Abstract
In this study, methane storage capacity of granular activated carbons (GACs) and two types of multi-walled carbon nanotubes (MWCNTs) was investigated and compared. An experimental apparatus consisting a dual adsorption vessel was set up for measurement of equilibrium adsorption of methane on adsorbents using volumetric technique at pressure range of 0–50 bar at different temperatures. The first type of MWCNs has shown lower methane uptake (4.5 mmol g−1) compared to GACs (6.5 mmol g−1) at the temperature of 283.15 K and the pressure of 50 bar, while 33 mmol g−1 of methane storage capacity was achieved using the second type of MWCNTs that is much higher than methane storage on GACs at the same operating conditions. The superior uptake performance for the second type of MWCNTs can be attributed to its specific characteristics such as smaller pore size and higher pore volume. The experimental data of adsorption were almost equally well described by Langmuir, Freundlich and Sips equations to determine the model isotherms. The isosteric heat of methane adsorption on the adsorbent was calculated based on Clausius–Clapeyron and the Sips isotherm model using the experimental data at different temperatures. Results revealed that the isosteric heat of methane adsorption on MWCNTs was lower than the heat of methane adsorption on GACs. Low values obtained for isosteric heats of adsorption indicated dominance of physisorption mechanism for all adsorbents. In general, the obtained data indicated that some well-structured MWCNTs with uniform and narrow size distribution as well as higher pore volume are potential materials for methane storage and deserve further study.
1. Introduction
Many researchers attempt to find alternative fuels for transportation due to the instability in the market of petroleum-based fuels and increasingly strict environmental regulations. Among the possible fuels, natural gas (mainly composed of methane) is commonly considered as a suitable, nonpolluting energy source for the future, because it is available in large quantities in many countries and it is environmentally preferable to liquid fuels due to its cleaner combustion Citation1. Using natural gas for vehicular application depends on the storage capacity in an onboard fuel tank. Storage vessels at high pressures (20 MPa) are employed in current gas vehicles. Compared with the compressed natural gas (CNG) method, adsorbed natural gas (ANG) on a suitable microporous adsorbent offers a promising opportunity for natural gas vehicle technology Citation2. It has been thought to provide comparable methane densities as CNG at lower pressures (3.5–4 MPa) and room temperature, which presents a perspective in transport and other applications. Several studies about the use of ANG have been focused on the development of new materials, particularly carbon-based ones including molecular sieves, zeolites and especially activated carbon (AC) for its good characteristics Citation3,Citation4. The experiment of methane adsorption was done by Arami-Niya et al. Citation5 using granular AC (GAC) synthesised by impregnation of oil palm shell by ZnCl2. Among their results, 12 cm3 STP g−1 (0.5 mmol g−1) was the maximum value of methane adsorption which was achieved at the pressure of 800 mm Hg. However, this value was increased by 100% under further physical activation with CO2. Note that the authors of that research considered the pressure around atmospheric pressure and hence cannot be regarded as a good benchmark for comparison in this study. Another group of researchers carried out an investigation on the adsorption of methane on a series of microporous AC, AC fibres and superactivated carbon (SAC) at 298 K and 4 Mpa (40 bar) Citation6. The maximum storage capacity realised in that study was 13 mmol g−1 with SAC was attributed to its higher micropore volume. A comparison was made between adsorption of methane on porous metal carboxylates and other adsorbents such as AC and zeolite Citation7. The results achieved in this study confirms that the metal carboxylates had one of the highest adsorption capacities for methane with maximum 282 cm3 STP g−1 (12.6 mmol g−1) at 50 bar and 298 K. They proposed that porous adsorbent for methane adsorption should have high porosity rather than high specific adsorption sites or structures. Bagheri and Abedi Citation8 examined the adsorption of methane on corncobs-based ACs with different BET surface areas at various pressures in a volumetric adsorption apparatus. Their results showed the highest methane storage capacity of 0.27 g g−1 (17 mmol g−1) at 298 K and 500 psi (35 bar). Rios et al. Citation9 measured adsorption isotherms in a magnetic suspension microbalance to investigate storage capacity of natural gas and the effect of its composition on the amount of adsorption by utilising AC as adsorbent. The highest adsorption capacity of methane reported in this study was around 6 mmol g−1 at 35 bar and 298 K. However, they emphasised on preferential adsorption of heavier hydrocarbons and CO2 which should be considered for the evaluation of adsorption behaviour.
