Abstract
β-Glucosidase from Trichoderma reesei was immobilised on synthetic superparamagnetic magnetite (Fe3O4) nanoparticles with a mean diameter of 10 nm and were used to supplement cellulase in the enzymatic hydrolysis of three substrates: wheat straw pretreated by steam explosion, Eucalyptus globulus pretreated by hydrothermolysis and E. globulus pretreated by hydrothermolysis followed by alkaline extraction. The hydrolysis yields for each pretreated material, using immobilised β-glucosidase (I-βG) and free cellulase, were 76.1%, 83.6% and 75.6%, respectively, and resulted in an improved hydrolysis yields compared with only cellulase. These yields were at most 10% lower than yields reached with free enzymes. The (I-βG) was magnetically recovered and successfully reused twice. The differences in the hydrolysis yields were not significant (p > 0.05) in the case of steam-exploded wheat straw and E. globulus pretreated by hydrothermolysis followed by alkaline extraction. The immobilisation of enzymes provides an opportunity to reduce the costs of enzymes in the bioethanol production process.
1. Introduction
Studies of the production of bioethanol from lignocellulosic biomass have dramatically increased over the years due to its potential use as an alternative fuel Citation1 with various benefits including the reduction of greenhouse gas emissions by approximately 30–85% in comparison with gasoline, promotion of energy independence and increased rural economic development Citation2.
Lignocellulose is the most abundant renewable biomass produced by photosynthesis and consists primarily in plant cell wall materials, a complex natural composite with three main biopolymers: cellulose, hemicellulose and lignin Citation3,Citation4. The bioconversion of this substrate to bioethanol involves three major steps: a pretreatment process to reduce substrate recalcitrance; enzyme catalysed hydrolysis of cellulose and hemicellulose components to fermentable sugars and fermentation to produce ethanol Citation3.
Enzymatic hydrolysis is a process catalysed by a group of enzyme-denominated cellulases with different enzymatic activities that synergically act over cellulose. Cellulases are produced extracellulary by a large number of microorganisms including fungi, actinomycetes and bacteria Citation5. Cellulase from Trichoderma reesei is the most common choice for enzymatic hydrolysis in bioethanol production due to its high level of extracellular cellulase Citation5,Citation6.
There are three major types of cellulase enzymes. Endo-β-1,4-glucanase (EG or endo-1,4-β-D-glucan-4-glucanhydrolase, EC 3.2.14) produces nicks in the cellulose polymer exposing reducing and non-reducing ends. Exoglucanases, including cellodextrinases (1,4-β-D-glucan-4-glucanhydrolase, EC 3.2.1.74) and cellobiohydrolase (CBH or 1,4-β-D-glucancellobiohydrolase, EC 3.2.1.91), act upon reducing and non-reducing ends to liberate cello-oligosaccharides, cellobiose and glucose. Finally, β-glucosidase (BG-EC 3.2.1.21) cleaves the cellobiose to release glucose, thereby completing the hydrolysis process Citation5.
The technology to generate fermentable sugars by enzymatic hydrolysis is a key step in the production of bioethanol from pretreated lignocellulose material, although the enzyme cost has been significantly reduced in the last decade still is considered an economic barrier to full-scale process commercialisation Citation7 because it contributes to cellulosic ethanol production cost in approximately 20–40% Citation5. This high cost is due to the poor activity of cellulases, the large amounts used and long incubation times Citation2.
Since covalent bonds provide the strongest linkages between enzyme and carrier Citation8, covalent enzymatic immobilisation presents an opportunity to retain and recycle an expensive ingredient of the bioethanol process reducing the costs of catalyst production. Magnetite (Fe3O4) nanoparticles, a magnetic iron oxide, have been widely applied to immobilise enzymes and proteins such as lipase Citation9, glucose oxidase Citation10, bovine serum albumin (BSA) Citation11 and homing peptides Citation12. Cellulase and β-glucosidase has also been successfully immobilised using Fe3O4 as support Citation13–16. Immobilised β-glucosidase (I-βG) from Aspergillus niger on Fe3O4 particles functionalised with polyethyleneimine was used in enzymatic hydrolysis of lignocellulosic material with a good hydrolysis yield Citation14. It has also been immobilised on nanoparticles functionalised with 3-aminopropyltriethoxysilane (3-APTS) Citation15 and on polyacrylamide-Fe3O4 beads Citation16.
