Abstract
Background Old rats require more time for bone to bridge a fracture gap than young rats. To explore possible mitochondrial dysfunction in this delay, we measured levels of mRNA derived from mitochondrial genes in healing fractures of young, adult, and old rats.
Methods Diaphyseal femoral fractures were induced in female rats at 6, 26, and 52 weeks of age (young, adult, and old rats, respectively). At baseline, at 3 days, and 1, 2, 4, and 6 weeks after fracture, the fracture site was harvested. Total RNA was extracted, and cRNA was prepared and hybridized to 54 Affymetrix U34A microarrays (2 rats/array and 3 arrays/age/time point).
Results Radiographic union occurred progressively later with age. Of the 107 mitochondria-related genes reviewed, all 8 located within the mitochondrial DNA and some nuclear genes (of the electron transport chain and tricarboxylic acid cycle) showed a prolonged reduction in gene expression after fracture in the oldest rats, to about half the level of expression detected in young rats at 6 weeks after fracture. The transcript levels of apoptotic genes increased after fracture in rats of all three ages, while the transcript levels of 23 mitochondriarelated genes were largely unaltered after fracture.
Interpretation Delayed fracture healing in old rats is associated with reduced mRNA expression of genes forming the mitochondrial energy pathways.
Mitochondria are needed for cellular energy production (Cholley et al. Citation2001). There are high numbers of mitochondria in the osteoblasts during the synthesis of organic matrix and mineral deposition (Tonna and Pillsbury Citation1959, Tonna Citation1966, Gothlin Citation1973). Aging reduces the functioning of the mitochondria in the osteoblast (Wei and Lee Citation2002), the number of mitochondria per osteoblast (Tonna and Pillsbury Citation1959), and the size of each mitochondrion (Tonna and Pillsbury Citation1959). For adult animals, fracture restores the level of mitochondrial respiratory enzyme function and mitochondrial size and number to those seen in young animals (Tonna Citation1966). For old animals, however, there is no significant stimulation of mitochondrial function after skeletal injury (Tonna Citation1966).
Bridging of the fracture gap with new bone is slower in older rats (Meyer, Jr. et al. Citation2001), older mice (Lu et al. Citation2005), and older humans (reviewed by Meyer, Jr. et al. Citation2003). While young rats, 6 weeks old at fracture, regain normal skeletal mechanics at the fracture site 4 weks after fracture, adult, 26-week-old rats require 10 weeks, and old, 52-week-old rats require more than 6 months (Meyer, Jr. et al. Citation2001). The cause for this delay in bone repair has not been clear (Meyer, Jr. et al. Citation2003, Desai et al. Citation2003), and this led us to search for genes with abnormal levels of mRNA expression following fracture in old rats.
DNA microarray technology measures mRNA expression levels of large numbers of genes in the same assay. We have employed this methodology to assess gene expression at the mRNA level in healing fractures at various times after surgery in young, adult, and old rats (Meyer, Jr. et al. Citation2006). We hypothesized that there would be abnormal mRNA expression of mitochondrial genes in the fracture callus of older rats.
Material and methods
Rats
This project has been described in detail previously (Meyer et al. Citation2004), and the present report is an analysis of genes related to mitochondrial function. Briefly, at 1 or 6 months of age, intact female Sprague-Dawley rats were obtained from Harlan Sprague-Dawley, Inc. (Indianapolis, IN). Our vivarium has been accredited by the Association for Assessment and Accreditation of Laboratory Animal Care International, and the experimental protocol was approved by the Institutional Animal Care and Use Committee.