During recent times, carbon nanotubes have been proposed as storage nanomaterials for gas adsorption because of their exceptional properties and unique features in terms of highly uniform pore size, high surface areas and attractive surface potentials Citation10–12. Referring to interesting properties of CNTs, representation of appropriate gas storage properties by ANG method is projected. At present, investigations about gas storage on CNTs mainly focus on hydrogen storage. For other gas, such as methane and oxygen, much less attention has been received that majority of these studies were concentrated on the single wall carbon nanotubes (SWCNTs) as adsorbent Citation13–16. There are limited researches about methane adsorption on multi-walled carbon nanotubes (MWCNTs). In these works, amount of adsorbed methane on MWCNTs was investigated and results showed lower storage capacity than usual and inexpensive adsorbent such as GACs. Yulong et al. Citation17 studied the methane adsorption on MWCNTs and attained preferable mass storage capacity about 11.7%; while earlier works showed methane storage capacity on AC was higher than MWCNTs Citation18. Heretofore, study of methane storage on MWNTs by superior storage capacity in comparison with GACs has not been reported. Thus, investigation of methane storage on MWCNTs and promotion of methane adsorption properties by this novel adsorbent is an interesting research work topic.
The aim of this research work is to investigate methane adsorption capacity on two kinds of strong adsorbents (MWCNTs and GACs) by experimental methods and comparison of their storage capacity through adsorption isotherms. In addition their isosteric heat and also kinetic of adsorption are evaluated and compared.
2. Experimental
2.1. Materials and methods
Two types of MWCNTs were used to investigate methane adsorption capacity. One of them was prepared with CVD method in Research Institute of Petroleum Industry of Iran (type 1) and the other was a commercial one purchased from Alpha Nanotechnologies Company, Ltd. (China) which has been synthesised with CVD method. The granular activated carbon used in this study was a coal-based, extruded carbon with 1–2 mm diameter. It was purchased from AppliChem Company, (Germany). Methane with purity of 99.99% was purchased from Technical Gas Services, UAE.
Surface characteristic and pore structure are very important properties of adsorbents which almost all applications about adsorption are associated with them. Surface characteristics of the used adsorbents were determined with BET analysis and microscopic observations. The BET analysis was carried out based on the adsorption isotherm of N2 at 77 K measurement. Microscopic observation of the samples was conducted using SEM and TEM.
For home-made (type 1) MWCNTs with purity of about 90%, the measured specific surface area, mean pore diameter and the apparent density were 126 m2 g−1, 16 nm and 0.43 g cm−3, respectively. The total pore volume of this type of MWCNTs was 0.5140 cm3 g−1. The external MWCNTs (type 2) were 15–20 nm in outer diameter, about 4 nm in inner diameter and about 30 µm in length. Their measured SAA was 294 m2 g−1 and the apparent density only 0.06 g cm−3 (very light). The total pore volume of this type of MWCNTs was 0.6231 cm3 g−1. The purity of this kind of MWCNTs was greater than 95%. For the GACs, the measured SAA and the apparent density was 822 m2 g−1 and 0.66 g cm−3, respectively. shows the SEM and TEM images of MWCNTs (types 1 and 2). The SEM image of GACs is shown in .
Figure 1. SEM (A) and TEM (B) images of MWCNTs (type 2) and SEM (C) and TEM (D) images of MWCNTs (type 1).