The main limitation of commercial cellulase is the relatively low amounts of β-glucosidase enzyme. Therefore, the cellulose complex must be supplemented with β-glucosidase enzyme to reach higher concentrations of glucose and to avoid the inhibition of endo- and exoglucanases by cellobiose. In this work, the covalent immobilisation of β-glucosidase enzyme on synthetic superparamagnetic Fe3O4 nanoparticles and their application in the saccharification process were studied. The magnetic property of the bioconjugates enzyme/Fe3O4 nanoparticles should improve the recovery of the enzyme magnetic support system in biotechnology applications, such as the saccharification process of pretreated lignocellulosic materials used in bioethanol production.
2. Materials and methods
2.1. Materials
All reagents were pro-analysis and used as received without further purification. FeSO4 · 7H2O, FeCl3 · 6H2O, ammonia 25% wt, 3-APTS, glutaraldehyde 25% wt, cellobiose, D-glucose, D-xylose and D-arabinose were purchased from Merck. p-Nitrophenyl β-D-glucopyranoside and p-nitrophenol were purchased from Sigma. Nanopure water (NPW) was used in all preparations, unless otherwise stated. Cellulase complex (NS50013) and β-glucosidase (NS50010) enzymes were kindly provided by Novozymes (USA).
2.2. Synthesis of Fe3O4 nanoparticles
Superparamagnetic Fe3O4 nanoparticles with a mean diameter of 10 nm were synthesised by co-precipitation route from Fe2+/Fe3+ in aqueous solutions (molar ratio 1:2) by adding ammonia under constant stirring at 10,000 rpm Citation17. Shape, morphology and size distribution of the Fe3O4 nanoparticles were determined using transmission electron microscopy (TEM). The images were taken using a JEOL JEM 1200EXII microscope at an accelerating voltage of 120 kV. The samples were re-dispersed in a NPW:ethanol (1:1) matrix, and then deposited on a 200-mesh carbon-nitrocellulose-coated TEM copper grid. To determine the size distribution and the mean average size, 100 particles were measured for each sample.
2.3. Covalent immobilisation of β -glucosidase
Covalent bonding between enzymes and carriers is based on a chemical reaction between the active amino acid residues on the enzyme surface and the active functional groups that are attached to the carrier's surface Citation18. The first step to immobilise the enzyme is to prepare amino-functionalised Fe3O4 nanoparticles. Thus, 1.5 g of dry Fe3O4 was re-dispersed in 200 mL of ethanol for 30 min by sonication. Then, 2.0 mL of 3-APTS were added and this solution was kept at room temperature overnight under magnetic stirring. Silanized nanoparticles were magnetically harvested and washed repeatedly with ethanol and dried under vacuum at room temperature. Fourier transform infrared spectroscopy (FT-IR, Perkin Elmer) was used to confirm chemical bond formation between active Fe3O4 and 3-APTS. The magnetic properties of Fe3O4 uncoated and coated by 3-APTS were analysed at 300 K using a MPMS superconducting quantum interference device magnetometer (SQUID, Quantum Design, XL model, applied field ±20 kOe).
Subsequently, 1.0 g of dry amino-functionalised Fe3O4 was re-dispersed by sonication in 100 mL of 5% glutaraldehyde 50 mM and phosphate buffer (pH = 7.4) and later stirred overnight at room temperature. The nanoparticles were then magnetically harvested and washed with buffer solution. Afterwards the nanoparticles were combined with a 50 mL solution of 2% v/v β-glucosidase (Novozymes β-glucosidase) in buffer solution. This mixture was gently stirred overnight at room temperature. (I-βG) on Fe3O4 nanoparticles were magnetically separated and washed with phosphate buffer 50 mM at pH 7.4, re-suspended in this buffer solution and stored at 4°C until used. The supernatant and the washing solutions were used to determine the unbound proteins concentration on the functionalised Fe3O4 nanoparticles. Immobilised proteins concentration was calculated from the difference between protein concentration in the initial solution and the unbound proteins concentration in the supernatant and washing solutions. These concentrations were determined using the Bio-Rad Protein assay kit based on the Bradford's method through visible spectrometry (Shimadzu UV-1650 PC, 595 nm, b = 1.0 cm). BSA was used as protein standard for the calibration curve (linear range 0.2–1.0 mg mL−1, R = 0.994). β-Glucosidase specific activity was determined by monitoring the hydrolysis of p-nitrophenyl β-D-glucopyranoside and expressed as international units per mg of protein (IU mg−1) Citation18,Citation19. Cellulase activity was determined using International Union of Pure and Applied Chemistry procedure Citation20.
