Ho JC, et al. 2D WS2: from vapor phase synthesis to device applications. Adv Electron Mater. 2021;7:1–36.
- Tan SM, Pumera M. Bottom-up electrosynthesis of highly active tungsten sulfide (WS3-x) films for hydrogen evolution. ACS Appl Mater Interfaces. 2016;8(6):3948–3957.
- Wu Z-S, Winter A, Chen L, et al. Three-dimensional nitrogen and boron Co-doped graphene for high-performance all-solid-state supercapacitors. Adv Mater 2012;24:5130–5135.
- Ghosh K, Ng S, Iffelsberger C, et al. Inherent impurities in graphene/polylactic acid filament strongly influence on the capacitive performance of 3D-printed electrode. Chem Eur J. 2020;26(67):15746–15753.
- Komornicki S, Radecka M, Sobaś P. Structural properties of TiO2-WO3 thin films prepared by r.f. sputtering. J Mater Sci Mater Electron. 2004;15(8):527–531.
- Liu L, Ye K, Lin C, et al. Grain-boundary-rich polycrystalline monolayer WS2 film for attomolar-level Hg2+ sensors. Nat. Commun. 2021;12(1):1–8.
- Gao G, Lv M, Shao Y, et al. Crystal facet-dependent activity of h-WO3 for selective conversion of furfuryl alcohol to ethyl levulinate. Phys Chem Chem Phys 2020;22:26923–26934.
- Lu Y, Huang B, Xu X, et al. Construction and characteristics of a novel green photocatalyst:iron (III) doped titania nanomesh. Ceram Int. 2021;47(16):23497–23506.
- Shao Y, Jin X, Li C, et al. An effective non-equivalent ion exchange method for building an advanced Z-scheme WO3/Bi2WO6 photocatalyst. New J. Chem. 2021;45(46):21863–21868.
- Pacholik G, Enzlberger L, Benzer A, et al. In situ XPS studies of MoS2-based CO2 hydrogenation catalysts. J. Phys. D: Appl. Phys. 2021;54(32):1–10.
- Wang H, Wei L, Shen J. Metal-free catalyst for efficient PH-universal oxygen reduction electrocatalysis in microbial fuel cell. J Electroanal. Chem. 2022;911:1–8.
- Swaminathan R, Pazhamalai P, Mohan V, et al. Topochemically prepared tungsten disulfide nanostructures as a novel pseudocapacitive electrode for high performance supercapacitor. J Colloid Interface Sci. 2023;652:845–855.
- Gogotsi Y, Penner RM. Energy storage in nanomaterials – capacitive, pseudocapacitive, or battery-like? ACS Nano. 2018;12(3):2081–2083.
- Lindstro H, So S, Solbrand A, et al. Li+ ion insertion in TiO2 (anatase). 2. Voltammetry on nanoporous films. J Phys Chem B. 1997;101, 39:7717–7722.
- Augustyn V, Come J, Lowe MA, et al. High-rate electrochemical energy storage through Li+ intercalation pseudocapacitance. Nat Mater. 2013;12(6):518–522.
- Palacios-Corella M, Ghosh K, Redondo E, et al. Polyoxometalate-enhanced 3D-printed supercapacitors. ChemSusChem. 2022;15(23):1–8.
- Alhabeb M, Maleski K, Anasori B, et al. Guidelines for synthesis and processing of two-dimensional titanium carbide (Ti3C2Tx MXene). Chem Mater. 2017;29(18):7633–7644.
- Mathis TS, Kurra N, Wang X, et al. Energy storage data reporting in perspective—guidelines for interpreting the performance of electrochemical energy storage systems. Adv Energy Mater. 2019;9:1–13.
- Mohan M, Shetti NP, Aminabhavi TM. Phase dependent performance of MoS2 for supercapacitor applications. J. Energy Storage. 2023;58:1–19.
- Pan Z, Li X, Yang C, et al. One-step construction of Ti3C2Tx/MoS2 hierarchical 3D porous heterostructure for ultrahigh-rate supercapacitor. J. Colloid Interface Sci. 2023;634:460–468.
- Ghosh K, Ng S, Iffelsberger C, et al. Inherent impurities in graphene/polylactic acid filament strongly influence on the capacitive performance of 3D-printed electrode. Chem. Eur. J. 2020;26(67):15746–15753.
- Santo J, Penumakala PK, Adusumalli RB. Mechanical and electrical properties of three-dimensional printed polylactic acid–graphene–carbon nanofiber composites. Polym. Compos. 2021;42(7):3231–3242.
- Mastria R, Scarfiello R, Altamura D, et al. In-plane aligned colloidal 2D WS2 nanoflakes for solution-processable thin films with high planar conductivity. Sci Rep. 2019;9(1):1–13.
- Zhang, S., Pan, N. Supercapacitors Performance Evaluation. Adv. Energy Mater. 2015;5:1–19.
- Alkhalaf S, Ranaweera CK, Kahol PK, et al. Electrochemical energy storage performance of electrospun CoMn2O4 nanofibers. J. Alloys Compd. 2017;692:59–66.
- Senthilkumar ST, Selvan RK, Lee YS, et al. Electric double layer capacitor and its improved specific capacitance using redox additive electrolyte. J. Mater. Chem. A Mater. 2013;1(4):1086–1095.