Surgery
Female rats at 6 (young), 26 (adult) or 52 (old) weeks of age were anesthetized, and an intramedullary rod was placed retrograde in the left femur as described previously (Meyer, Jr. et al. Citation2001). A closed simple transverse mid-diaphyseal femoral fracture was induced with a Bonnarens and Einhorn (Citation1984) device. For the zero-time controls, intact rats were randomly selected from those scheduled for surgery. Radiographs were taken at surgery, 1 week after surgery, and at killing. Rats of each of the 3 ages were killed at 6 different time points (0, 0.4 (3 days), 1, 2, 4, and 6 weeks after fracture) and the femora were rapidly removed. One-third of the length of the femur, centered on the fracture site, was collected. This was composed of the fracture callus with associated cortical bone and marrow. The tissue was frozen in liquid nitrogen and kept at –75°C.
Preparation of RNA samples and microarray processing
The Affymetrix GeneChip Expression Analysis Technical Manual (701021, rev. 1; Affymetrix, Santa Clara, CA) was followed for sample preparation. The method has been reported (Meyer et al. Citation2004) and is described here in brief. TRIzol (Invitrogen, Carlsbad, CA) was used to extract total RNA from the tissue following disruption with a Brinkmann Polytron homogenizer. Each microarray sample was a pool of RNA from 2 rats of the same age and time point, to reduce the variability caused by interanimal differences. Samples with 15 μg RNA were reverse transcribed to double-stranded cDNA with a Superscript Double-Stranded cDNA Synthesis Kit (11917-010; Invitrogen). Biotinlabeled cRNA was then formed by in vitro transcription (IVT) with the Enzo RNA Transcript Labeling Kit (900182; Affymetrix). Non-enzy-matic fragmentation of the biotin-labeled cRNA was done. BioB, bioC, bioD, and cre (900299; Affymetrix) were added to each sample. The fragmented cRNA was hybridized to Rat U34A microarrays (900249; Affymetrix). Fluorescent labels were attached to the biotin. The Agilent GeneArray Scanner (G2500A; Agilent Technologies, Palo Alto, CA) was used to scan each array twice. For each time point and each age, 3 replicate samples (from 6 rats) were tested on separate arrays; thus, 54 arrays were used in total.
Data analysis
Mitochondria-related genes were identified from among the 8,700 genes on the Rat U34A GeneChip Microarray by searching the NetAffx database (http://www.affymetrix.com) for the Gene Descriptor “mitochon”. This search revealed 107 mito-chondria-related transcripts that formed the basis of this report. GenBank accession numbers and the most recent gene names are shown in the Figures and Tables for each gene. If the gene name referred to the sequence of a segment of DNA containing more than one gene, the name of the gene specific to the probe set on the array was employed.
Affymetrix Microarray Suite 5.0 was used for quality control and to scale the mRNA expression (signal value) of all genes to an average of 500 for each array. Based on the signal values of each probe set and the background fluorescence, Microarray Suite 5.0 categorized each gene as Present, Marginal, or Absent. The Present/Absent transition for most genes was at about 100 units of signal value. Each bar in each graph shows the average signal (± SEM) for 3 microarrays that were done for each age at each time point. Statistical comparisons between the mean values of the various treatment groups were done with Student's t test (Zar Citation1984). Since the response to fracture for most transcripts occurred over several time points, the data from these time points were pooled prior to testing to increase the statistical power.
The Minimum Information About a Microarray Experiment (MIAME) standards of the Microarray Gene Expression Data (MGED) Society (http://www.mged.org) were followed in this report. The full microarray data set for all genes with CEL files can be found in the NCBI Gene Expression Omnibus (http://www.ncbi.nlm.nih.gov/geo) as series GSE594. Gene expression levels for all genes are also shown in tabular format in an on-line appendix (Meyer, Jr. et al. Citation2006). The full dataset with the current names of the 107 genes in this study is shown in an on-line supplementary information.
Results
The radiographic data have been described in detail previously (Desai et al. Citation2003, Meyer et al. Citation2004). In summary, the young rats—6 weeks old at frac-ture—formed bone to bridge the fracture gap by 4 weeks after fracture. The adult rats—26 weeks old at fracture—had a vigorous periosteal reaction 6 weeks after fracture and reached radiographic union 8–10 weeks after fracture. In contrast, the old rats—52 weeks old at fracture—had minimal periosteal reactions 6 weeks after fracture and did not reach radiographic union during the time course of this experiment.
