Abstract
Short multi-walled carbon nanotubes (S-MWCNTs) were obtained by ball milling method and employed as a supporting matrix to explore a novel immobilisation and biosensing platform of redox proteins such as haemoglobin (Hb). Compared to long MWCNTs, the shortened MWCNTs-based biosensor did not show better electrocatalytic activity, which was contrary to previous theoretical expectations. The electron transfer rate constants of Hb on long and short MWCNTs modified electrodes in phosphate buffer solution were 1.08 and 0.95 s−1, respectively. The lower electron transfer rate between haemoglobin and the electrode can be attributed to their larger resistive properties, which were confirmed by cyclic voltammetry and AC impedance.
1. Introduction
During the past few years, the direct electron transfer (DET) reaction between redox proteins and electrode surface has been extensively studied Citation1–4 because of its importance in elucidating the intrinsic thermodynamic and kinetic properties of proteins and its potential applications in bioelectronic devices. Among the various redox proteins, haemoglobin (Hb) is one of the most important heme-containing metalloprotein, which could be utilised as an ideal model protein to explore relevant biological process and redox behaviour of heme proteins or enzymes. Unfortunately, the redox centres are shielded by the protein, thus the DET between haemoglobin and the electrode surface is rather difficult Citation5–7. Moreover, the adsorption of protein molecules onto bare electrode surface may lead to their denaturation, which decreases DET rate Citation8,Citation9. Therefore, one of the main challenges for the fabrication of high-performance electrochemical biosensors is to provide a suitable microenvironment for the proteins and enhance the DET capacity between the proteins and underlying electrodes.
Many materials were used in order to reach this goal, such as insoluble surfactants Citation10, polymers Citation11, clay Citation12, egg-phosphatidylcholine Citation13,Citation14, zirconium dioxide nanoparticles Citation15, octadecylamine Citation16, carbon nanotube (CNT) Citation17 and 11-mercaptohendecanoic acid Citation18. CNTs have attracted much attention in the past decade because of their unique properties and promising applications. Their novel properties, such as small dimensions, good conductivity, biocompatibility and high stability, make CNTs ideal candidates for constructing high-performance biosensors that effectively facilitate DET of redox proteins Citation19–21.
The mechanism of the electrocatalysis of CNTs is not fully understood yet, but it is generally believed that the electrocatalytic properties of CNTs originate from the open ends Citation22. Esplandiu's group Citation23 characterised the electrochemical performance of different composites made of bamboo-like, single-walled and multi-walled CNTs of different diameters. They suggested that the density of edges is the main parameter controlling the good electrochemical performance of the composite transducers. Therefore, short multi-walled carbon nanotubes (S-MWCNTs) with more open ends are essentially required to facilitate the electrochemical reaction of MWCNTs. However, the electrocatalysis effect of shortened MWCNTs on promoting DET reaction between redox proteins and electrode surface has not been studied yet.
Different methods have been used to cut long nanotubes into short ones, such as ultrasonication Citation24, STM voltage Citation25 and mechanical ball milling Citation26. However, ball milling method is a much more effective method to obtain uniform S-MWCNTs with open ends in terms of facilitating electrocatalytic properties of MWCNTs. Chitosan (Chi) is selected to suspense MWCNTs owing to its splendid film-forming properties, biological compatibility and good adhesion. The ball milling method was used to shorten long MWCNTs (L-MWCNTs) to obtain more edges, and the electrochemical performance of L-MWCNTs and S-MWCNTs++ modified electrodes were studied in this article. Since S-MWCNTs have more edges and defects, i.e. possessing more electroactive sites, it is expected that the S-MWCNTs/Chi has better electrochemical performance compared with that of the L-MWCNTs/Chi. Surprisingly, the experiment results turned out to be opposite: the S-MWCNTs/Chi composite was less effective. Thereby, the S-MWCNTs/Chi composite by electrochemistry method and SEM characterisation were further studied, and the results display that the S-MWCNTs were deeply buried in chitosan film. This probably led to weaker conductive property and poorer electroactive property of the S-MWCNTs/Chi composite.
