Abstract
Background The initial stability of an exchanged hip arthroplasty is crucial for the survival of the revised joint. Several factors can affect the outcome. The amount of liquid in morsellized bone has a major influence on the constrained stiffness properties of impacted bone applied in revision joint surgery.
Method To determine whether water or fat is the main contributing liquid, we performed an experimental study on impacted morsellized cortico-cancellous bovine bone to compare the constrained e-moduli in native bone and bone with modified water and fat content. The bone was impacted into bone pellets by a standardized method by which the construction procedure was monitored. Other stiffness properties were recorded during subsequent load testing.
Results Low water content significantly increased the constrained stiffness moduli during load, while high water content significantly reduced it. Low fat content increased stiffness significantly only during the initial phase of loading.
Interpretation Our findings indicate that the preparation and usage of morsellized bone in revision joint surgery should be performed under dry conditions to improve the initial stability of the revised prosthesis. ▪
The total number of primary hip arthroplasties has been increasing during the last decade. In the same period, reports from the Swedish National Total Hip Arthroplasty Register have shown that the number of revision arthroplasties has remained constant (Herberts and Malchau Citation2000). Cemented prostheses, surrounded by an envelope of impacted morsellized bone, are widely used in revision hip surgery (Gie et al. Citation1993, Slooff et al. Citation1993, Welten et al. Citation2000, Schreurs et al. Citation2003). Early axial subsidence and torsion may disturb the geometry of the implant system and hence reduce the durability of the revised prosthesis (Franzén et al. Citation1995, Eldridge et al. Citation1997, Brodt et al. Citation1998, Ornstein et al. Citation2002). Various factors influence the mechanical stability of impacted bone: particle size and grading (Brewster et al. Citation1999, Ullmark Citation2000), moisture (Voor et al. Citation2000, Dunlop et al. Citation2003), addition of bone substitutes (Verdonschot et al. Citation2001), and impaction energy (Ullmark Citation2000, Fosse et al. Citation2004). Despite extensive application, the significance and function of several of these factors is obscure.
Impacted bone behaves in a visco-elastic fashion, with time and stress-dependent deformation (Giesen et al. Citation1999). The bone granules form a matrix with a void porous space mainly filled with air, water and fat. According to principles of soil mechanics, deformation in loaded granulated and moist matter depends on pore pressure, the friability of each morsel and the movement of bone particles in relation to each other. Constrained stiffness is assumed to be optimal when the particles become interlocked, thus preventing further sliding (Janbu Citation1963). In this experimental study, we concentrated on the importance of the fluid content of impacted morsellized bone. To what extent does the presence of water and fat influence deformation during impaction and loading?
Material and methods
Bone samples were obtained from the juxtaarticular bone of newly slaughtered 3–5-year-old cattle. To confirm the homogeneity of the whole bone, the bone mineral density was measured using 2-mm sliced CT-images (Somatomar; Siemens AG, Forcheim, Germany) and DEXA (Hologic QDR 4500 software: subregion array spine; Bedford, Massachusetts, USA) before it was ground. Mean mineral density of the intact bone was 1.45 ± 0.25 g/cm3(unpublished data).
Connective tissue and joint cartilage were removed from the bones before storage in a freezer at –26°C. A standard grinder (Howex 100; Howex AB, Sweden) for clinical application was used to grind the bone. All morsellized bone was carefully mixed to achieve homogenous bone quality (Brodt, Swan and Brown Citation1998). A portion of loose granulated bone was separated in an approved 5-level sieve to characterize the dispersion of the particle sizes produced (Analysensieb DIN ISO 3310; Fritsch, Laborgerätebau, Idar-Oberstein, Germany). Based on the cumulative mass distribution curve, the bone had a median morsel size of 2.0 mm (Craig Citation2004).
The morsellized bone was stored in a freezer (–26°C) until further use, and it was thawed in portions. The moisture and fat content of the bone mass were manipulated to obtain morsellized bone of 5 qualities, before production of bone pellets by a bone impaction jig which had been constructed for this purpose ().