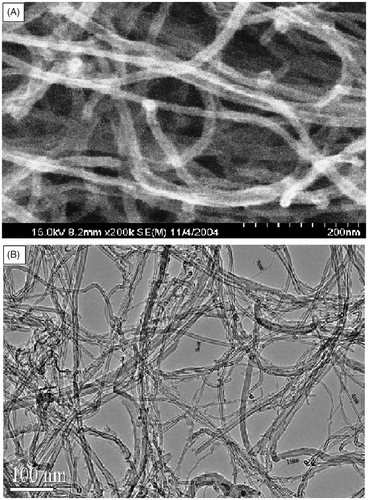
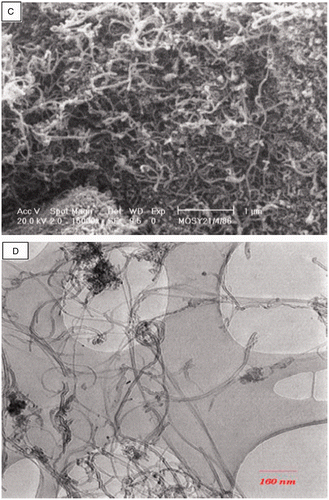
The main goal in the experimental adsorption studies is to determine the amount of material adsorbed on the adsorbent in various equilibrium conditions. In this work, first methane adsorption experiments were done with home-made MWCNTs (prepared in Research Institute of Petroleum Industry of Iran) and the results were compared with GACs. Then the commercial MWCNTs purchased from Alpha Nanotechnologies Company, Ltd. (China) were tested to investigate its capacity for methane adsorption in comparison to GACs.
2.2. Apparatus
Adsorption experiments were carried out in agreement with the volumetric method, the pressure drop due to adsorption was measured in a closed system. In this section, the volumetric apparatus (dual volume) used in this study for methane storage test by MWCNTs and GACs is presented. The gas was admitted from the gas cylinder into the pressure cell through valve 1. The temperature of pressure cell was measured by a PT100 temperature probe located in the pressure cell. The pressure in pressure cell was measured by a pressure transducer with the precision of 0.01 bar located in the pressure cell. The gas was then expanded in the adsorption cell using valves 3 and 5. The pressure of adsorption cell was measured using the second pressure transducer located in the adsorption cell. The temperature of adsorption cell was measured by a second PT100 temperature probe located in the adsorbent. The whole installation is temperature controlled by a water bath. The whole system vacuumed to 0.1 mbar by a vacuum pump before each experimental run. The procedure was completely automated by a control unit interfaced to a computer. The maximum allowable pressure in the installation was 50 bars and its working temperature range was 283–343 K. The measurement of methane-storage capacity on adsorbents was carried out with an apparatus; the experimental set up is shown in .
Due to the methane adsorption, the cell pressure reduced until the equilibrium condition was reached. The adsorbed gas amount was calculated using material balance and a suitable equation of state (SRK in this study) at equilibrium condition.
The material balance equation for calculation of adsorbed amount is shown below:
3. Theory
3.1. Adsorption isotherm models
Simulation of experimental data of methane adsorption on MWCNTs and GACs is usually performed by several isotherm models such as Langmuir, Freundlich and Sips. Such isotherm models are empirical equations that are used to describe the physical adsorption of gases on microporous solids Citation19. Applicability of common adsorption isotherm models for description of gas adsorption on SWCNT has been reported in the literature Citation20–22. Utilisation of Langmuir isotherm model has been extensively investigated in the adsorption study Citation23. In addition, Sips adsorption isotherm model was employed for simulation of experimental adsorption data in some previous works Citation20,Citation21,Citation24,Citation25. Sips isotherm was originally proposed to describe adsorption energy distribution of the sites of a solid surface. Sips isotherm reduces to Freundlich isotherm at low pressure but exhibits saturation at high pressure Citation24. The advantages of this model are its ability to fit experimental data. For the multi-layer adsorption regime, Sips equation with three parameters is expected to predict the experimental data more closely than Langmuir and Freundlich models Citation25,Citation26. For these reasons, the Sips model is predominantly used in modelling and design of adsorbents. Equations (Equation2)–(4) represent Langmuir, Freundlich and Sips equations, respectively.
3.2. Isosteric heat of adsorption
Having some knowledge about adsorption equilibrium as well as heat of adsorption is essential for proper design and operation of any gas-phase adsorption process. The isosteric heat gives an assessment of the interaction between adsorbent lattice atoms and adsorbate molecules. The latter is usually estimated from the temperature dependence of the adsorption isotherm Citation27,Citation28. Therefore, correlations that capture the correct temperature dependence over a relatively wide range are essential for the proper design and operation of gas-phase adsorption processes. For any given isotherm model, the isosteric heat of adsorption, Q st, is typically estimated using the Clausius–Clapeyron equation as follows Citation29:
The proof for derivation of Equation (Equation7) is given in Appendix.