2. Experimental
2.1. Chemicals and apparatus
Haemoglobin (from bovine erythrocytes, Shanghai Kayon Biological Technology CO., Ltd) and chitosan (from Crab Shells, minimum 85% deacetylated, Sigma) were used as received. CNTs (diameter 15–30 nm and length 1–2 µm) were purchased from Shenzhen Duowei High Material Company. Hydrogen peroxide (30%) was from Beijing Chemical Company. All the solutions were prepared with double-distilled water. The 0.1 M phosphate buffer solution (PBS, pH = 7.4) was always employed as supporting electrolyte. All other chemicals were of analytical grade.
Electrochemical experiments were carried out using a CHI 660B electrochemical workstation (Shanghai CHI Instrument Co.), with a conventional three-electrode cell. The modified glassy carbon electrodes (GCEs) were used as the working electrode, a platinum wire as the counter electrode, and a saturated calomel electrode as the reference electrode. The electrolyte was bubbled with high-purity nitrogen for at least 30 min prior to experiments.
Ultraviolet–visible (UV–vis) spectroscopy was carried out by a U-3010 spectrophotometer (Hitachi, Japan). The images of scanning electron microscope (SEM) and transmission electron microscope (TEM) were obtained with a field-emission SEM (LEO, 1530 VP) and TEM (JEM-100CXII, Japan), respectively. Ball milling was operated in a planetary ball mill Pulverisette (QM-3SP4, Nanjing). The measurement of specific surface areas of samples was carried out by Micromeritics Vacprep 061 sample Degas system (Micromeritics, America).
2.2. Pretreatment of MWCNTs
S-MWCNTs were prepared by ball milling of the received L-MWCNTs in an agate mortar with a single 5 cm diameter agate ball for 120 h. In addition, the ball milling amplitude was 3 mm and the frequency was 3000 vibrations min−1.
These two kinds of MWCNTs were first heated in air at 600°C for 2 h to remove the amorphous carbon. And then 50 mg MWCNTs were stirred in 100 mL of 6 mol L−1 HNO3 solution for 24 h before centrifuged. The precipitate was rinsed with deionised water and dried at 80°C in a furnace.
2.3. Modification of the electrodes
A chitosan solution (0.6 wt%) was prepared by dissolving an appropriate amount of chitosan powder in 0.10 M acetic acid solution and stirred for 30 min, and the pH was adjusted to about 5.0 with concentrated NaOH solution. A total of 1 mg L-MWCNTs and S-MWCNTs were added to 1 mL chitosan solution, respectively. The mixtures were placed in the ultrasonic bath to obtain homogeneous and black dispersions. Then 4 µL of the dispersion was dropped onto freshly polished GCE disks (3 mm in diameter) using a microsyringe. As a result, the L-MWCNTs/Chi/GCE and S-MWCNTs/Chi/GCE were well prepared after the solvent was evaporated at room temperature. The obtained electrodes were immersed into 10 mg mL−1 Hb solution at 4°C for 10 h. Furthermore, the Hb/MWCNTs/Chi GCEs were transferred to 0.1 M PBS for 15 min before use. All Hb immobilised electrodes were stored at 4°C when not in use.
3. Results and discussion
3.1. TEM characterisation of MWCNTs
shows that TEM images of the MWCNTs materials before as well as after ball milling are presented. The original nanotubes are entangled with lengths about 1–2 µm as shown in . After ball milling, nanotubes masses were broken, and the length of the nanotubes measured to be around 200–500 nm was obviously much smaller. Furthermore, the ball-milled nanotubes were separated and their bodies with open ends were straighter.
Moreover, we compared the specific surface areas of the samples. The specific surface areas of the L-MWCNTs and S-MWCNTs are 201.29 and 148.11 m−2 g−1, respectively. The former is 36% higher than the latter. Hence we expect that the shorter CNTs would show better electroactivity than the longer ones.