Figure 1. Flow chart of study logistics from intact bone to production of morsellized bone, the preparation of different water and fat modifications, and the construction of bone pellets by the standard impaction routine applied to each of the 32 pellets.Dotted lines indicate on which levels measurements were performed.ICME:impaction constrained modulus of elasticity;AMD:apparent mass density;CCME:consolidated constrained modulus of elasticity;TCME:total constrained modulus of elasticity; BMD: bone mineral density.
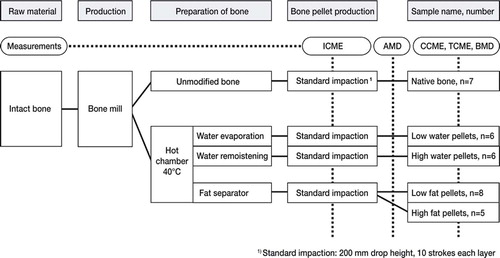
A) Native bone. Using our standard impaction procedure (Fosse et al. Citation2004), we constructed seven bone pellets from a portion of unmodified morsellized bone.
B) Low-water-content bone. A weighed mass of morsellized bone was evaporated in a hot chamber at 40°C for 3 weeks. The temperature was chosen to avoid coagulation of protein. Bone mass weight was measured daily. After a weight loss of 15%, there was no further decrease in weight, and the bone was considered dry. Prior to bone impaction, the loose dried bone was carefully and thoroughly stirred to assure an even distribution of fat. We made 6 bone pellets from a portion of dried bone by our standard impaction procedure. This sample constituted the low-water-content pellets.
C) High-water-content bone. Another portion of dried bone was moistened by mixing it carefully with 0.9% saline solution to increase its weight by 25%. Immediately afterwards, the loose bone mass was impacted into 7 pellets.
D) Bone with 3 levels of fat content. The fat content was reduced by the combined effects of temperature and gravity. 7 Plexiglas tubes (inner diameter 36 mm, height 250 mm) were filled with morsellized bone and mounted vertically in jars (). The lower end opening of the tubes was closed by a stainless steel mesh (0.63 × 0.63 mm with thread diameter 0.2 mm) which kept the bone within the tube, but allowed gravity to drain fat. To reduce fat viscosity, these separators were placed in an incubator at 40°C for 4 weeks. The evaporative weight loss was recorded. Loose bone from tubes was squeezed out into 3 even portions (top, middle and bottom part) by a piston. Each third was carefully stirred to distribute moisture evenly. The bone was then impacted into bone pellets by a standard method. 4 pellets were produced from bone from the upper third, 4 from the middle third and 5 were made from loose bone from the lower third.
Figure 2. Fat separator consisting of a perpendicular Plexiglas cylinder filled with morsellized bone. Fat is drained by gravity through a stainless steel mesh at the bottom (arrow). To facilitate fat drainage by reducing viscosity, the separator was kept in an incubator (40°C).
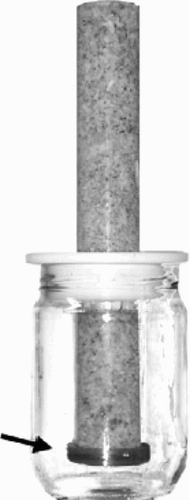
Before impaction into pellets, one portion of bone of each quality, except for the small amounts of remoistened bone, was put aside for assessment of fluid content.
Impaction technique
A jig with an instrumented slap hammer was designed to perform controlled impactions, with impaction forces that resembled those in clinical practice. A 660-gram slap hammer, hitting a load cell connected to a piston, delivered the impaction force. The morsellized bone was impacted into a cylindrical acetic plastic (POM) chamber of inner cross-sectional area 10.0 cm2 and height 15.0 mm (). This bone impaction chamber was tight and did not allow fluids to escape during impaction. The slap hammer, load cell, piston and bone impaction chamber were all kept in line by a central, vertical rod, which ensured that the impaction force of the hammer compressed the bone strictly vertically at each blow. To make the first layer of one bone pellet, 4.0 g of loose bone granules was fed into the chamber, and impacted by dropping the slap hammer 10 times from 200 mm. More bone was added, and the standard impaction procedure repeated until the bone chamber was full. Bone impacted in an impaction chamber was defined as a bone pellet (Fosse et al. Citation2004).