In general, formulating isosteric heat of adsorption is possible using any isotherm by applying Clausius–Clapeyron Equation (Equation5) and temperature dependent parameters (6). An equation similar to Equation (Equation7) can be derived using other isotherms such as Langmuir and Freundlich model isotherms. The reason for the use of Sips isotherm for this purpose has been its better fit to the experimental data.
4. Results and discussions
4.1. Experimental adsorption data and isotherm model
shows the variation of methane equilibrium uptake by MWCNTs (both types) and GACs versus pressure at different temperatures. As it is clear from the presented data in , the amount of uptake achieved by home-made MWCNTs (type 1) is lower than (roughly 70%) that was adsorbed by the GACs materials. This can be attributed to higher specific area of GACs which is much more than that of MWCNTs (type 1) as well as the difference in pore size distribution. Most of GACs contain a full range of pores in which micropores (with pore diameter less than 2 nm) could well capture methane molecules with molecular size of 0.388 nm, while this kind of MWCNTs (type 1) with its mesoporous structure (average size of 16 nm) is not suitable for methane adsorption through molecular sieving. On the other hand, methane adsorption capacity on MWCNTs (type 2) is significantly greater than GACs that this high-adsorption capacity is concluded from the unique surface properties of this kind of MWCNTs such as highly uniform pore size and high pore volume. Since the average size of methane molecules is 0.388 nm, they can be easily loaded within the this kind of MWCNTs (type 2) with uniform pore size of about 4 nm, while most of total pores available in GACs are too large for methane molecules (except of micropores). In general, the amount of uptake by all adsorbents increased with an increase in the pressure and decreased with an increase in temperature. Dealing with methane adsorption on GACs and MWCNTs (type 1), it is obvious that the amount of uptake approaches to a limit when the sorbent monolayer is saturated at the pressures near 50 bars; while for MWCNTs (type 2) adsorption of methane seems still to be continued beyond this pressure. It can be concluded that MWCNTs can be a favourable adsorbent for methane storage than GACs, if it is manufactured with a suitable pore size, high pore volume and proper surface properties. Therefore, The MWCNTs (type 1) was ruled out as a candidate for the methane storage in our studies.
Figure 4. Adsorption isotherms of methane on MWCNTs (types 1 and 2) and GACs at different temperatures and pressures.
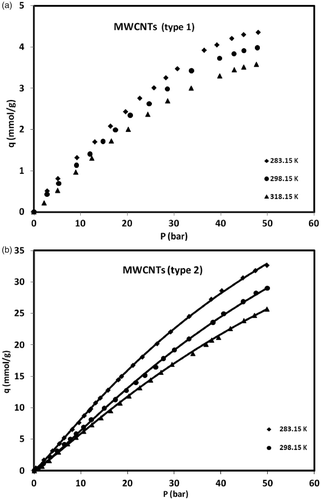
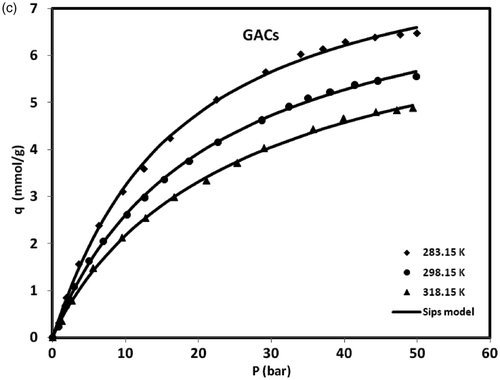
Several isotherm models were used to simulate experimental data of methane adsorption on MWCNTs and GACs. It was found that Langmuir, Freundlich and Sips isotherm models give almost perfect fit to the experimental adsorption data. The parameters of Langmuir, Freundlich and Sips isotherm models recovered from a nonlinear fit to experimental data are summarised in for MWCNTs and GACs at various temperatures. Regression coefficient values (r squares) near to unity shown in indicated a perfect match between experimental data and the fitted values. The fitted values were also shown along with experimental data for comparison in .
Table 1. Langmuir, Freundlich and Sips isotherm parameters for methane adsorption on MWCNTs and GACs in various temperatures.