3.2. UV–vis characterisation of composite film
UV–vis spectroscopy is a useful tool for monitoring any possible changes of the soret absorption band which is well recognised to be associated with the heme group. The band shift may provide the information of possible denaturation of heme proteins, particularly conformational changes Citation27. shows UV–vis absorption spectra of different materials. As can be seen from the figure, no soret band appeared for MWCNTs/Chi composites while both Hb/L-MWCNTs/Chi and Hb/S-MWCNTs/Chi composites showed absorption peaks at 405 nm which peaks should be ascribed to the Hb's absorption. Although the peaks of Hb/MWCNTs/Chi composites were much lower than that of the pure Hb, they appeared the position as same as the native Hb in 0.1 M PBS, suggesting that secondary structure of Hb immobilised on the surface of MWCNTs/Chi film was not destroyed and the Hb retained its biological activity.
3.3. Direct electrochemistry of Hb and its electrocatalytic behaviour towards H2O2
First, the direct electrochemical behaviour of Hb on the modified electrodes was explored and monitored by cyclic voltammetry (CV). shows the cyclic voltammograms of MWCNTs/Chi GCE and Hb/MWCNTs/Chi GCE in N2-saturated 0.1 M PBS (pH = 7.4) at a scan rate of 0.1 V s−1. A pair of small redox peaks were observed at about −0.10 V (dashed line) when L-MWCNTs/Chi GCE was immersed in PBS, which ascribed to the redox process of the oxygen-containing groups on the MWCNTs surface and implied that the surface of the MWCNTs had been activated Citation28. As for Hb/L-MWCNTs/Chi GCE, another pair of well-defined and near symmetric redox peaks appeared at −0.306 and −0.366 V with peak separation of 60 mV and formal potential of −0.336 V. The peak located at the characteristic potentials of heme Fe(III)/Fe(II) redox couples of the proteins was similar to those previously reported, i.e. −0.334 V in PUE/MWCNTs film Citation29, −0.343 V in CTAB/MWCNTs film Citation30 and −0.341 V on AQ/PG Citation31. These results indicated that the MWCNTs/Chi composite film could provide a favourable microenvironment for Hb to exchange electrons with the electrode and accelerate the electron transfer rate.
Figure 3. CVs of: (a) Hb/L-MWCNTs/Chi (solid line) and L-MWCNTs/Chi (dashed line); (b) Hb/S-MWCNTs/Chi (solid line) and S-MWCNTs/Chi GCE (dashed line) in 0.10 M N2-saturated PBS (pH = 7.4) at a scan rate of 100 mV s−1.
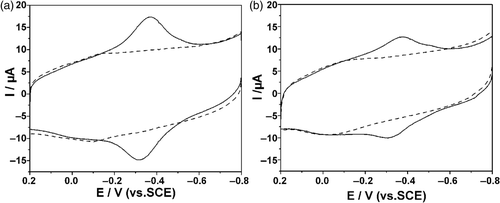
The effects of scan rate on the response of Hb/L-MWCNTs/Chi GCE are shown in . Both the reduction and oxidation peak currents increased linearly with increasing scan rate from 20 to 140 mV s−1 and peak potential separation remained almost constant, demonstrating that the electrochemical behaviour of Hb had the typical property of a surface controlled process. When n △Ep <200 mV, the heterogeneous surface electron transfer rate constant ks of Hb on the modified electrode can be estimated by Laviron equation Citation32:
Figure 4. CVs of Hb/L-MWCNTs/Chi GCE in 0.10 M N2-saturated PBS (pH = 7.4) at a scan rate of 20, 40, 60, 80, 100, 120 and 140 mV s−1 (from inner to outer). The inset shows the calibration curves of peaks vs. scan rates.
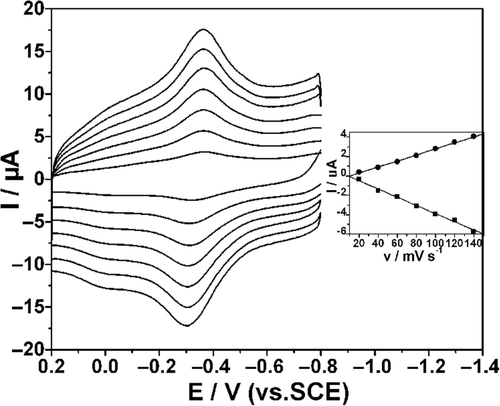
Taking a charge transfer coefficient α of 0.5, and △Ep of 60 mV at 100 mV s−1, the electron transfer rate constant ks can be calculated to be 1.08 s−1.