Figure 3. The impactor rig assembled (left). The impactor jig (middle picture) shows the sliding hammer, load cell, piston and bone chamber, kept in line by a central rod. Strain gauges are attached to the vertical bars of the load cell. The sliding hammer strikes the top of the load cell. Strain gauges record the deformation of the passing energy wave, before it compresses the morsellized bone in the bone impaction chamber (right) under the piston. Deformation of the load cell is dependent on the energy mass and stiffness of the bone material.
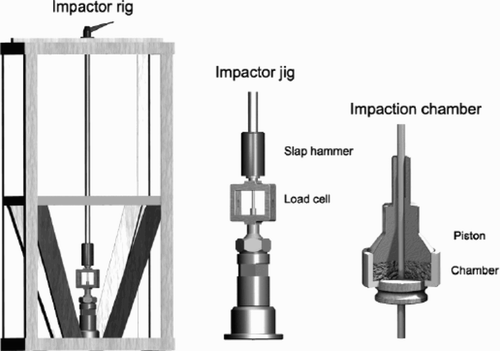
Measurements on impacted bone pellets
The height and weight of each pellet were recorded to calculate the volume, and thus determine the apparent mass density (AMD). During the production of pellets, each stroke gave a brief impact in the load cell. These deformations were recorded by strain gauges and were interpreted as reflecting the stiffness of the bone material at the instant of one particular impaction blow. The construction of a bone pellet was thus followed stroke-by-stroke and layer-by-layer. The fifth measurement in the fourth layer was chosen to represent the pellet as a whole, and it was defined as the impact constrained module of elasticity (ICME) (Fosse et al. Citation2004).
The bone pellets were prepared and tested in a load testing machine (Lloyd LR 10K with load cell DCL 2.5 kN, Lloyd instruments Ltd., Hants, England) to obtain the load-deformation characteristics of the pellet (Fosse et al. Citation2004). Incremental load-steps were used to obtain a gradual dissipation of excess pore pressures during the load step. The load steps were carried out at load levels of 10, 45, 95, 400, 800, 1,400 and 2,300 N. The load head speed was 10 mm/min. Bone samples were compressed without stress relaxation for 20 min at each load level.
The initial load-deformation characteristics of a bone pellet are governed by pore pressure, as the mass is considered undrained. The change in porosity during loading in this short consolidation phase is governed by the fluid content and drainage conditions. The granules will not, however, slide in-between each other unless there is space available in which to move. The deformation of the pellet during this short period is controlled by the pore pressure. The corresponding stiffness property is called the consolidated constrained modulus of elasticity (CCME), which refers to the deformation level at the end of the consolidation period. CCME is derived from the undrained period of the stress-strain curve (). Data from load level 1,400 N are referred to, as this load approximates the vertical load in the impacted bone of the revised hip joint during standing (Huiskes and Verdonschot Citation1997). The duration of the theoretical consolidation period is estimated through Taylor's construction on the time-deformation curve (Janbu Citation1963). It is reasonable to assume that this is the same for morsellized bone (Dunlop Citation2004).
Figure 4. Strain-time progress (○) at load level 1,400 N for one native bone pellet (graph a) illustrates the theoretical duration of the consolidation period based on Taylor's construction (broken line). Strain-stress progress (▴) for all load levels (graph b) indicates the relationship between consolidated and total constrained modulus of elasticity (CCME and TCME) of the impacted bone. CCME is the slope of the initial deformation during consolidation at load level 1,400 N, while TCME indicates the slope of total deformation lapse from zero to 1,400 N. CCME is by far the highest. Incompressible fluids govern the deformation before the pellet is drained and consolidated.
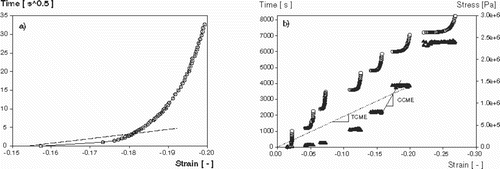
When the pore pressure at continuous load is fully dissipated, the bone mass is considered drained. Further deformation depends mainly on the sliding of the granules and the brittleness of each particle. These creep settlements are mainly due to particle size and form, size grading, surface friction factors and the fragility of each granule. For practical purposes, a combined elastic modulus was determined, incorporating theoretical consolidation period and creep period: the total constrained modulus of elasticity (TCME) (). Hence, TCME describes the deformation progress from the load test start at 0.01 kN, including load step 1.4 kN.