2.4. Carbohydrates analysis
A Merck Hitachi LaChrom HPLC equipped with a refractive index detector, a Aminex HPX 87H column (Bio-Rad, Hercules, CA, USA) at 45°C and H2SO4 5.0 mM as mobile phase at a flow rate of 0.6 mL min−1 was used to quantify cellobiose and monosaccharide concentrations. Calibration curves of cellobiose (linear range 0.5–7.0 g L−1, R = 0.999), D-glucose (linear range 0.5–6.0 g L−1, R = 0.999), D-xylose (linear range 0.6–7.0 g L−1, R = 0.999) and D-arabinose (linear range 0.1–0.8 g L−1, R = 0.999) were made. All experiments were performed in triplicate.
2.5. (I- β G) time stability
Percentage of hydrolysis of cellobiose (2.5 g L−1) in sodium citrate buffer 50 mM at pH 4.8 was used to determine the stability of immobilised enzyme for 45 days.
2.6. Substrate preparation
Wheat straw was chipped, and 110 g of dry weight were pretreated by steam explosion (WS-SE) at 205°C and 400 psi for 5 min. Pretreated wheat straw was recovered in a cyclone.
Eucalyptus globulus chips were pretreated by hydrothermolysis (EG-H) at 180°C for 15 min in 1 gal reactor (Parr Instruments, Moline, IL, USA). Part of the pulp was subjected to delignification through alkaline extraction (EG-HA) to prevent unproductive binding of the enzymes to lignin. The hydrothermal pulp obtained was mixed with 8% NaOH solution at 10% consistency, 68°C for 1 h in 1 gal reactor. Chemical characterisation of raw and pretreated materials was performed following TAPPI Standard Method Citation21 and Puls et al. Citation22.
2.7. Enzymatic hydrolysis
Enzymatic hydrolysis was performed using a commercial preparation of T. reesei cellulase complex (61.0 FPU mL−1). 20 FPU of cellulase per gram of dry pretreated material was used, and was supplemented with I-βG on 1.0 g of silanized Fe3O4. Control experiments were carried out using 20 FPU of cellulase per g of dry pretreated material supplemented with free β-glucosidase (F-βG) and without supplementation of β-glucosidase.
Enzymatic hydrolysis of the pretreated material at 5.0% (w/w) of consistency in sodium citrate buffer 50 mM at pH 4.8 was performed in a shaker at 160 rpm and 50°C for 72 h. Samples were taken at 6, 12, 24, 48 and 72 h. The content of cellobiose and the glucose released by the enzymatic hydrolysis were analyzed by HPLC. The hydrolysis yield was expressed as the percentage of glucose released in relation to the potential glucose in the pretreated material.
3. Results and discussion
3.1. Fe3O4 silanization
The physical–chemical characterisation of synthesised superparamagnetic Fe3O4 nanoparticles with a mean diameter of 10 nm and saturation magnetisation (MS) of 72.0 emu g−1 is well documented in Valenzuela et al. Citation17. TEM image shows that the synthesised Fe3O4 nanoparticles have spherical shaper with a size distribution between 4 and 15 nm with mean diameter on 10 nm ().
displays the FT-IR spectrum of (a) Fe3O4 and (b) Fe3O4 chemically bonded to 3-APTS. The Fe3O4 spectrum shows the characteristic absorption bands of Fe–O bond at 583.05 and 633.75 cm−1. When silanized, these absorption bands slightly shifts to higher wave numbers 584.07 and 634.80 cm−1, respectively, a phenomenon attributed to the formation of Fe–O–Si bonds. Fe–O–OH groups on the surface of Fe3O4 nanoparticles are replaced by Fe–O–Si(O–)2–R. Since –Si(O–)2 is more electronegative than H, the strength of the Fe–O bond is enhanced, producing the shift of the absorption bands Citation23. The absorption band at 1129.38 cm−1, assigned to the stretching vibration of the C–N bond; the band at 1029.42 cm−1, due to the stretching vibration of the Si–O bond; the band at 3397.08 cm−1, due to the stretching vibrations of –NH2 and the bands at 1568.04 and 692.92 cm−1corresponding to bending vibrations of the –NH2 group, confirmed that 3-APTS was chemically bonded to Fe3O4.