Hb/S-MWCNTs/Chi GCE was performed in the same condition as L-MWCNTs/Chi GCE and the cyclic voltammogram are depicted in . The direct electrochemistry of Hb was also observed on the Hb/S-MWCNTs/Chi GCE. However, the Hb/S-MWCNTs/Chi GCE showed larger peak separation and lower peak current with smaller ks of 0.95 s−1, indicating that S-MWCNTs/Chi was less effective for promoting DET of Hb compared with L-MWCNTs/Chi.
It is well known that proteins containing heme groups are capable of reducing hydrogen peroxide (H2O2) Citation33. Hence we studied the electrocatalytic behaviour of haemoglobin towards H2O2 on the two kinds of modified electrodes. In this study, the catalytic effect of haemoglobin for hydrogen peroxide (H2O2) was observed on either Hb/L-MWCNTs/Chi GCE or Hb/S-MWCNTs/Chi GCE. As can be seen from , in blank phosphate buffer, the modified electrode only gave the electrochemical behaviour of Hb, a pair of quasi-reversible anodic and cathodic peaks. When 5 mM H2O2 was added into the solution, significant increase in the reduction peak at about −0.36 V was observed, and the oxidation peak current almost disappeared simultaneously, which implied a typical electrocatalytic reduction process of H2O2. It is reasonable to believe that the catalytic peak come from the interaction between Hb and H2O2. The electrocatalytic process can be expressed by Equation (2) Citation34:
Figure 5. CVs of: (a) Hb/L-MWCNTs/Chi and (b) Hb/S-MWCNTs/Chi GCE in 0.10 M N2-saturated PBS (pH = 7.4) in the presence (solid line) and absence (dashed line) of 5 mM H2O2 at a scan rate of 100 mV s−1.
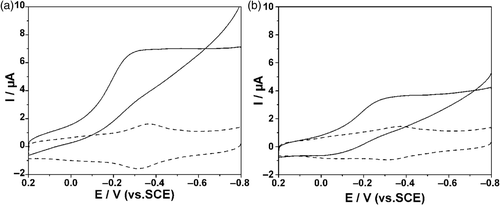
Figure 6. Amperometric responses at: (a) Hb/L-MWCNTs/Chi and (b) H/S-MWCNTs/Chi GCE upon subsequent addition of 0.1 mM H2O2 in 0.10 M N2-saturated PBS (pH = 7.4) at −0.36 V. The inset shows the calibration curves.
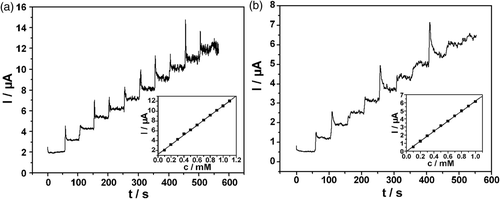
The above results indicated that ball-milled MWCNTs showed no promoted but weakened effect on the direct electrochemical behaviour of haemoglobin and corresponding electrocatalytic behaviour towards H2O2, although the MWCNTs shortened by ball milling had more edges and more defects on the walls. The results were consisted with the study of Pumera et al. Citation35 who mixed L-MWCNTs (0.5–200 µm) and S-MWCNTs (0.5–2 µm) with epoxy resin to construct CNT-epoxy composite electrodes. They found that L-MWCNTs based epoxy composite electrodes showed strong electrocatalytic activity towards NADH and hydrogen peroxide while S-MWCNTs based epoxy composites showed similar oxidation potential as graphite-epoxy composite electrode for both NADH and hydrogen peroxide. However, the explanation for the difference between electrocatalytic behaviour of long and short CNTs towards NADH was not given. Hereby, we proposed a hypothesis that in the MWCNTs/Chi composite, the electrocatalysis effects on promoting the direct electrochemistry of haemoglobin are not only related to the amount of MWCNTs active sites, but also to some other factors, such as the conductive properties affected by the dispersant which will be discussed in the following section.