107 transcripts from genes associated with mitochondrial function were identified as having probe sets on the Rat U34A GeneChip microarray and these formed the dataset studied in this report. 84 of these transcripts had altered mRNA expression after fracture. 5 patterns were detected for these genes:
1. All 8 genes located within the mitochondrial DNA and 23 nuclear genes related to mitochondrial function showed a moderate decrease in gene expression after fracture (3 examples are shown in ). The transcript levels for the 3 genes in showed a decrease at 0.4 weeks after fracture compared to the zero-time, no-fracture samples, which was highly significant for each gene (p < 0.001). There was recovery to baseline activity by 6 weeks after fracture in the young rats. No recovery occurred in the old rats, however. For the 3 genes shown in , the oldest rats had their gene expression depressed to about half the level of young rats at 4 and 6 weeks after fracture (p < 0.001 (M35826, ), p < 0.001 (J01436, ), and p = 0.01 (U68544, )). The first 2 genes ( and ) are located in the mitochondrial DNA. Data for all 31 of these genes are shown in Table 1 of the on-line supplementary information.
Figure 1. mRNA levels of 3 genes from mitochondrial (A and B) and nuclear (C) DNA with decreased expression after fracture and significantly slower recovery in old rats. Samples were fracture calluses collected from female Sprague-Dawley rats at 6 (young), 26 (adult), or 52 (old) weeks of age at femoral fracture. Samples were harvested at the indicated number of weeks after fracture. Each bar represents the average signal ± SEM of three arrays. The ordinate is mRNA concentration measured in units of fluorescence. Note that expression decreased for each gene after fracture (p < 0.001), and recovered faster in the young rats than in the old rats. Asterisks denote significant differences between young and old rats at 4 and 6 weeks after fracture, at p < 0.05 (*), p < 0.01 (**), or p < 0.001 (***).
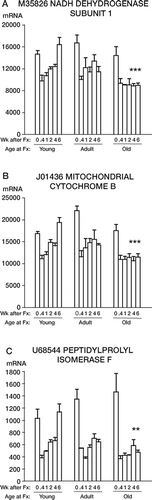
2. Ten nuclear genes for mitochondrial enzymes showed a variation on this first pattern. In the old rats, there was lower activity in the unfractured bone at the zero time point (). For these genes, there was no change in mRNA expression after fracture in the old rats. The young rats again had higher expression of these genes 4 and 6 weeks after fracture compared to the old rats (p = 0.002 (K00750, ) and p = 0.04 (U75393, )). The data for the genes in this category are shown in Table 2 of the on-line supplementary information.
Figure 2. mRNA levels of 2 mitochondrial genes whose expression was depressed in old rats. Note that the old rats had reduced RNA levels in unfractured bone, and were minimally affected by fracture. In contrast, the young rats had a higher level of RNA expression initially, which decreased transiently after fracture. The data are presented as in .
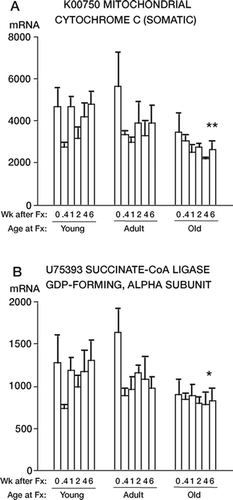
3. In the third pattern, for all three ages fracture caused a decrease in mitochondrial gene activity for 25 nuclear-encoded genes (). Some genes in this group had a profound drop in mRNA expression after fracture (the decreases for all 3 genes in were significant; p < 0.001). While there was subsequent recovery to baseline activity in rats of all 3 ages, the recovery in expression of most of these genes was slower in the old rats. Gene expression at 1 and 2 weeks after fracture was less in the old rats than in the young rats (p = 0.03 for both X59736 () and D90109 ()). The most severe drop in mRNA levels after fracture was seen in genes for the electron transport chain, with smaller changes in the genes for other functions. These data are shown in full in Table 3 of the on-line supplementary information.