4.2. Isosteric heat of adsorption
The isosteric heat of methane adsorption was calculated using Sips isotherm model. lists the optimal isotherm parameters obtained by fitting all the adsorption equilibrium data at multiple temperatures for methane. These parameters obtained using the MATLAB software (version 7.0.4) assuming a temperature independent qm value. In every case, the obtained regression coefficient, r2, is greater than 0.999. The excellent agreement between the fitted values based on Sips model and the experimental data demonstrates that this isotherm model can be confidently employed to correlate the adsorption equilibria of methane on MWCNTs. The maximum amount of methane adsorbed at saturation conditions (qm ), as predicted by the Sips isotherm model for MWCNTs (type 2) and GACs is equal to 80.92 and 8.647 mmol g−1, respectively.
Table 2. Sips isotherm parameters for methane adsorption on MWCNTs and GACs.
depicts the variation of the isosteric heat of methane adsorption with pressure determined from the temperature dependence of Sips isotherm model. Selected temperatures for plotting the curves are those at which the adsorption equilibria were measured. The values of Q stare quite satisfactory when compared with the values reported in the literature Citation31. A decreasing trend in the value of Q st with increasing pressure (loading) is obvious in . This can be explained by knowing that at lower pressures, methane molecules can come into direct contact with the adsorbent due to low surface coverage. This causes a strong interaction between adsorbate and adsorbent surfaces accompanied by high isosteric heat of adsorption. However, at high surface coverage, the weak interaction between methane and adsorbents occurs due to pore filling at high equilibrium pressure, hence the heat of adsorption decreases. As it is shown in , the isosteric heat of methane adsorption on MWCNTs (type 2) is lower than GACs and its amount drastically decreases with increasing the uptake and this trend represents that MWCNTs (type 2) adsorbents in all experiments have more active heterogeneous surface than GACs.
4.3. Adsorption kinetic study
Adsorption kinetic is important to control the process efficiency. Due to superior performance of MWCNTs (type 2) in methane adsorption, this sample was chosen for kinetic study. and show variation of methane uptake by MWCNTs (type 2) and pressure versus variation of contact time at different initial loading pressures in constant temperature of 298.15 K, respectively.
Figure 6. Effect of contact time on methane uptake amount onto MWCNTs (type 2) at different initial pressure and 298.15 K.
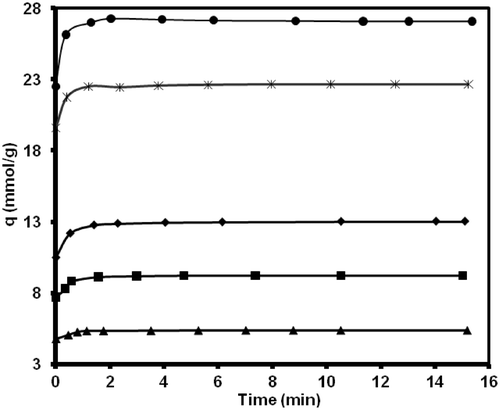
Figure 7. Effect of contact time on pressure drop of methane at different initial pressure and 298.15 K.
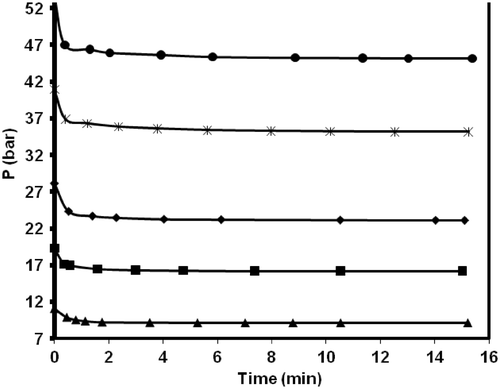
and show opposite trends, where a decrease in the adsorption cell pressure with time accompanied with an increase in methane uptake. The recorded data reveals a fast kinetics for the adsorption of methane on MWCNTs (type 2) in which most of adsorption occurs at early time (minutes) of adsorption experiments and then adsorbent is saturated by the adsorbate. The kinetic data of adsorption can be analysed by different kinetic models. Here, two models, namely pseudo-second order model and intra-particle diffusion model were used for this purpose.
4.3.1. Pseudo-second order models
The kinetics data of methane adsorption onto MWCNTs were analysed using pseudo-second order model Citation32:
Figure 8. Plot of Pseudo-second order kinetic model for methane adsorption on MWCNTs (type 2) at different initial pressure in 298.15 K.