3.4. Electrochemical characterisation of the MWCNTs/Chi composite
The electrochemical responses of the two kinds of MWCNTs/Chi composites modified electrodes were investigated by CV. The electrochemical reaction of the reversible ferricyanide/ferrocyanide redox couple was mainly used as a probe to evaluate the surface electrochemical reactivities between different electrodes. Such redox couple was chosen due to its wide use in the electrochemical community, which facilitates the comparison with different systems reported in the literature. Peak current and peak separation are the two main features to analyse in a cyclic voltammogram. Peak current gives us insights on the macroscopic electroactive surface area and the latter provides a measure of the heterogeneous reaction rate constant.
illustrates the cyclic voltammograms of bare GCE and modified electrodes in 5 mM [Fe(CN)6]4− with 0.1 M KCl at 100 mV s−1. Peak separations and peak currents are listed in . We can see a pair of quasi-reversible redox peaks with peak separation of 98 mV and symmetrical peak currents at bare GC electrode (curve b). Lower peak current (Ip ) and larger △Ep were observed (curve a) after the electrode was modified with 4 µL of chitosan, which was due to the chitosan membrane hindering the diffusion of ferricyanide ion towards the GCE surface. Comparing S-MWCNTs/Chi GCE and L-MWCNTs/Chi GCE with bare GCE, the peak current increased while the peak separation decreased, indicating MWCNTs had a high catalytic active surface. Furthermore, the L-MWCNTs/Chi GCE showed much higher peak current and smaller peak separation than the S-MWCNTs/Chi GCE. The increase of peak currents could be ascribed to the increase of the electrochemically active surface area. The electrochemical surface area was calculated by quantifying the peak current according to Equation (3):
Figure 7. CVs of: Chi/GCE (a), bare GCE (b), S-MWCNTs/Chi GCE (c) and L-MWCNTs/Chi GCE (d) in 5 mM [Fe(CN)6]4– with 0.1 M KCl at 100 mV s−1.
![Figure 7. CVs of: Chi/GCE (a), bare GCE (b), S-MWCNTs/Chi GCE (c) and L-MWCNTs/Chi GCE (d) in 5 mM [Fe(CN)6]4– with 0.1 M KCl at 100 mV s−1.](/cms/asset/d04803e0-c99f-4aff-8cd8-d3bb20718dab/tjen_a_655784_o_f0007g.gif)
Table 1. Peak separation and rate constants for different electrodes in 5 mM [Fe(CN)6]4– with 0.1 M KCl at 100 mV s−1.
In this equation, α represents the symmetry factor which is taken approximately as 0.5; Dred = 6.32 × 10−6 cm2 s−1 corresponds to the diffusion coefficient of the reduced species; ν = 0.1 V s−1 represents the scan rate; A the electrochemical active area and Cred = 0.05 M the bulk concentration of the electroactive species. The calculated electrochemical surface areas are listed in . In order to get quantitative insight of the electron transfer process at the MWCNTs/Chi composites and electrolyte interface, we evaluated the electron transfer rate constant from the peak separation using the Nicholson method Citation36. The resulting reaction rate constants are also listed in .