All bone pellets were tested for their bone mineral density (BMD) by dual-energy X-ray assay (Hologic QDR 4500 software; subregion array spine (Orwoll and Oviatt Citation1991)). BMD was measured after the load test and the results were corrected for the height of the pellet. Impacted and load-tested bone pellets and corresponding loose bone material were put into a 103°C oven for approximately 4.5 h and dried to constant weight. The relative loss of weight of the total dry mass was registered as the water content by mass. After evaporation of water, fat was extracted using diethyl ether in accordance with the Soxtec System HT. The relative loss of weight was registered as the percentage of fat (Commission Directive 98/64/EC of 3 September, Citation1998).
Statistics
The differences in the outcome variables AMD, BMD, ICME, CCME and TCME were tested by ANOVA. Tukey's HSD was used as a post hoc test.
Statistical analyses were done using SPSS 11.5 (SPSS Inc., Chicago, Ill, USA). P-values < 0.05 were considered to indicate significant differences.
Results
The native loose bone of the baseline study contained 11% water and 34% fat (). Evaporation of the bone in a drying chamber reduced the water content to 2.8% without changing the fat content. Low-water-content bone pellets had an increased AMD (apparent mass density), p = 0.002, increased BMD (bone mineral density), p < 0.001, increased CCME (consolidated constrained modulus of elasticity), p < 0.001, and increased TCME (total constrained modulus of elasticity), p < 0.001, compared to the native bone pellets (). High-water-content pellets, however, had a higher AMD (p < 0.001) than native bone, but lower constrained deformation moduli than other samples.
Table 1. Water and fat distribution as measured in loose bone and impacted bone. A hyphen indicates that no material was analyzed
Table 2. Relationship of outcome values after impaction of loose bone with 5 different fluid concentrations into bone pellets. Mean values are given with standard deviation values in parentheses
Two of the Plexiglas fat separator tubes were discharged due to mold formation. The water content of loose bone from each of the remaining tube thirds was low, but with no significant difference. The fat content was significantly higher in the bottom third, and there was no difference in fat content between the upper two thirds (). The corresponding bone pellets had the same fat distribution as the loose bone thirds. Hence, the pellets made of fat-separated bone were regrouped into low fat (n = 8) and high fat (n = 5) pellets. These pellets had similar (low) water content.
Low-fat-content pellets had significantly higher CCME than high-fat-content pellets. No significant differences could be seen in any other outcome variable between these two samples of bone. However, both had significantly higher constrained deformation moduli than bone pellets with high water content. There was a change in these moduli even compared to native bone, though this rise was not significant.
Discussion
In this study, the physical properties of the loose unmodified bone were comparable to findings in corresponding studies (Brodt et al. Citation1998, Brewster et al. Citation1999, Bavadekar et al. Citation2001, Verdonschot et al. Citation2001). Mechanically, the bone pellets were characterized by their axial stiffness. Clinically, impaction and load forces consist of both axial and shear stresses. This is, however, a very complicated scenario and the single effects are almost impossible to measure synchronously. The present method is reproducible and capable of being standardized, and it represents the mechanical behavior of impacted bone in a realistic manner.
We considered it important to dry the morsellized bone without affecting any constituents by chemical or mechanical means. Extracting water chemically could change the character of proteins, as might temperatures above 40°C. Centrifuging the bone mass could change the size and distribution, with unpredictable effects on mechanical behavior (Brewster et al. Citation1999). Granulate moisture influences mechanical behavior (Giesen et al. Citation1999, Ullmark and Nilsson Citation1999, Voor et al. Citation2000, Dunlop et al. Citation2003). The first impaction strokes on loose morsellized bone remove air and some fluids. Synchronously, the strokes organize a matrix of particles embedded in a void space, which is filled with air, organic debris and incompressible liquids. The stress of swift strokes may not facilitate effective and persistent drainage, and the result of impaction also depends on the amount of fluid to be drained. During loading, an initial pore pressure delays deformation. As the pore pressure is fully dissipated, the sample is drained, and the pellet becomes deformed mainly because of creep. Morsellized bone surface properties such as shape and roughness—together with liquid properties such as viscosity—govern friction, cohesion and surface tension. These properties may influence drainage during impaction and loading.