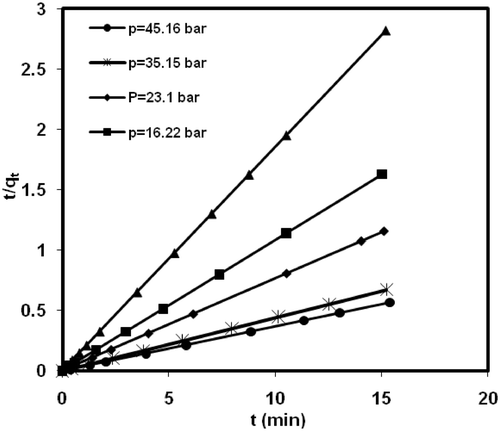
Table 3. Pseudo-second order kinetic model parameters for methane adsorption onto MWCNTs at different initial pressure.
4.3.2. Intra-particle diffusion model
Adsorption on porous solid adsorbent from gaseous phase generally involves the transport of adsorbate in bulk phase, solid phase (by either pore diffusion and/or surface diffusion), and actual adsorption step. The slowest transport step would determine the overall rate of adsorption Citation33. Adsorption kinetic data was further used to determine whether the intra-particle diffusion is the rate-limiting step and also to find the diffusion rate constant, ki (mmol g min−0.5). intra-particle diffusion model is characterised by the relationship between specific adsorption and the square root of time, according to the following equation Citation34:
5. Conclusion
In this study, the adsorption of methane at equilibrium condition on three carboneous adsorbents including two kinds of MWCNTs and GACs, was experimentally determined by volumetric technique. A series of experiments was conducted at several temperatures and pressures to evaluate the amount methane storage with these adsorbents. In comparison, the results revealed the superior methane adsorption capacity of one kind of MWCNTs over GACs; while the other demonstrated lower storage capacity of methane. GACs adsorbent contains full range of pores in which only micropores have an effective role in capture of methane molecules. Indeed, most of total pores available in GACs are too large for methane molecules (except of micropores). The performance of MWCNTs (type 1) with its mesoporous structure (average size of 16 nm) was not also satisfactory for methane adsorption through molecular sieving and showed methane uptake even lower than GACs. On the other hand, methane adsorption capacity on the MWCNTs (type 2) was considerably greater than GACs. This high adsorption capacity can be concluded from the unique surface properties of this kind of MWCNTs such as highly uniform pore size and high pore volume. Since the average size of methane molecules are 0.388 nm, they can be easily loaded within this kind of MWCNTs (type 2) with uniform pore size of about 4 nm. It was found that increasing pressure and decreasing temperature increases the amount of adsorption for all adsorbents. Subsequently the experimental data was fitted to model isotherms for prediction purposes. Results revealed that the trend of variation in the amount of methane uptake by MWCNTs and GACs follows the Langmuir and Sips isotherm models. Evaluation of methane isosteric heat of adsorption on MWCNTs and GACs from the Sips isotherm model using the thermodynamic-based Clausius–Clapeyron equation showed that decreasing temperature or increasing methane uptake decreases the isosteric heat of adsorption. There was a rapid decrease in isosteric heat of adsorption with an increase in loading of methane on adsorbents. This can be explained by knowing that at high surface coverage, interaction between adsorbate and adsorbent becomes weak due to pore filling, hence the heat of adsorption decreases. It can be concluded that MWCNTs can be a more favourable adsorbent for methane storage than GACs, if it is manufactured with a good pore size, high pore volume and proper surface properties. To the best of our knowledge, the current study revealed the potential for methane storage upon MWCNTs and deserves further study.
Acknowledgements
The authors gratefully acknowledge National Iranian Oil Products Refining and Distribution Company (NIOPRDC) for their valuable supports and funding of this research project.
References
- Tagliabue , M , Farrusseng , D , Valencia , S , Aguado , S , Ravon , U , Rizzo , C , Corma , A and Mirodatos , C . 2009 . Natural gas treating by selective adsorption: Material science and chemical engineering interplay . Chem. Energy J. , 155 : 553 – 566 .
- Parkyns , N D and Quinn , D F . 1995 . Porosity in Carbon , London : Edward Arnold .
- Zhao , G , Aziz , B and Hedin , N . 2010 . Carbon dioxide adsorption on mesoporous silica surfaces containing amine-like motifs . Appl. Energy , 87 : 2907 – 2913 .