Electrochemical impedance spectroscopy (EIS) is a powerful technique for studying the interface properties of surface-modified electrodes and often used for understanding chemical transformations and processes associated with the conductive supports Citation37. The impedance spectra for the different electrodes are showed in . The semicircle located near the origin is probed by higher frequencies, which means that the dynamics of electron transfer in higher frequency range is observed and the current excited by voltage is under kinetic control. The low frequency region, where the slope of Zre versus Zim is unity, is dominated by mass transfer of the redox species to and from the interfacial region. It can be observed that the diameter of the semicircles of chitosan film (curve a) was much larger than that of bare GCE (curve b), which indicated that a film of chitosan was formed on the electrode surface, and blocked the electron transfer of [Fe(CN)6]3−/4− to some degree. The semicircle of S-MWCNTS/Chi GCE (curve c) was remarkably smaller than bare GCE (curve b) and chitosan modified GCE (curve a). This obvious change suggested that the MWCNTs accelerated electron transfer of the electrochemical probe. And the semicircle of curve a was even smaller which meant that L-MWCNTs/Chi composite had smaller resistance than S-MWCNTs/Chi composite, and thus electron transfer was easier to take place between the former electrode and electrolyte. The results were in agreement with CVs ().
3.5. Morphology of MWCNTs/Chi composite
There would be an electrostatic interaction between positively charged polycation of Chi and negatively charged MWCNTs, and it would decrease the van der Waals forces among MWCNTs bundles by wrapping the MWCNTs in polymer chains as reported previously Citation38. Comparatively speaking, a homogeneous and black suspension was obtained after L-MWCNTs and chitosan were mixed ultrasonically for more than 30 min, while it was just needed no more than 10 min for the S-MWCNTs to do it, indicating that there existed stronger interaction between the S-MWCNTs and chitosan.
It could be clearly observed in that the L-MWCNTs twisted together and fabricated a porous meshy structure. Whereas in the , the diameter of S-MWCNTs in the chitosan film was much larger than that of L-MWCNTs, suggesting that S-MWCNTs were entrapped with thick chitosan film and would block the electron transfer between electrode surface and Hb or ferricyanide/ferrocyanide redox in some way. And the darker area also proved the weaker conductive property of the S-MWCNTs/Chi composite, which was probably the reason why S-MWCNTs/Chi composite had weaker electroactivity towards Hb and ferricyanide redox.
4. Conclusions
We shortened the L-MWCNTs by ball milling method and comparatively studied the direct electrochemistry and electrocatalytic behaviour of Hb on both L-MWCNTs/Chi composite and S-MWCNTs/Chi composite modified electrodes. TEM showed the ball-milled MWCNTs were much shorter and had more open ends which were supposed to be the active sites. However, to our surprise, we found that ball-milled MWCNTs dispersed in chitosan had weakened effect on the direct electrochemistry of Hb, and the electron transfer rate constants of Hb on L-MWCNTs/Chi and S-MWCNTs/Chi composite modified electrodes in PBS were 1.08 and 0.95 s−1, respectively. We ascribed the weakened electroactivity to larger resistance of S-MWCNTs/Chi composite which was revealed by the CV and EIS in ferricyanide/ferrocyanide redox couple. Compared to L-MWCNTs, there existed stronger interaction between the S-MWCNTs and chitosan, so when MWCNTs were dispersed in chitosan, S-MWCNTs tended to be entrapped by thick film of insulated chitosan, showed by SEM images. Readers may get some directions from this article for the fabrication of electrochemical biosensors based on MWCNTs.
Acknowledgement
Financial support from the National Natural Science Foundation 232 of China (no. 20875033) is gratefully acknowledged.
References
- Wang , L and Wang , E K . 2004 . Direct electron transfer between cytochrome c and a gold nanoparticles modified electrode . Electrochem. Commun. , 6 : 49 – 54 .
- Zhao , G C , Yin , Z Z , Zhang , L and Wei , X W . 2005 . Direct electrochemistry of cytochrome c on a multi-walled carbon nanotubes modified electrode and its electrocatalytic activity for the reduction of H2O2 . Electrochem. Commun. , 7 : 256 – 260 .
- Ding , X , Hu , J and Li , Q . 2006 . Direct electrochemistry and superficial characterization of DNA-cytochrome c-MUA films on chemically modified gold surface . Talanta , 68 : 653 – 658 .
- Kumar , S A and Chen , S M . 2007 . Myoglobin/arylhydroxylamine film modified electrode: Direct electrochemistry and electrochemical catalysis . Talanta , 72 : 831 – 838 .