shows magnetic properties for Fe3O4 coated and uncoated with 3-APTS at 300 K. In both the cases, the magnetic curves showed no hysteresis loop and they are reversible. Additionally, neither coercivity nor remanence was observed. The saturation magnetization (M s) for uncoated Fe3O4 and coated were 72.0 and 55.9 emu g−1, respectively. These results agree with Yamaura et al. Citation24 proving that 3-APTS coating contributes as non-magnetic mass to the total sample volume.
3.2. β -Glucosidase immobilisation
Mean concentration of I-βG determined by the Bradford method was 0.10 ± 0.02 mg mL−1. Specific enzymatic activity of I-βG and F-βG was 110.7 ± 0.2 and 112.3 ± 0.3 UI mg−1, respectively.
I-βG hydrolysed 100% of cellobiose 2.5 g mL−1 in 60 min and can be magnetically harvested and reutilised over 45 days without losing its ability to hydrolyse cellobiose. These results indicate that the enzyme enzymatic activity was maintained and no denaturation of the immobilised enzyme is observed when stored at 4°C and used for different time periods at 50°C.
3.3. Enzymatic hydrolysis of pretreated material
shows the chemical composition of raw wheat straw, WS-SE, raw E. globulus, EG-H and EG-HA.
Table 1. Chemical composition of raw wheat straw, WS-SE, raw E. globulus, EG-H and EG-HA.
The dry weight in basis of glucane in WS-SE was 31.9%, corresponding to 87.6% of solid recovery of glucane from the initial raw material. The xylane component was practically completely solubilised and low amount of lignin was removed, which are characteristics feature of this pretreatment Citation2. The recovery of glucane content from E. globulus in EG-H and EG-HA were 98.9% and 96.9%, respectively. Hydrothermolysis pretreatment removed only 9.2% of lignin whereas alkaline extraction subsequent to hydrothermolysis removed 59.2% of lignin.
The results of enzymatic hydrolysis of the pretreated materials by cellulase supplemented with I-βG and F-βG and without supplementation are displayed in . The highest yields of glucose were reached at 72 h and it can be seen that the mean hydrolysis yields on pulp basis obtained for cellulase supplemented with I-βG were 76.1%, 83.6% and 75.6% for WS-SE, EG-H and EG-HA, respectively. The I-βG were magnetically recovered and reused twice and the differences in the hydrolysis yields were not significant (p > 0.05) for WS-SE and EG-HA, whereas the I-βG recycled from EG-H hydrolysis suffer a significant decrease of 10% in the yield of hydrolysis that can be attributed primarily to the loss of Fe3O4 during the multiple washes after the first recovery mainly due to the complex matrix composition of E. globulusmaterial resulting from the hydrothermolysis pretreatment that has higher content of lignin (). In the third recycle, the yield of hydrolysis decreases notoriously near 20% for WS-SE and for EG-H indicating loss in the enzymatic activity or loss of enzyme from the support during the washes. Since no improvement in the enzymatic hydrolysis was found when the hydrothermal pulp was subjected to alkaline extraction with NaOH due to the redistribution over the fibre of the 10.5% of the remaining lignin by the alkali treatment, thus decreasing the accessibility of the enzymes to the cellulose surface Citation25, a third reutilisation was not performed.
Figure 4. Glucose yields from enzymatic hydrolysis of wheat straw and E. globulus pulps at 72 h with immobilised β-glucosidase and free cellulase used three times. I-βG F-C (1), (2), (3): immobilised β-glucosidase and free cellulase (numbers of use); F-βG F-C: free β-glucosidase and free cellulase; F-C: free cellulase.
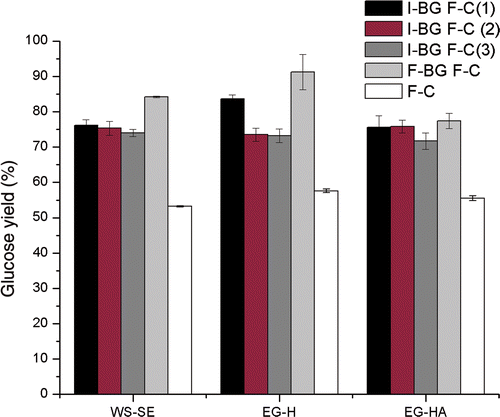
The sedimentation of I-βG during orbital shaking may explain lower hydrolysis yields for all cases when compared with the hydrolysis of cellulase supplemented with F-βG. The mean specific enzymatic activity after the third hydrolysis was 107.5 ± 1.5 IU mg−1, similar to the value before hydrolysis.