- Huang , C C , Chen , H M and Chen , C H . 2010 . Hydrogen adsorption on modified activated carbon . Int. J. Hydr. Energy , 35 : 2777 – 2780 .
- Arami-Niya , A , Wad Daud , W MA and Mjalli , F S . 2010 . Using granular activated carbon prepared from oil palm shell by ZnCl2 and physical activation for methane adsorption . Anal. Appl. Pyrol. , 89 : 197 – 203 .
- Monge , J A , Castello , D L , Amoros , D C and Solano , A L . 2009 . Fundamentals of methane adsorption in microporous carbons . Micropor. Mesopoe. Mater. , 124 : 110 – 116 .
- Lee , J S , Jhung , S H , Yoon , J W , Hwang , Y K and Chang , J S . 2009 . Adsorption of methane on porous metal carboxylates . Ind. Eng. Chem. , 15 : 674 – 676 .
- Bagheri , N and Abedi , J . 2011 . Adsorption of methane on corn cobs based activated carbon . Chem. Eng. Res. Des. , 89 : 2038 – 2043 .
- Rios , R B , Neto , M B , Aroma , MR Jr , Torres , A EB , Azevedo , D CS and Cavalcante , C L . 2011 . Experimental analysis of the efficiency on charge/discharge cycles in natural gas storage by adsorption . Fuel , 90 : 113 – 119 .
- Bhattacharya , M , Hong , S , Lee , D , Cui , T and Goyal , S M . 2011 . Carbon nanotube based sensors for the detection of viruses . Sensor. Actuat. B Chem. , 155 : 67 – 74 .
- Otvos , Z , Onyestyak , G , Hancz , A , Kiricsi , I and Rees , L . 2006 . Surface oxygen complexes as governors of neopentane sorption in multiwalled carbon nanotubes . Carbon , 44 : 1665 – 1672 .
- Lu , C and Chiu , H . 2008 . Chemical modification of multiwalled carbon nanotubes for sorption of Zn2+ from aqueous solution . Chem. Eng. J. , 139 : 462 – 468 .
- Lithoxoos , P , Labropoulos , A , Peristeras , L D , Kanellopoulos , N , Samios , J and Economou , I G . 2010 . Adsorption of N2, CH4, CO and CO2 gases in single walled carbon nanotubes: A combined experimental and Monte Carlo molecular simulation study . J. Supercritical Fluids , 55 : 510 – 523 .
- Bekyarova , E , Murata , K , Yudasaka , M , Kasuya , D , Iijima , S , Tanaka , H , Kahoh , H and Kaneko , K . 2003 . Single-wall nanostructured carbon for methane storage . J. Phys. Chem. B , 107 : 4681 – 4684 .
- Cao , D , Zhang , X , Chen , J , Wang , W and Yun , J . 2003 . Optimization of single-walled carbon nanotube arrays for methane storage at room temperature . J. Phys. Chem. B , 107 : 13286 – 13292 .
- Murata , K , Hashimoto , A , Yudasaka , M , Kasuya , D , Kaneko , K and Iijima , S . 2004 . The use of charge transfer to enhance the methane-storage capacity of single wall carbon nanostructured carbon . Adv. Mater. , 16 : 1520 – 1522 .
- Yulong , W , Fei , W , Guohua , L , Guoqing , N and Mingde , Y . 2008 . Methane storage in multi-walled carbon nanotubes at the quantity of 80 g . Mater. Res. Bull. , 43 : 1431 – 1439 .
- Lozano-Castello , D , Cazorla-Amoros , D , Linares-Solano , A and Quinn , D F . 2002 . Activated carbon monoliths for methane storage: influence of binder . Carbon , 40 : 2817 – 2825 .
- Datsyuk , V , Kalyva , M , Papagelis , K , Parthenios , J , Tasis , D , Siokou , A , Kallitsis , I and Galiotis , C . 2008 . Chemical oxidation of multiwalled carbon nanotubes . Carbon , 46 : 833 – 840 .
- Llano-Restrepo , M . 2010 . Accurate correlation, structural interpretation, and thermochemistry of equilibrium adsorption isotherms of carbon dioxide in zeolite NaX by means of the GSTA model . Fluid Phase Equilibria , 293 : 225 – 236 .