- Faulkner , K M , Bonaventura , C and Crumbliss , A L . 1995 . A spectroelectrochemical method for differentiation of steric and electronic effects in hemoglobins and myoglobins . J. Biol. Chem. , 270 : 13604 – 13612 .
- Fan , C H , Suzuki , I , Chen , Q , Li , G X and Anzai , J I . 2000 . An unmediated hydrogen peroxide sensor based on a hemoglobin-SDS film modified electrode . Anal. Lett. , 33 : 2631 – 2644 .
- Yu , J H and Ju , H X . 2003 . Amperometric biosensor for hydrogen peroxide based on hemoglobin entrapped in titania sol–gel film . Anal. Chim. Acta. , 486 : 209 – 216 .
- Heller , A . 1990 . Electrical wiring of redox enzymes . Acc. Chem. Res. , 23 : 128 – 134 .
- Armstrong , F A . 1990 . Bioinorganic Chemistry Structure and Bonding , Berlin/Heidelberg : Springer .
- Nassar , A E , Willis , W S and Rusling , J F . 1995 . Electron transfer from electrodes to myoglobin: Facilitated in surfactant films and blocked by adsorbed biomacromolecules . Anal. Chem. , 67 : 2386 – 2392 .
- Sun , H , Hu , N and Ma , H . 2000 . Direct electrochemistry of hemoglobin in polyacrylamide hydrogel films on pyrolytic graphite electrodes . Electroanalysis , 12 : 1064 – 1070 .
- Zhou , Y L , Hu , N F , Zeng , Y H and Rusling , J F . 2002 . Heme protein-clay films: Direct electrochemistry and electrochemical catalysis . Langmuir , 18 : 211 – 219 .
- Han , X J , Huang , W M , Jia , J B , Dong , S J and Wang , E . 2002 . Direct electrochemistry of hemoglobin in egg–phosphatidylcholine films and its catalysis to H2O2 . Biosens. Bioelectron. , 17 : 741 – 746 .
- Han , X J , Cheng , W L , Zhang , Z L , Dong , S J and Wang , E . 2002 . Direct electron transfer between hemoglobin and a glassy carbon electrode facilitated by lipid-protected gold nanoparticles . Biochim. Biophys. Acta , 1556 : 273 – 277 .
- Zhao , G , Feng , J J , Xu , J J and Chen , H Y . 2005 . Direct electrochemistry and electrocatalysis of heme proteins immobilized on self-assembled ZrO2 film . Electrochem. Commun. , 7 : 724 – 729 .
- Yin , F , Shin , H K and Kwon , Y S . 2005 . A hydrogen peroxide biosensor based on Langmuir–Blodgett technique: Direct electron transfer of hemoglobin in octadecylamine layer . Talanta , 67 : 221 – 226 .
- Zhao , Y D , Bi , Y H and Zhang , W D . 2005 . The interface behavior of hemoglobin at carbon nanotube and the detection for H2O2 . Talanta , 65 : 489 – 494 .
- Ding , X Q , Hu , J B and Li , Q L . 2006 . Direct electrochemistry and superficial characterization of DNA-cytochrome c-MUA films on chemically modified gold surface . Talanta , 68 : 653 – 658 .
- Yan , Y M , Zheng , W , Zhang , M N , Wang , L , Su , L and Mao , L Q . 2005 . Bioelectrochemically functional nanohybrids through co-assembling of proteins and surfactants onto carbon nanotubes: Facilitated electron transfer of assembled proteins with enhanced faradic response . Langmuir , 21 : 6560 – 6566 .
- Xu , Z A , Gao , N , Chen , H J and Dong , S J . 2005 . Biopolymer and carbon nanotubes interface prepared by self-assembly for studying the electrochemistry of microperoxidase-11 . Langmuir , 21 : 10808 – 10813 .
- Zhao , F , Wu , X E , Wang , M K , Liu , Y , Gao , L X and Dong , S J . 2004 . Electrochemical and bioelectrochemistry properties of room-temperature ionic liquids and carbon composite materials . Anal. Chem. , 76 : 4960 – 4967 .