Low water content in morsellized bone considerably affects the axial stiffness of impacted bone during load testing. However, evaporated dried bone had an ICME (impact constrained modulus of elasticity) that was lower than, but not significantly different from, baseline bone. Dried and impacted bone may be too stiff to be measured by the present ICME measuring method. The stroke impact peak of these stiff pellets is probably too short and sharp to be recognized by the current amplifier.
A completely dry morsellized impacted bone graft may be stable. However, liquids lubricate and favor particle movement during impaction, resulting in a denser material. Additionally, a certain amount of fluid improves cohesion, as the particles adhere to each other more efficiently. Particles that cohere may restrain further compression during impaction; and during loading, cohesion probably improves stiffness by its gluing effect. Further studies on this subject are needed.
Voor and co-workers (Citation2000) stated that a low liquid content reduces the strain level. Their samples were made from cancellous bovine bone, and the impaction force was almost 25% higher than in our study. They emphasized the important connection between liquid fraction and mechanical behavior, and stated that fat has more influence than water. Lower fat content results in reduced viscosity, which should enhance drainage. How-ever, their conclusion was based on only one bone pellet in each sample.
We separated fat from loose bone material by gravity and temperature, which does not damage the remaining mass. Water evaporated during preparation in the drying chamber (40°C), but it is assumed that the temperature did not change the physical or chemical properties of the proteins. ICME decreased, though not significantly, in the low-fat-content bone pellets. In the short phase of an impaction stroke, pellet deformation is mainly governed by pore pressure, and the speed of drainage is crucial. Low fat content reduces viscosity, facilitating drainage, and the pellet is further compressed. On the other hand, reducing fat content significantly increases CCME (consolidated constrained modulus of elasticity) during the early, consolidation phase of loading. This difference vanished as the samples were drained and fully consolidated.
In the drained, consolidated phase, creep dominates deformation. TCME (total constrained modulus of elasticity) represents the mechanical properties of impacted morsellized bone in clinical use. The mechanical behavior of pellets with low and high fat fractions is comparable to those of low-water-content bone pellets. A high TCME is mainly due to low water content. However, these dried samples all had constrained deformation moduli that were significantly superior to those of native bone pellets.
Conclusions
The liquid part of morsellized bone consists of water and fat. For practical purposes, it does not seem worthwhile to emphasize the extraction of only one of these fluids before impaction. Bone morsellized for impaction in revision joint surgery should be prepared and applied under as dry conditions as possible. This will increase the initial mechanical stability of the revised prosthesis. However, further studies are required to investigate the biological effects of this improvement.