The yield of glucose during enzymatic hydrolysis using cellulase without β-glucosidase supplementation was lower than using supplementation with F-βG or I-βG, increasing the concentrations of cellobiose and glucose (data not shown) that produces end-product inhibition of the endo- and exoglucanases components of the cellulase complex.
4. Conclusion
(I-βG) on superparamagnetic amino functionalised Fe3O4 nanoparticles was successfully used to supplement cellulase for the saccharification of wheat straw pretreated by steam explosion and E. globulus pretreated by hydrothermolysis and hydrotermolysis followed by alkaline extraction. The results showed that after 72 h the enzymatic hydrolysis by cellulase supplemented with I-βG are lower than the enzymatic hydrolysis with cellulase supplemented with F-βG. This difference can be attributed to the sedimentation of the enzyme/Fe3O4 support. Immobilised enzymes were reutilised twice maintaining the hydrolysis yield without losing their specific enzymatic activity after which the yield of hydrolysis decreases.
Further studies with immobilised cellulolytic enzymes must be performed with higher concentrations of Fe3O4 or with nanoparticles with broad size to immobilised higher concentration of enzymes to increase the consistency of pretreated solids to obtain more fermentable sugars. Also simultaneous saccharification of fermentation with immobilised enzymes must be studied to consider the interaction between the pretreated solid material chosen, the enzyme/Fe3O4 complex and fermentation microorganisms, even though Fe3O4 has been used to immobilise Saccharomyces cerevisiae due to its low toxicity level. The reusability of immobilised enzymes on Fe3O4 nanoparticles without losing activity in addition to new technologies used to produce cheaper enzymes with higher enzymatic activities provide a good opportunity to reduce the overall cost of the bioethanol production process.
Acknowledgements
The financial support for this work was provided by FONDECYT (Grants Nos. 1070492 and 7070109). We greatly appreciate the contribution and support in the SQUID measurements provided by Dr S.K. Sharma and Dr M. Knobel from Low Temperature Material Laboratory, Physics Institute, UNICAMP. Roberto Valenzuela thanks the student grants received from the Science and Analytical Technology Program, Pharmacy Faculty and Graduate School of Universidad de Concepción.
References
- Kumar , S , Singh , S P , Mishra , I M and Adhikari , D K . 2009 . Recent advances in production of bioethanol from lignocellulosic biomass . Chem. Eng. Technol. , 32 : 517 – 526 .
- Sainz , M B . 2009 . Commercial cellulosic ethanol: The role of plant-expressed enzymes . In Vitro Cell. Dev. Biol. Plant. , 45 : 314 – 329 .
- Ragauskas , A J , Williams , C K , Davison , B H , Britovsek , G , Cairney , J , Eckert , C A Jr , Frederick , W J , Hallet , J P , Leak , D J , Liotta , C L , Mielenz , J R , Murphy , R , Templer , R and Tschaplinski , T . 2006 . The path forward for biofuels and biomaterials . Science , 311 : 484 – 489 .
- Zhang , Y HP , Ding , S Y , Mielenz , J R , Ciu , J B , Elander , R T , Laser , M , Himmel , M E , McMillan , J R and Lynd , L R . 2007 . Fractionating recalcitrant lignocellulose at modest reaction conditions . Biotechnol. Bioeng. , 97 : 214 – 223 .
- Sukumaran , R K . 2009 . “ Bioethanol from lignocellulosic biomass: Part II production of cellulases and hemicellulases ” . In Handbook of Plant-Based Biofuels , Edited by: Pandey , A . 141 – 158 . Boca Raton : CRC Press .
- Oliva , J M . 2003 . Efecto de los Productos de Degradación Originados en la Explosión por Vapor de Biomasa de Chopo Sobre Kluyveromyces Marxianus , Madrid : Editorial Ciemat .