- Moore , R R , Banks , C E and Compton , R G . 2004 . Basal plane pyrolytic graphite modified electrodes: Comparison of carbon nanotubes and graphite powder as electrocatalysts . Anal. Chem. , 76 : 2677 – 2682 .
- Pacios , M , del Valle , M , Bartroli , J and Esplandiu , M J . 2008 . Electrochemical behavior of rigid carbon nanotube composite electrodes . J. Electroanal. Chem. , 619–620 : 117 – 124 .
- Shelimov , K B , Esenaliev , R O , Rinzler , A G , Huffman , C B and Smalley , R E . 1998 . Purification of single-wall carbon nanotubes by ultrasonically assisted filtration . Chem. Phys. Lett. , 282 : 429 – 434 .
- Venema , L C , Wildoer , J WG , Tuinstra , H LJT , Dekker , C , Rinzler , A G and Smalley , R E . 1997 . Length control of individual carbon nanotubes by nanostructuring with a scanning tunneling microscope . Appl. Phys. Lett. , 71 : 2629 – 2631 .
- Pierard , N , Fonseca , A , Konya , Z , Willems , I , Van Tendeloo , G and Nagy , B J . 2001 . Production of short carbon nanotubes with open tips by ball milling . Chem. Phys. Lett. , 335 : 1 – 8 .
- Chi , Z H and Asher , S A . 1998 . UV resonance Raman determination of protein acid denaturation: Selective unfolding of helical segments of horse myoglobin . Biochemistry , 37 : 2865 – 2872 .
- Luo , H X , Shi , Z J , Li , N Q , Gu , Z N and Zhuang , Q K . 2001 . Investigation of the electrochemical and electrocatalytic behavior of single-wall carbon nanotube film on a glassy carbon electrode . Anal. Chem. , 73 : 915 – 920 .
- Liu , S Q , Lin , B P , Yang , X D and Zhang , Q Q . 2007 . Carbon-nanotube-enhanced direct electron-transfer reactivity of hemoglobin immobilized on polyurethane elastomer film . J. Phys. Chem. B , 111 : 1182 – 1188 .
- Cai , C X and Chen , J . 2004 . Direct electron transfer and bioelectrocatalysis of hemoglobin at a carbon nanotube electrode . Anal. Biochem. , 325 : 285 – 292 .
- Yang , J , Hu , N F and Rusling , J F . 1999 . Enhanced electron transfer for hemoglobin in poly (ester sulfonic acid) films on pyrolytic graphite electrodes . J. Electroanal. Chem. , 463 : 53 – 62 .
- Laviron , E . 1979 . General expression of the linear potential sweep voltammogram in the case of diffusionless electrochemical systems . J. Electroanal. Chem. , 101 : 19 – 28 .
- Wang , C H , Yang , C , Song , Y Y , Gao , W and Xia , X H . 2005 . Adsorption and direct electron transfer from hemoglobin into a three-dimensionally ordered macroporous gold film . Adv. Funct. Mater. , 15 : 1267 – 1275 .
- Nassar , A EF , Zhang , Z , Chynwat , V , Frank , H A and Rusling , J F . 1995 . Orientation of myoglobin in cast multibilayer membranes of amphiphilic molecules . J. Phys. Chem. , 99 : 11013 – 11017 .
- Pumera , M , Merkoci , A and Alegret , S . 2006 . Carbon nanotube-epoxy composites for electrochemical sensing . Sens Actu-B , 113 : 617 – 622 .
- Nicholson , R S . 1965 . Theory and application of cyclic voltammetry for measurement of electrode reaction kinetics . Anal. Chem. , 37 : 1351 – 1355 .
- Katz , E and Willner , I . 2003 . Probing biomolecular interactions at conductive and semiconductive surfaces by impedance spectroscopy: Routes to impedimetric immunosensors, DNA-sensors, and enzyme biosensors . Electroanalysis , 15 : 913 – 947 .
- Ajayan , P M . 1999 . Nanotubes from carbon . Chem. Rev. , 99 : 1787 – 1799 .