Figure 3. mRNA levels of genes for 3 mitochondrial . mRNA levels for 3 genes related to mitochondrial enzymes; mRNA expression was profoundly depressed activity that are upregulated by fracture (p < 0.001 at 0.4 3 days after skeletal fracture. Note also the significantly weeks compared to 0 weeks). The data are presented as slower recovery in the old rats for the genes shown in in . panels A and B. The data are presented as in , except that the asterisks refer to comparisons between young and old rats at 1 and 2 weeks after fracture.
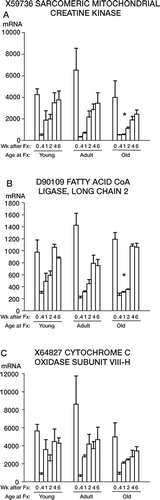
4. Eighteen transcripts were found for which expression levels were increased by fracture (3 are shown in ). For the examples shown in , the increase at 0.4 weeks over the nofracture value was significant at p < 0.001 for all genes. Data for these 18 genes are shown in Table 4 of the on-line supplementary information.
Figure 4. mRNA levels for 3 genes related to mitochondrial activity that are upregulated by fracture (p < 0.001 at 0.4 weeks compared to 0 weeks). The data are presented as in .
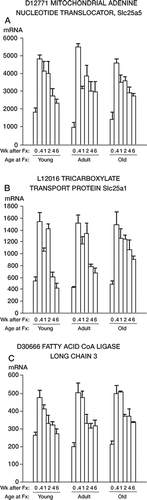
5. There was a group of 23 genes whose mRNA levels did not change markedly after fracture. These genes are shown in Table 5 of the on-line supplementary information.
Discussion
During fracture repair, the genes coding for cartilage, bone matrix proteins and cytokines are upregulated many fold (Desai et al. Citation2003, Meyer, Jr. et al. Citation2003). In contrast, in old rats fracture causes prolonged downregulation of mitochondrial genes that are needed for the electron transport chain ( and ) as well as some genes related to the TCA cycle (). Transcript levels for these genes were suppressed to about half of the expression levels for the young rats at 6 weeks after fracture. While a few genes related to mitochondrial activity were upregulated by fracture (), mRNA for most genes involved in energy production remained downregulated in the old rats during the 6-week study period. It is this persistently lower level of mRNA expression in the old rats, with a reduction of about 50% for most genes compared to young rats, that leads us to hypothesize a role for the mitochondria in the failure of old rats to achieve rapid healing of their femoral fractures.
Functioning mitochondria are needed for energy production and thermogenesis for normal metabolic activity (Cholley et al. Citation2001). Mitochondria are found in large numbers in active osteoblasts. With advancing age, the number of mitochondria per osteoblast becomes reduced, as does the size of each mitochondrion (Tonna and Pillsbury Citation1959). Fracture increases mitochondrial respiratory enzyme function in adult rats and adult mice, and mitochondrial size and number revert to or approach the levels of young animals. However, in an early study old rats and old mice at one year of age or greater failed to show this stimulation of mitochondrial function after skeletal injury (Tonna Citation1966). These morphological and biochemical findings are in agreement with our data for reduced mitochondrial response to fracture in the old rats. We have found changes not only in mRNA levels of genes located in the mitochondrial DNA, but also of genes resident in the nuclear DNA that code for mitochondrial proteins.
Age is accompanied by reduced activity of the mitochondria in most tissues (Wei and Lee Citation2002). This age-related change in the mitochondria is thought to contribute to the pathophysiology of osteoporotic fractures in older men (Varanasi et al. Citation1999), to osteoarthritis (Martin and Buckwalter Citation2002), to insulin resistance in the elderly (Petersen et al. Citation2003), to ischemia-reperfusion injury in the older heart (Lesnefsky and Hoppel Citation2003), reduced oxidative capacity in human muscle (Conley et al. Citation2000) and heart (Rosenfeldt et al. Citation2002), and to the toxicity of 1-methyl-4-phenylpyridine (MPP+) (Desai et al. Citation1996). Mitochondria in the brains of older mice were found to be slower to recover from an insult (Desai et al. Citation1996), as were the mitochondria of bone in the present study. We hypothesize that such age-induced damage to the mitochondria impairs skeletal fracture healing in older rats.
Fracture induced a transient downregulation of the mRNA expression of some mitochondrial genes (, , and ). This may have been a result of disruption of the blood supply to the fracture site, which would limit aerobic metabolism. Also, the initial steps in fracture healing involve the formation of cartilage, with its limited vascular supply. In addition, we have noted the downregulation of mRNA for blood cell formation in the region of the fracture (Meyer, Jr. et al. Citation2006). All three effects would reduce the demands for mitochondrial activity.
The genomic response to skeletal fracture is complex. Of the 8,700 genes on each U34A microarray, an average of 3,300 was scored as present. Of this total, more than two-thirds of the genes had a change in mRNA expression levels following fracture. Most of these genes were not known to participate in the healing process of bone before the use of microarray technology (Hadjiargyrou et al. Citation2002, Meyer, Jr. et al. Citation2006). These changes are caused by both changes in the types of cells at the fracture site and changes in the activity of the existing cells. An earlier study of gene expression following fracture made use of microarrays constructed from genes whose expression increased after fracture. The expression of 21 mitochondrial genes was found to be increased following fracture (Hadjiargyrou et al. Citation2002).
The response of most genes to fracture is not changed by age (Meyer, Jr. et al. Citation2006). In addition to the mitochondrial changes reported in this paper, we have found changes in mRNA gene expression that suggest a slowing of the reinnervation of the fracture site in old rats (Meyer et al. Citation2004). There is also a group of genes whose expression is upregulated to a higher level and/or for a longer time period in the fracture callus of old rats (Meyer and Meyer Citation2007).
Other changes related to age have been found in osteoblast function. There is a reduced response of older osteoblasts to stimulation (Battmann et al. Citation1997). There is also a decrease in the chondrogenic potential of the periosteum with age (O’Driscoll et al. Citation2001), and there is less cellular proliferation with age (Tonna and Cronkite Citation1963, Sekine et al. Citation1995). There are also fewer osteoblast precursors in the bone marrow stem cells (Quarto et al. Citation1995, Bergman et al. Citation1996). It is unclear whether such changes at the cellular and tissue level may be due to the changes that occur in the mitochondria with age.
The expression of genes related to the bone morphogenetic proteins, Indian hedgehog, collagen type II and aggrecan suggests a rapid formation of soft callus at the fracture site of adult rats (Desai et al. Citation2003) and old rats (Meyer, Jr. et al. Citation2003). This soft callus was found to be responsible for the rapid partial restoration of biomechanical properties after fracture in adult and old rats (Meyer, Jr. et al. Citation2001). However, the full restoration of biomechanical stiffness and load to failure, as well as radiographic union, requires the formation of bone to bridge the fracture gap. It is the formation of bone that is delayed in these adult and old rats (Meyer, Jr. et al. Citation2001, Desai et al. Citation2003, Meyer, Jr. et al. Citation2003). Since mitochondria are concentrated at the sites of mineralization in the fracture callus (Gothlin Citation1973, Ketenjian and Arsenis Citation1975), mitochondrial defects would be expected to impair this process.
In this report, we measured messenger RNA levels. It must be kept in mind that protein levels are not necessarily strongly correlated to mRNA levels. Proteins are affected by posttranslational control mechanisms that affect protein levels without a change in mRNA levels, and mRNA may be present without protein synthesis. Certainly, low mRNA levels such as those seen in predict low rates of protein synthesis for those genes with considerable confidence. In situ hybridization studies are now under way to localize the changes noted from the microarray results found in this study.
Typically, microarray projects are bedevilled by false positive responses—since large numbers of genes are being measured. This leads to random changes in the expression of unaffected genes that appear to be responses to treatment. In the present project, the genes selected for review were not defined by apparent response to treatment or by data-mining cluster analysis. Instead, the NetAffx database was searched for all genes on the U34A microarray which were related to the mitochondria, and 107 genes were found. Among this group, only 23 genes were unaffected by fracture. This response to fracture by 78% of the mitochon-dria-related genes under review attests to the idea that there is an important change in mitochondrial function in old rats.
Measurement of mRNA gene expression by RTPCR and by microarray technology gives comparable results for genes with moderate expression levels when the probe sets and PCR primers are in the same region of the gene (Etienne et al. Citation2004). The findings in this report of moderate expression levels, good agreement between the three replicates, and a strong response to fracture give us confidence in the ability of microarray technology to report changes in gene expression for these transcripts.
In conclusion, the slower formation of bone to bridge a fracture gap in adult and old rats is associated with changes in the mRNA expression of genes related to mitochondrial function. Such changes may reflect a reduced energy production that may limit the ability of the bone-forming cells to repair skeletal fractures.
Supplementary information on http://www.actaor-thopscand/downloadables, file Meyer & Meyer: Mitochondrial genes.
We thank Wiguins Etienne and Jessica Heath for their technical assistance and Khristina Bretthauer for her administrative assistance. This project was supported in part by grants from the North Carolina Biotechnology Center and the Orthopaedic Trauma Association.
No competing interests declared.
- Battmann A, Battmann A, Jundt G, Schulz A. Endosteal human bone cells (EBC) show age-related activity in vitro. Exp Clin Endocrinol Diabetes 1997; 105: 98–102
- Bergman R J, Gazit D, Kahn A J, Gruber H, McDougall S, Hahn T J. Age-related changes in osteogenic stem cells in mice. J Bone Miner Res 1996; 11: 568–77
- Bonnarens F, Einhorn T A. Production of a standard closed fracture in laboratory animal bone. J Orthop Res 1984; 2: 97–101
- Cholley F, Edery P, Ricquier D, Peudenier S, Slama A, Tardieu M. Mitochondrial respiratory chain deficiency revealed by hypothermia. Neuropediatrics 2001; 32: 104–6
- Conley K E, Jubrias S A, Esselman P C. Oxidative capacity and ageing in human muscle. J Physiol 2000; 526: 203–10
- Desai B J, Meyer M H, Porter S, Kellam J F, Meyer R A, Jr. The effect of age on gene expression in adult and juvenile rats following femoral fracture. J Orthop Trauma 2003; 17: 689–98
- Desai V G, Feuers R J, Hart R W, Ali S F. MPP+-induced neurotoxicity in mouse is age dependent: Evidenced by the selective inhibition of complexes of electron transport. Brain Res 1996; 715: 1–8
- Etienne W, Meyer M H, Peppers J, Meyer R A, Jr. Comparison of mRNA gene expression by RT-PCR and DNA microarray. BioTechniques 2004; 36: 618–26
- Gothlin G. Electron microscopic observations on fracture repair in the rat. Acta Pathol Microbiol Scand (A) 1973; 81: 507–22
- Hadjiargyrou M, Lombardo F, Zhao S, Ahrens W, Joo J, Ahn H, Jurman M, White D W, Rubin C T. Transcriptional profiling of bone regeneration. Insight into the molecular complexity of wound repair. J Biol Chem 2002; 277: 30177–82
- Ketenjian A Y, Arsenis C. Morphological and biochemical studies during differentiation and calcification of fracture callus cartilage. Clin Orthop 1975, 107: 266–73
- Lesnefsky E J, Hoppel C L. Ischemia-reperfusion injury in the aged heart: Role of mitochondria. Arch Biochem Biophys. 2003; 420: 287–97
- Lu C, Miclau T, Hu D, Hansen E, Tsui K, Puttlitz C, Marcucio R S. Cellular basis for age-related changes in fracture repair. J Orthop Res 2005; 23: 1300–7
- Martin J A, Buckwalter J A. Aging, articular cartilage chondrocyte senescence and osteoarthritis. Biogerontology 2002; 3: 257–64
- Meyer M H, Meyer R A, Jr. Genes with greater up-regulation in the fracture callus of older rats with delayed healing. J Orthop Res 2007, (in press)
- Meyer M H, Etienne W, Meyer R A, Jr. Altered mRNA expression of genes related to nerve cell activity in the fracture callus of older rats: A randomized, controlled, microarray study. BMC Musculoskelet Disord 2004; 5: 24
- Meyer R A, Jr, Tsahakis P J, Martin D F, Banks D M, Harrow M E, Kiebzak G M. Age and ovariectomy impair both the normalization of mechanical properties and the accretion of mineral by the fracture callus in rats. J Orthop Res 2001; 19: 428–35
- Meyer R A, Jr, Meyer M H, Tenholder M, Wondracek S, Wasserman R, Garges P. Gene expression in older rats with delayed union of femoral fractures. J Bone Joint Surg (Am) 2003; 85: 1243–54
- Meyer R A, Jr, Desai B R, Heiner D E, Fiechtl J, Porter S, Meyer M H. Young, adult, and old rats have similar changes in mRNA expression of many skeletal genes after fracture despite delayed healing with age. J Orthop Res 2006; 24: 1933–44
- O'Driscoll S W, Saris D B, Ito Y, Fitzimmons J S. The chondrogenic potential of periosteum decreases with age. J Orthop Res 2001; 19: 95–103
- Petersen K F, Befroy D, Dufour S, Dziura J, Ariyan C, Rothman D L, DiPietro L, Cline G W, Shulman G I. Mitochondrial dysfunction in the elderly: Possible role in insulin resistance. Science 2003; 300: 1140–2
- Quarto R, Thomas D, Liang C T. Bone progenitor cell deficits and the age-associated decline in bone repair capacity. Calcif Tissue Int 1995; 56: 123–9
- Rosenfeldt F L, Pepe S, Linnane A, Nagley P, Rowland M, Ou R, Marasco S, Lyon W. The effects of ageing on the response to cardiac surgery: Protective strategies for the ageing myocardium. Biogerontology 2002; 3: 37–40
- Sekine J, Sano K, Inokuchi T. Effect of aging on the rat condylar fracture model evaluated by bromodeoxyuridine immunohistochemistry. J Oral Maxillofac Surg 1995; 53: 1317–21
- Tonna E A. Response of the cellular phase of the skeletion to trauma. Periodontics 1966; 4: 105–14
- Tonna E A, Cronkite E P. The periosteum: Autoradiographic studies on cellular proliferation and transformation utilizing tritiated thymidine. Clin Orthop 1963, 30: 218–32
- Tonna E A, Pillsbury N. Mitochondrial changes associated with aging of periosteal osteoblasts. Anat Rec 1959; 134: 739–59
- Varanasi S S, Francis R M, Berger C E, Papiha S S, Datta H K. Mitochondrial DNA deletion associated oxidative stress and severe male osteoporosis. Osteoporos Int 1999; 10: 143–149
- Wei Y H, Lee H C. Oxidative stress, mitochondrial DNA mutation, and impairment of antioxidant enzymes in aging. Exp Biol Med 2002; 227: 671–82
- Zar J H. Biostatistical Analysis. Prentice-Hall, Inc, Englewood Cliffs 1984