- Bavadekar A, Cornu O, Godts B, Delloye C, Van Tomme J, Banse X. Stiffness and compactness of morselized grafts during impaction: nan in vitro study with human femoral heads. Acta Orthop Scand 2001; 72(5)470–6
- Brewster N T, Gillespie W J, Howie C R, Madabhushi S P, Usmani A S, Fairbairn D R. Mechanical considerations in impaction bone grafting. J Bone Joint Surg (Br) 1999; 81(1)118–24
- Brodt M D, Swan C C, Brown T D. Mechanical behavior of human morselized cancellous bone in triaxial compression testing. J Orthop Res 1998; 16(1)43–9
- Commission Directive 98/64/EC of 3 September, 1998. Determination of crude oils and fats. Commission Directive 98/64/EC of 3 september 1998 establishing Community methods of analysis for the determination of aminoacids, crude oils and fats, and olaquindox in feed-ingstuffs and amending. 1998; 98, Directive 71/393/EEC
- Craig R F. Craig's soil mechanics. Spon Press, London 2004
- Dunlop D. Impaction bone grafting. A mechanical appraisal with reference to soil engineering. Impaction bone grafting in revision arthroplasty, C Delloye, Bannister, G. Marcel Dekker, Inc, New York 2004; 57–72
- Dunlop D G, Brewster N T, Madabhushi S P, Usmani A S, Pankaj P, Howie C R. Techniques to improve the shear strength of impacted bone graft: the effect of particle size and washing of the graft. J Bone Joint Surg (Am) 2003; 85(4)639–46
- Eldridge J D, Smith E J, Hubble M J, Whitehouse S L, Learmonth I D. Massive early subsidence following femoral impaction grafting. J Arthroplasty 1997; 12(5)535–40
- Fosse L, Rønningen H, Lund-Larsen J, Benum P, Grande L. Impacted bone stiffness measured during construction of morsellised bone samples. J Biomechanics 2004; 37(11)1757–66
- Franzén H, Toksvig-Larsen S, Lidgren L, Onnerfält R. Early migration of femoral components revised with impacted cancellous allografts and cement. A preliminary report of five patients. J Bone Joint Surg (Br) 1995; 77(6)862–4
- Gie G A, Linder L, Ling R S, Simon J P, Slooff T J, Tim-perley A J. Impacted cancellous allografts and cement for revision total hip arthroplasty. J Bone Joint Surg (Br) 1993; 75(1)14–21
- Giesen E B, Lamerigts N M, Verdonschot N, Buma P, Schreurs B W, Huiskes R. Mechanical characteristics of impacted morsellised bone grafts used in revision of total hip arthroplasty. J Bone Joint Surg (Br) 1999; 81(6)1052–7
- Herberts P, Malchau H. Long-term registration has improved the quality of hip replacement: a review of the Swedish THR Register comparing 160,000 cases. Acta Orthop Scand 2000; 71(2)111–21
- Huiskes R, Verdonschot N. Biomechanics of artificial joints: The hip. Basic orthopaedic biomechanics, V C Mow, W C Hayes. Lippencott-Raven Publishers, Philadelphia 1997; 2: 395–460
- Janbu N. Soil compressibility as determined by oedometer and triaxial tests. Procedings, European Conference on Soil Mechanics and Foundation Engineering. Wiesbaden, 1963; 1+2
- Ornstein E, Atroshi I, Franzen H, Johnsson R, Sandquist P, Sundberg M. Early complications after one hundred and forty-four consecutive hip revisions with impacted morselized allograft bone and cement. J Bone Joint Surg (Am) 2002; 84(8)1323–8
- Orwoll E S, Oviatt S K Longitudinal presicion of dual energy x-ray arbsorptiometry in a multi-center study. J Bone Miner Res 1991; 6: 191–7
- Schreurs B W, Thien T M, de Waal Malefijt M C, Buma P, Veth R P, Slooff T J. Acetabular revision with impacted morselized cancellous bone graft and a cemented cup in patients with rheumatoid arthritis: three to fourteen-year follow-up. J Bone Joint Surg (Am) 2003; 85(4)647–52
- Slooff T J, Schimmel J W, Buma P. Cemented fixation with bone grafts. Orthop Clin North Am 1993; 24(4)667–77
- Ullmark G. Bigger size and defatting of bone chips will increase cup stability. Arch Orthop Trauma Surg 2000; 120(7–8): 445–7)
- Ullmark G, Nilsson O. Impacted corticocancellous allografts: recoil and strength. J Arthroplasty 1999; 14(8)1019–23
- Verdonschot N, van Hal C T, Schreurs B W, Buma P, Huiskes R, Slooff T J. Time-dependent mechanical properties of HA/TCP particles in relation to morsellized bone grafts for use in impaction grafting. J Biomed Mater Res 2001; 58(5)599–604
- Voor M J, Nawab A, Malkani A L, Ullrich C R. Mechanical properties of compacted morselized cancellous bone graft using one-dimensional consolidation testing. J Biomech 2000; 33(12)1683–8
- Welten M L, Schreurs B W, Buma P, Verdonschot N, Slooff T J. Acetabular reconstruction with impacted morcellized cancellous bone autograft and cemented primary total hip arthroplasty: a 10- to 17-year follow-up study. J Arthroplasty 2000; 15(7)819–24