- Cheng , J J and Timilsina , G R . 2011 . Status and barriers of advanced biofuel technology: A review . Renew. Energy , 36 : 3541 – 3549 .
- Cao , L . 2005 . Carrier-Bound Immobilized Enzymes: Principles, Applications and Design , Wienheim : Wiley-CHC .
- Lee , D G , Ponvel , K M , Kim , M , Hwang , S , Ahn , I H and Lee , S H . 2009 . Immobilization of lipase on hydrophobic nano-sized magnetite particles . J. Mol. Catal. B Enzym. , 57 : 62 – 66 .
- Rossi , L M , Quach , A D and Rosenzweig , Z . 2004 . Glucose oxidase-magnetite nanoparticle bioconjugate for glucose sensing . Anal. Bioanal. Chem. , 380 : 606 – 613 .
- Pan , B F , Gao , F and Gu , H C . 2005 . Dendrimer modified magnetite nanoparticles for protein immobilization . J. Colloid Interface Sci. , 284 : 1 – 6 .
- Gan , Z F , Jiang , J S , Yang , Y , Du , B , Qian , M and Zhang , P . 2008 . Immobilization of homing peptide on magnetite nanoparticles and its specificity in vitro . J. Biomed. Mater. Res. Part A , 84A : 10 – 18 .
- Garcia , A , Oh , S and Engler , C R . 1989 . Cellulase immobilization on Fe3O4 and characterization . Biotechnol. Bioeng. , 33 : 321 – 326 .
- Dekker , R FH . 1990 . Application of a magnetic immobilized β-glucosidase in the enzymatic saccharification of steam-exploded lignocellulosic residues . Appl. Biochem. Biotechnol. , 23 : 25 – 39 .
- Shinkai , M , Honda , H and Kobayashi , T . 1991 . Preparation of fine magnetite particles and application for enzyme immobilization . Biocatal. Biotransform. , 5 : 61 – 69 .
- Oswald , P R , Evans , R A , Henderson , W , Daniel , R M and Fee , C J . 1998 . Properties of a thermostable β-glucosidase immobilized using tris(hydroxymethyl)phosphine as a highly effective coupling agent . Enzyme Microb. Technol. , 23 : 14 – 19 .
- Valenzuela , R , Fuentes , M C , Parra , C , Baeza , J , Durán , N , Sharma , S K , Knobel , M and Freer , J . 2009 . Influence of stirring velocity on the synthesis of magnetite (Fe3O4) by the co-precipitation method . J. Alloys Compd. , 488 : 227 – 231 .
- Wood , T M and Bhat , K M . 1988 . “ Methods for measuring cellulase activities ” . In Methods in Enzymology, Vol. 160 , Edited by: Wood , TM and Kellogg , ST . 87 – 112 . London : Academic Press, Inc. .
- Bowers , G N Jr , McComb , R B , Christensen , R G and Schaffer , R . 1980 . High-purity 4-nitrophenol: Purification, characterization, and specifications for use as a spectrophotometric reference material . Clin. Chem. , 26 : 724 – 729 .
- Ghose , T K . 1987 . Measurment of cellulase activity . Pure Appl. Chem. , 59 : 257 – 268 .
- TAPPI . 2000 . TAPPI Test Methods. Technical Association of the Pulp and Paper Industry , Atlanta : TAPPI Press .
- Puls , J , Poutanen , K , Körner , H and Viikari , L . 1985 . Biotechnical utilization of wood carbohydrates after steaming pre-treatment . J. Appl. Microbiol. Biotechnol. , 22 : 416 – 423 .
- Ma , M , Zhang , Y , Yu , W , Shen , H , Zhang , H and Gu , N . 2003 . Preparation and characterization of magnetite nanoparticles coated by amino silane . Colloids Surf. A , 212 : 219 – 226 .
- Yamaura , M , Camilo , R L , Sampaio , L C , Macedo , M A , Nakamura , M and Toma , H E . 2004 . Preparation and characterization of (3-aminopropyl)triethoxysilane-coated magnetite nanoparticles . J. Magn. Magn. Mater. , 279 : 210 – 217 .
- Yang , B , Boussaid , A , Mansfield , S D , Gregg , D J and Saddler , J N . 2002 . Fast and efficient alkaline peroxide treatment to enhance the enzymatic digestability of steam-exploded softwood substrates . Bioechnol. Bioeng. , 77 : 678 – 684 .