- Somy , A , Mehrnia , M R , Delavari Amrei , H , Ghanizadeh , A and Safari , M H . 2009 . Adsorption of carbon dioxide using impregnated activated carbon promoted by Zinc . Int. J. Greenhouse Gas Control , 3 : 249 – 254 .
- Jahanshahi , M , Panahi , H , Hajizadeh , S and Moniri , E . 2008 . Boronate-containing copolymer grafted on Eupergit C as matrix for affinity chromatography . J. Chromatographia , 68 : 41 – 47 .
- Vasanth Kumar , K , Monteiro de Castro , M C , Martinez-Escandell , M , Molina-Sabio , M and Rodriguez-Reinoso , F . 2011 . Heat of adsorption and binding affinity for hydrogen on pitch-based activated carbons . Chem. Eng. J. , 168 : 972 – 978 .
- Pakseresht , S , Kazemeini , M and Akbarnejad , M . 2002 . Equilibrium isotherms for CO, CO2, CH4 and C2H4 on the 5A molecular sieve by a simple volumetric apparatus . Sep. Purif. Technol. , 28 : 53 – 60 .
- Yang , R T . 1987 . Gas Separation by Adsorption Processes , Stoneham, MA : Butterworths .
- Agnihotri , S , Rood , M J and Rostam-Abadi , M . 2005 . Adsorption equilibrium of organic vapors on single-walled carbon nanotubes . Carbon , 43 : 2379 – 2388 .
- Jakobtorweihen , S and Keil , F J . 2009 . Adsorption of alkenes and their mixtures in single-walled carbon nanotubes and bundles . Mol. Simul. , 35,01-02 : 90 – 99 .
- Walton , K , Cavalcante , C Jr and LeVan , M D . 2006 . Adsorption of light alkanes on coconut nanoporous activated carbon . Braz. J. Chem. Eng. , 23 : 555 – 561 .
- Ruthven , D M . 1999 . Principles of Adsorption and Adsorption Processes , New York : John Wiley & Sons .
- Fujiwara , A , Ishii , K , Suematsu , H , Kataura , H , Maniwa , Y , Suzuki , S and Achiba , Y . 2001 . Gas adsorption in the inside and outside of single-walled carbon nanotubes . Chem. Phys. Lett. , 336 : 205 – 211 .
- Chue , K T , Kim , J N , Yoo , Y J , Cho , S H and Yang , R T . 1995 . Comparison of activated carbon and zeolite 13X for CO2 recovery from flue gas by PSA . Ind. Eng. Chem. Res. , 34 : 591 – 598 .
- Ho , Y S and Mckay , G . 1999 . Pseudo-second order model for sorption process . Process Biochem. , 34 : 451 – 465 .
- Kumar , A , Kumar , S and Gupta , D V . 2007 . Adsorption of phenol and 4-nitrophenol on granular activated carbon in basal salt medium: Equilibrium and kinetics . J. Hazard. Mater. , 147 : 155 – 166 .
- Weber , W and Morris , J . 1963 . Kinetics of adsorption on carbon from solution . J. Sanit. Eng. Div. Am. Soc. Civ. Eng. , 89 : 31 – 60 .
- Srihari , V and Das , A . 2008 . The kinetic and thermodynamic studies of phenol-sorption onto three agro-based carbon . Desalination , 225 : 220 – 234 .
- Rodrigues , L A and da Silva , M LCP . 2010 . Thermodynamic and kinetic investigations of phosphate adsorption onto hydrous niobium oxide prepared by homogeneous solution method . Desalination , 263 : 29 – 35 .
- Fasfous , I I , Radwan , E S and Dawoud , J N . 2010 . Kinetics, equilibrium and thermodynamics of the sorption of tetrabromobisphenol A on multiwalled carbon nanotubes . Appl. Surf. Sci. , 256 : 7246 – 7252 .
- Zhu , H Y , Jiang , R , Xiao , L and Zeng , G M . 2010 . Preparation, characterization, adsorption kinetics and thermodynamics of novel magnetic chitosan enwrapping nanosized c-Fe2O3 and multi-walled carbon nanotubes with enhanced adsorption properties for methyl orange . Bioresour. Technol. , 101 : 5063 – 5069 .
Appendix
With the following temperature-dependent parameters:
We have: