Abstract
Sulphur (S), nitrogen (N)-doped TiO2/graphene oxide (GO) composites were environmentally friendly synthesised using thiourea (CS(NH2)2) as a binary-element doping reagent by a simple colloidal blending method. The S, N-doped TiO2/GO composites show higher photocatalytic degradation rates on methyl orange (MO) compared to TiO2. The average value of k (the apparent rate constant) for the S, N-doped TiO2/5%GO (k = 0.035 min−1) was found to be eight times higher than that of TiO2 grown in solution (k = 0.0038 min−1) and four times higher than that of P25 (k = 0.008 min−1). The investigation showed that the contribution of GO to the high photocatalytic activities of the composites come from its high specific surface area, oxygen-containing functional groups and large aromatic domains that were inclined to be bound by conjugated MO molecules via π–π stacking. The doping of S and N can increase the numbers of photo-generated electrons and holes, which produce more charge carriers to form reactive species, and thus promote the degradation of MO. This work could offer a route to improve the photocatalytic activities of TiO2 and facilitate their application in reality.
1. Introduction
Titanium dioxide (TiO2) is of great interest in many current and emerging areas, such as water spitting, solar energy conversion and air purification because of its high oxidative power, photostability, and nontoxicity Citation1–3. However, a severe disadvantage of this semiconductor material is its large band gap of 3.2 eV, which is too wide to absorb visible light Citation4. Another problem is the charge carrier recombination that hinders the photocatalytic activity Citation5. Therefore, developing a novel method that provides TiO2 photocatalyst with sufficient photosensitivity in the visible light region and enhances charge separation in the electron-transfer process has been one of major research efforts in recent years Citation6–9. For this purpose, many methods were investigated including doping of transition metals Citation10,Citation11, nitrogen (N) Citation12–15, sulphur (S) Citation16–18 and fluorine Citation19–22 to improve the photocatalytic activity of TiO2.
Nonmetal doping has been established as a viable method to enhance TiO2 photocatalytic activity. Particularly, the composites of TiO2 and carbon materials are being considered as potential photocatalysts in a field of purification of air and water Citation23,Citation24. The synergetic effect of carbon materials on photocatalyst enhancement of TiO2 is owed to the fact that carbon materials act as an electron sink for the hindrance of electron-hole pair recombination Citation25,Citation26. Graphene is an atomic sheet of sp2 bonded carbon atoms that are arranged into a honeycomb structure Citation27. Apart from its unique electronic properties, this material with two-dimensional plane structure has several other excellent attributes, such as large theoretical specific surface areas and high transparency due to its one-atom thickness Citation28–30. However, graphene is poorly soluble in water and polar organic solvents and easily aggregates owing to strong van der Waals force. Therefore, we use graphene oxide (GO) instead of graphene, and the oxygen-containing functional groups of GO improve its hydrophilic properties Citation31, which makes it as an excellent support to anchor inorganic nanocrystals Citation32–36.
Doping of single elements, such as N Citation12–15 and S Citation16–18 was investigated to improve photocatalytic efficiency of TiO2 due to the resulted oxynitrides and oxysulfides absorbing visible light to some extent. However, few reports were presented to dope bi- or multi-elements in TiO2 catalysts. In this work, S, N-doped TiO2/GO composites were prepared using thiourea (CS(NH2)2) as a bi-element doping reagent under different conditions, and the degradation rates on methyl orange (MO) indicated that thiourea and GO greatly improved the photocatalytic activity of TiO2.
2. Experimental technique
2.1. Materials and reagents
Nature graphite powders were obtained from Duratight Sealing product Co., Qingdao, China. Titanium tetrabutoxide (Ti(OC4H9)4), CS(NH2)2, ethanol, acetone, chloroform (CHCl3) and MO were purchased from sinopharm Chemical Reagent Co. All chemicals are analytical grade without further purification and were used as received.
2.2. Preparation of GO nanosheets
GO was prepared by oxidising natural graphite powders based on our earlier report Citation37. Firstly, a reaction flask containing a magnetic stir bar was charged with sulphuric acid (76 mL) and nitric acid (36 mL), and then cooled by immersion in an ice bath followed by stirring. Nature graphite (4 g) was added under vigorous stirring to avoid agglomeration. After the graphite powder was well dispersed, potassium chlorate (34 g) was slowly added for 30 min to avoid a rapid increase of temperature. The reaction flask was allowed to stir for 4 h at room temperature. Secondly, the mixture was slowly poured into deionised water under stirring and then filtered after stratification. Thirdly, the brown-black solutions were diluted and ultrasonicated for 40 min to further exfoliate the graphite oxide sheets, and then centrifuged at 7000 rpm for 15 min in order to remove the un-exfoliated graphite. The collected supernatant was brown-yellow GO solution with homogeneous dispersion. Finally, NaOH (6 M) was added into the GO solution with stirring, floccules were filtered and washed with ethanol until neutral. The GO powers were obtained by drying in a vacuum oven at 50°C.
2.3. Synthesis of TiO2/GO
GO was added into 400 mL distilled water under sonication for 1 h. Ti(OC4H9)4 was dropped slowly into GO solution with strongly stirring, and simultaneously 5 mL 1M nitric acid was added in order to restrain Ti(OC4H9)4 hydrolysis. Then the mixture was further stirred under 100 C until it became powder. The product was calcined in air at 200°C for 2 h to remove unreacted organic molecules. Afterwards it was again calcined at a certain temperature (it is 500°C if no given data) for 4 h under Ar flow in a tube furnace. The prepared composites were denoted as ‘TiO2/nGO(T)’, where ‘n’ was the weight percentage of GO (1.25, 2.5, 5, 7.5, 10, 12.5%) in the TiO2/GO composites, and ‘T’ was the pyrolysis temperature.
2.4. Preparation of S, N-doped TiO2/GO
GO and CS(NH2)2 were added into 400 mL distilled water under sonication for 1 h, and S, N-doped TiO2/GO samples were prepared by hydrolysis Ti(OC4H9)4 in the foregoing solution in the presence of 5 mL 1M nitric acid. The mixture was heated at 100°C till it became a powder. Then the produced powders were again calcined at 600°C for 4 h under Ar flow in a tube furnace. The resulted samples were washed with water to remove residual CS(NH2)2, then dried under vacuum at 50°C. According to this method, the S, N-doped TiO2/GO(T) with different molar ratios of thiourea:TiO2 from 1:1 to 1:10 were synthesised.
2.5. Photocatalytic experiments
A quantity of 0.2 g of the catalyst was dispersed in a 500 mL aqueous solution of MO (20 mgL−1) in a cylindrical quartz vessel. The photocatalytic reaction was carried out for 30 min under stirring in dark in order to reach adsorption equilibrium. Then the MO solution with the catalysts was exposed to the UV irradiation (300 W OCRS-K HXSEI instrument Co. The wavelength range of UV light is from 250 to 400 nm) for 2 h, and the centrifuged MO solution was taken out to perform a UV/visible absorption measurement every 20 minutes.
2.6. Fabrication and measurements of film electrodes
The composites were homogeneously mixed in ethanol (150 mg mL−1) under sonication for 30 min. The resulted slurries were spread on a 1 × 2 cm conducting glass substrate (FTO, 15 Ωm−3) with a glass rod, using adhesive tapes as spacers. The resultant films were calcined at 450°C for 2 h in Ar atmosphere to achieve well electronic contact among the particles.
The electrochemical impedance spectra (EIS) measurements were carried out on a Zahner-Zennium electrochemical workstation by using three-electrode cells. The resultant electrode served as a working electrode and an Ag/AgCl (saturated KCl) electrode as a reference electrodes. EIS was performed in the presence of a 2.5 mM k3[Fe(CN)6]/k4[Fe(CN)6] (1:1) mixture as a redox probe in 0.1 M KCl solution. The impedance spectra were recorded with the help of ZPlot/ZView software under an ac perturbation signal of 5 mV over the frequency range of 1 MHz to 100 MHz.
Photocurrent experiments also take the three-electrode cells, and the electrolyte is the 0.5 mol L−1 Na2SO4 aqueous solution. The working electrode was irradiated horizontally by a high pressure mercury lamp and a cutoff filter. The photocurrent tests of photocatalysts were measured using the electrochemical workstation.
2.7. Characterisation
The morphologies and structures of the samples were examined by transmission electron microscopy (TEM) using a FEI Tecnai G20 instrument equipped with an energy-dispersive X-ray analyzer working at 200 kV. Selected area electronic diffraction (SAED) and energy-dispersive X-ray spectrometer (EDX) were also taken on the same apparatus. X-ray powder diffraction (XRD) patterns were obtained on an X-ray diffractometer (D/Max-IIIC, Rigaku, Japan) with Cu-Kα radiation (λ = 1.5406 Å). Raman spectrum was recorded on a Laser Confocal Raman Microspectroscopy (LabRAM HR 800 UV, HPRIBA JOBIN YVON) with 632.8 nm He–Ne laser excitation. A UV2300 spectrophotometer (Techcomp, China) was used to record the UV–vis spectra of various samples. AFM images were acquired in tapping mode in air using a Digital Instrument Nanoscope IV by depositing a dilute aqueous solution of S, N-doped TiO2/5%GO on single-crystal silicon. The specific surface area of the samples was measured by conventional N2 adsorption Brunauer–Emmett–Teller (BET) method (Micromeritics Gemini 2375).
3. Results and discussion
3.1. Morphologies and structures of the S, N-doped TiO2/GO composites
shows a TEM image of the S, N-doped TiO2/5%GO composites. It can be seen that the TiO2 nanoparticles with a diameter of about 10 nm are absorbed onto the surface of GO sheets. Because of the distribution of containing oxygen groups such as carboxylic groups on the edges of GO, the TiO2 nanoparticles were inclined to accumulate and disperse along the edges and wrinkled GO sheets. The EDX spectrum (b) illustrates the presence of S, N in the composites apart from element Ti, O and C. shows an AFM image of the S, N-doped TiO2/5%GO nanosheets with different thicknesses of 1.5 and 3.5 nm, respectively. The TiO2 nanoparticles (shown by white and bright dots) were also observed on the surfaces of GO and single-crystal silicon substrate.
A serial of wide-angle XRD patterns were obtained for TiO2/5%GO (T) prepared from 400 to 700°C and S, N-doped TiO2/5%GO (T) from 400 to 800°C shown in . The strong peaks were assigned to body-centered tetragonal TiO2 expressing the main (101) diffraction peak at 2θ = 25.4° along with the (004) and (200) peaks. These signals are structural characterisation of the predominant anatase phase of TiO2. The rutile phase was also detected by the main (110) peak at 2θ = 27.2° along with (110), (101) and (111) peaks Citation27. shows the phase transformation of TiO2/5%GO (T) with temperature change. The as-prepared powders were amorphous after calcination at 150°C. From 400°C, the peak intensity of anatase phase at 2θ = 25.4° become weaker with the increase of the calcination temperature, while the peak width and intensity of the rutile phase at 2θ = 27.2° become sharper and stronger. The sample heated at 700°C shows the peak of rutile (110) only and the anatase (101) disappears. shows the XRD patterns of S, N-doped TiO2/5%GO (T). The anatase phase TiO2 could be obtained even if those samples were heated up to 700°C. Little rutile TiO2 existed, indicating that the S, N-doped TiO2 samples exhibit considerably high thermal stability and highly resistant to the rutile phase transformation Citation24. No typical diffraction peaks belonging to the GO sheets are observed in all samples, and the reason can be ascribed to the relatively low content of GO in the composites covered by the diffraction signals of TiO2.
Figure 2. XRD patterns of TiO2/5%GO (T) (a) and S, N-doped TiO2/5%GO (T) (b) calcined at different temperatures; (c) the degradation rate of MO over S, N-doped TiO2/5%GO (T) calcined at different temperatures.
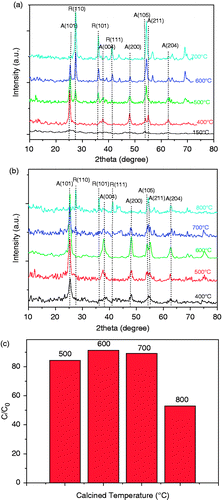
It has been known that the anatase phase of TiO2 shows better photocatalytic activity than rutile phase, and temperature is the most important factor in phase transformation. shows the degradation rate of MO over the S, N-doped TiO2/5%GO (T) composites calcined at different temperatures. It is obvious that the as-prepared catalysts have an optimal photocatalytic activity at 500°C due to its large surface area, small crystallite size and good crystallisation ().
Table 1. The surface area, energy gap and structural data of the samples.
shows the nitrogen adsorption-desorption isotherm of the as-synthesised samples. The type IV isotherm with an inflection of nitrogen-adsorbed volume at P/P 0 = 0.5 (H2 and H3 hysteresis loop according to IUPAC nomenclature) indicates the presence of well-developed mesoporosity. The differential pore size distribution based on the Barrent-Joyner-Halenda (BJH) method is shown in the inset in , which indicates that the main pore sizes of the S, N-doped TiO2/5%GO are about 6.1 nm, in agreement with the particle size determined from the TEM images, and it further confirms a uniform pore size distribution.
Figure 3. Nitrogen isotherm adsorption-desorption curves of TiO2, TiO2/5%GO (T), S, N-doped TiO2/5%GO (T) samples calcined at different temperatures. The inset shows the main pore size of the S, N-doped TiO2/5%GO (600).
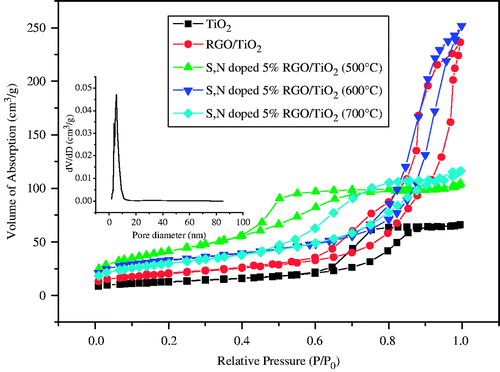
The specific surface area of the samples is measured using the BET method by N2 adsorption and desorption at 77 K. shows the physicochemical properties of TiO2/5%GO (T) and S, N-doped TiO2/5%GO (T) calcined at temperatures ranging from 500 to 700°C. The S, N-doped TiO2/5%GO (500) showed a specific surface area of 150 m2 g−1, which is three times larger than TiO2 obtained by a conventional method. While the samples calcined at 600°C and 700°C for 4 h, they still presented a comparatively high specific surface area of 116 m2 g−1 and 107 m2 g−1, respectively. The higher surface area of the samples may be attributed to the large areas of GO and TiO2 nanoparticles, and the embedding of the dopants in the TiO2 network also results in larger pore volume, pore size and surface area. Such architecture plays an important role in catalyst design for its ability to improve the molecular transport of reactants and products [33,38,39]. Adsorption on the catalyst surface area facilitates the aggregation of the reactant molecules for the photoreactions. And also, photogenerated electrons and vacancies as well as the adsorbed molecules can diffuse at the large catalyst surface, causing the photoreactions easier to happen. With the calcination temperature increasing, the surface area of the samples reduces slightly.
3.2. Photocatalytic activity of the S, N-doped TiO2/GO composites
First of all, we try to investigate the effect of GO contents of TiO2/GO composites on MO degradation rate. From the UV/visible absorption measurement, we obtained the degradation rate C/C 0 of ‘TiO2/nGO’ shown in . It is found that the photocatalytic activity of the TiO2/nGO composites was enhanced gradually with increasing the contents of the GO. When the content of GO got to 5%, the as-prepared photocatalysts had the optimal photocatalytic activity, and degraded MO by 80% in 2 h, which is almost 2.5 times as high as that of TiO2. However, the degradation rate decreased gradually with the further increasing of the GO contents. More GO lower the contact surface of TiO2 particles with the light irradiation, and thus lead to a decreased photocatalytic activity, which is still higher than that of TiO2 Citation29. So it is inferred that GO can enhance the photocatalytic activity of TiO2, and the TiO2/GO composites with 5% GO have the optimal photocatalytic efficiency. An amount of 5% was selected as the optimum amount of GO in the composites used in the following experiments.
To further understand the effect of GO on the photocatalytic activity of TiO2/nGO, the control experiments of the TiO2/5%GO and TiO2 were carried out in 20 mg L−1 aqueous solution of MO in dark for 30 min. The remaining concentration fraction of MO in the solution was obtained from UV/visible absorption. After equilibrium in the dark for 30 min, most of MO (ca. 92%) remained in the solution, and only 8% MO was absorbed on the TiO2. While for the TiO2/5%GO composites, 20% MO was absorbed on its surface. We tested the photocatalytic performance of TiO2, P25 (a well-known commercial TiO2 photocatalyst) and TiO2/5%GO. The amount of TiO2 kept the same in all these samples. The kinetics of the degradation reaction were fitted to a pseudo first-order reaction at low dye concentrations: ln(C/C
0) = k
t, where k is the apparent rate constant of photocatalytic reaction Citation31. From , the average value of k for the TiO2/5%GO (k = 0.017 min−1) was found to be ca. four times as high as that of TiO2 (k = 0.0038 min− 1) and two times as high as that of P25 (k = 0.008 min−1). The high photocatalytic activity of the TiO2/5%GO comes from GO that facilitates interfacial charge transfer and inhibits electron-hole recombination, and another possible contributing factor is that the composite has a higher BET surface area (150 m2 g−1) than TiO2 (45 m2 g−1; ). In addition, the conjugated dye molecules (MO, ) could bind to large aromatic domains on GO sheets via π–π stacking, which could favor increased reactivity.
Figure 5. Photocatalytic degradation of MO monitored as concentration versus irradiation time in the presence of TiO2, P25 and TiO2/5%GO (T).
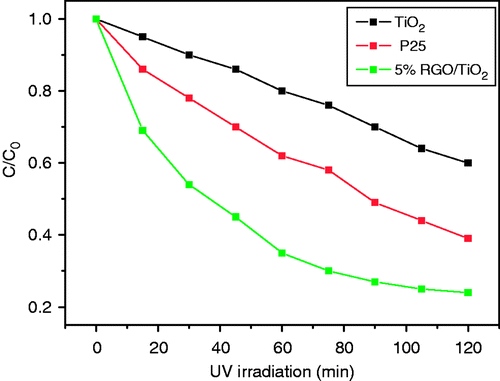
Apart from the GO content, we wonder whether the content of thiourea takes effect on the photocatalytic activities of the composites. shows the effect of thiourea contents on the photocatalytic activities of the composites. It can be seen that the composite has the best photocatalytic degradation efficiency while the molar rate of TiO2: CS (NH2)2 is 1:3, and the degradation rate of the composites (k = 0.035 min−1) was two times compared to that of TiO2/5%GO (k = 0.017 min−1). Anyway, the thiourea content hasn’t a prominent influence on the degradation rate of the TiO2/5%GO composite.
Figure 6. Influence of the thiourea content on photocatalytic degradation of MO under UV irradiation.
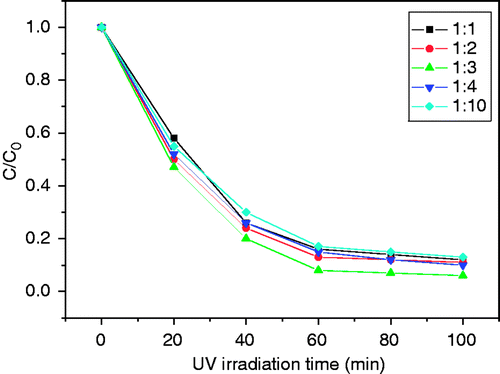
The UV/visible diffuse reflectance spectra of the samples are shown in . Compared with TiO2, the absorption edges of the TiO2/5%GO (T), and S, N-doped TiO2/5%GO (T) are found to shift to the lower-energy region. This result indicates that the narrowing of the band gap of TiO2 occurs with GO, N and S introduction, and the S, N-doped samples had the largest red shift of ca. 30–40 nm in the absorption. Further observation shows that the absorbance increases with increasing calcination temperature. The reason may be that the high-temperature calcination can induce S, N elements to be doped into the lattice of TiO2. Meanwhile, high-temperature calcination can promote the formation of the rutile phase, of which the band gap (3.0 eV) is smaller than that of anatase (3.2 eV). Therefore, the increase of calcination temperature resulted in the narrowing of the band gap. The band gap energies of all samples are given in . The results are not surprising that the direct band gap energies of the as-prepared composites are lower than that of anatase TiO2 (pH 1, 3.20 eV). S, N-doped TiO2/5%GO samples expand the wavelength response range into the visible region compared with TiO2/5%GO (T) and increased the number of photo-generated electrons and holes to participate in the photocatalytic reaction Citation34,Citation35. All these could make S, N-doped TiO2/5%GO (T) samples a better photocatalytic activity than TiO2/5%GO (T).
3.3. Photocatalytic degradation mechanism of the S, N-doped TiO2/GO composites
As shown in , the typical electrochemical impedance spectra were presented as Nyquist plots. It is observed that with the introduction of GO and element S, N, the semicircle in the plot became shorter, which indicated a decrease in the solid state interface layer resistance and the charge transfer resistance on the surface. In the TiO2/GO system, the excited electrons of TiO2 could transfer from the conduction band to GO via a percolation mechanism, and GO plays the role of an electron acceptor that accelerates the interfacial electron-transfer process from TiO2, which strongly retarding the recombination of charge carriers and thus improving the photocatalytic activity Citation36. However the S, N-doped TiO2/5%GO had a shorter semicircle than TiO2. In the S, N-doped TiO2/5%GO (T), the oxygen sites were partially replaced with nitrogen and sulphur atoms while TiO2 was simultaneously reduced. The charge imbalance caused by nitrogen, sulphur entering into the lattice of titania during heat-treatment formed oxygen vacancy which further reduced Ti4+ to Ti3+. The oxygen vacancy and Ti3+ species act as a hole trap. While the electrons and holes were generated in the initial stage of visible light illumination, the defects on the titania surface or in the bulk could suppress the recombination of electron-hole pairs and hence extend their lifetime Citation37–39.
Figure 8. (Colour online) (a) EIS changes of TiO2, TiO2/5%GO (T), S, N-doped TiO2/5%GO (T) electrodes. (b) Photocurrent response of TiO2, TiO2/5%GO (T), S, N-doped TiO2/5%GO (T).
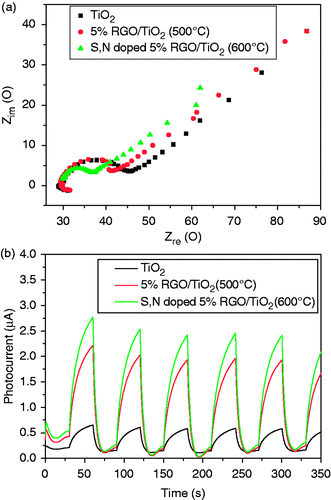
For a better understanding of charge recombination behavior in the as-prepared samples, we investigated the electron transport in sample films via to transient current. shows the photocurrents of the TiO2, TiO2/5%GO (T) and S, N-doped TiO2/5%GO (T) composites are 0.6 µA, 2.0 µA and 2.5 µA, respectively. It suggests that GO could act as an electron shuttle, thereby improving the charge separation efficiency. When the electrode is illuminated, the sudden photoinduced separation of electron-hole pairs shows up as photocurrent spike. The spike then decays as the electrons to the FTO and the holes to the electrolyte to oxidise water to form OH radicals, and a subsequent decrease in the photocurrent indicates that recombination is occurring within the film. It is observed that there is a fast and uniform photocurrent responding to each switch-on and switch-off event in both electrodes. Photoinduced hole-electron pairs separate at the interface between TiO2 and GO, electrons transfer along the plain of GO and then pass through the interface between GO and FTO to the external circuit.
4. Conclusion
S, N-doped TiO2/GO composites were synthesised using a simple environmentally friendly method. Superior adsorption and photocatalytic activity under UV were obtained on the composite system. On one hand, the results indicated the beneficial role of GO in MO photodegradation, in which GO with high specific surface area acts as an additional adsorbent for increasing adsorption of MO due to oxygen-containing functional groups. Moreover, GO can act as an electron transfer channel to reduce the recombination of the photo-generated electron holes and extend the light absorption into the visible region, leading to improved photo-conversion efficiency. On the other hand, the doping of element S and N further improves the photocatalytic activity compared to TiO2/GO, and increases the number of photo-generated electrons and holes to participate in the photocatalytic reaction. This work could provide a route to improve the photocatalysts of TiO2 and facilitate their application in reality.
Acknowledgements
This work has been supported by Ministry of Education of China (20114208110005), Hubei Provincial Department of Education (D20111002), and Wuhan Science and Technology Bureau (201271130447), China.
References
- Fujishima , A , Rao , TN and Tryk , DA . 2000 . Titanium dioxide photocatalysis . J. Photochem. Photobiol. C: Photochem. Rev. , 1 : 1 – 21 .
- Kamat , PV . 1993 . Photochemistry on nonreactive and reactive (semiconductor) surfaces . Chem. Rev. , 93 : 267 – 300 .
- Hoffmann , MR , Martin , ST , Choi , W and Bahnemann , DW . 1995 . Environmental applications of semiconductor photocatalysis . Chem. Rev. , 95 : 69 – 96 .
- Linsebigler , A , Lu , G and Yates , JT . 1995 . Photocatalysis on TiO2 surfaces: Principles, mechanisms, and selected results . Chem. Rev. , 95 : 735 – 758 .
- Lee , HJ , Leventis , HC , Moon , SJ , Chen , P , Ito , S , Haque , SA , Torres , T , Nuesch , F , Geiger , T , Zakeeruddin , SM , Grätzel , M and Nazeeruddin , MK . 2009 . PbS and CdS quantum dot-sensitized solid-state solar cells: ‘Old concepts, new results’ . Adv. Funct. Mater. , 19 : 2735 – 2742 .
- Yoo , DH , Cuong , TV , Pham , VH , Chung , JS , Khoa , NT , Kim , EJ and Hahn , SH . 2011 . Enhanced photocatalytic activity of graphene oxide decorated on TiO2 films under UV and visible irradiation . Current Appl. Phys. , 11 : 805 – 808 .
- Han , C , Pelaez , M , Likodimos , V , Kontos , AG , Falaras , P , O'Shea , K and Dionysiou , DD . 2011 . Innovative visible light-activated sulfur doped TiO2 films for water treatment . Appl. Catal. B: Environ. , 107 : 77 – 87 .
- Chen , QF , Shi , WM , Xu , Y , Wu , D and Sun , YH . 2011 . Visible-light-responsive Ag–Si codoped anatase TiO2 photocatalyst with enhanced thermal stability . Mater. Chem. Phys. , 125 : 825 – 832 .
- Menéndez-Flores , VM , Bahnemann , DW and Ohno , T . 2011 . Visible light photocatalytic activities of S-doped TiO2-Fe3+ in aqueous and gas phase . Appl. Catal. B: Environ. , 103 : 99 – 108 .
- Yuan , Z , Jia , J and Zhang , L . 2002 . Influence of co-doping of Zn(II)+Fe(III) on the photocatalytic activity of TiO2 for phenol degradation . Mater. Chem. Phys. , 73 : 323 – 326 .
- Harada , M , Sasaki , T , Ebina , Y and Watanabe , M . 2002 . Preparation and characterizations of Fe- or Ni-substituted titania nanosheets as photocatalysts . J. Photochem. Photobiol. A: Chem. , 148 : 273 – 276 .
- Asahi , R , Morikawa , T , Ohwaki , T and Taga , Y . 2001 . Visible-light photocatalysis in nitrogen-doped titanium oxides . Science , 293 : 269 – 271 .
- Morikawa , T , Asahi , R , Ohwaki , TT , Aoki , A and Taga , Y . 2001 . Band-gap narrowing of titanium dioxide by nitrogen doping . Jpn. J. Appl. Phys. , 40 : 561 – 563 .
- Yin , S , Zhang , Q , Saito , F and Saito , T . 2003 . Preparation of visible light-activated titania photocatalyst by mechanochemical method . Chem. Lett. , 32 : 358 – 359 .
- Lindgren , T , Mwabora , JM , Avendano , E , Jonsson , J , Hoel , A , Granqvist , C and Lindquist , S . 2003 . Photoelectrochemical and optical properties of nitrogen doped titanium dioxide films prepared by reactive dc magnetron sputtering . J. Phys. Chem. B , 107 : 5709 – 5716 .
- Umebayashi , T , Yamaki , T , Itoh , H and Asai , K . 2002 . Band gap narrowing of titanium dioxide by sulfur doping . Appl. Phys. Lett. , 81 : 454 – 456 .
- Ohno , T , Mitsui , T and Matsumura , M . 2002 . Photocatalytic activity of S-doped TiO2 photocatalyst under visible light . Chem. Lett. , 32 : 364 – 365 .
- Umebayashi , T , Yamaki , T , Tanaka , S and Asai , K . 2003 . Visible light-induced degradation of methylene blue on S-doped TiO2 . Chem. Lett. , 32 : 330 – 331 .
- Hattori , A , Yamamoto , M , Tada , H and Ito , S . 2003 . A promoting effect of NH4F addition on the photocatalytic activity of sol-gel TiO2 films . Chem. Lett. , 27 : 707 – 708 .
- Nukumizu , K , Nunoshige , J , Takata , T , Kondo , JN and Hara , M . 2003 . TixNOyFz as a stable photocatalyst for water oxidation in visible light (<570 nm) . Chem. Lett. , 32 : 196 – 197 .
- Yu , JC , Yu , J , Ho , W , Jiang , Z and Zhang , L . 2002 . Effects of F-doping on the photocatalytic activity and microstructures of nanocrystalline TiO2 powders . Chem. Mater. , 14 : 3808 – 3816 .
- Akhavan , O and Ghaderi , E . 2009 . Photocatalytic reduction of graphene oxide nanosheets on TiO2 thin film for photoinactivation of bacteria in solar light irradiation . J. Phys. Chem. C , 113 : 20214 – 20220 .
- Wang , F and Zhang , K . 2011 . Reduced graphene oxide-TiO2 nanocomposite with high photocatalystic activity for the degradation of rhodamine B . J. Mol. Catal. A: Chem. , 345 : 101 – 107 .
- Naik , B , Parida , KM and Gopinath , CS . 2010 . Facile synthesis of N- and S-incorporated nanocrystalline TiO2 and direct solar-light-driven photocatalytic activity . J. Phys. Chem. C , 114 : 19743 – 19782 .
- Liu , YJ , Wang , ZM , Aizawa , M , Peng , WQ and Hirotsu , T . 2009 . Nanoporous composite of carbon nanosheets and functional titania nanoparticles formed by reassembling of exfoliated graphite oxides with colloidal titania . Mater. Lett. , 63 : 260 – 262 .
- Zhang , H , Lv , X , Li , Y , Wang , Y and Li , J . 2010 . P25-graphene composite as a high performance photocatalyst . ACS Nano , 4 : 380 – 386 .
- Zhang , XY , Li , HP , Cui , XL and Lin , Y . 2010 . Graphene/TiO2 nanocomposites: Synthesis, characterization and application in hydrogen evolution from water photocatalytic splitting . J. Mater. Chem. , 20 : 2801 – 2806 .
- Manga , KK , Zhou , Y , Yan , Y and Loh , KP . 2009 . Multilayer hybrid films consisting of alternating graphene and titania nanosheets with ultrafast electron transfer and photoconversion properties . Adv. Funct. Mater. , 19 : 3638 – 3643 .
- Nguyen-Phan , TD , Pham , VH , Shin , EW , Pham , HD , Kim , S , Chung , JS , Kim , EJ and Hur , SH . 2011 . The role of graphene oxide content on the adsorption-enhanced photocatalysis of titanium dioxide/graphene oxide composites . Chem. Eng. J. , 170 : 226 – 232 .
- Wang , YJ , Shi , RS , L , J and Zhu , YF . 2010 . Significant photocatalytic enhancement in methylene blue degradation of TiO2 photocatalysts via graphene-like carbon in situ hybridization . Appl. Catal. B: Environ. , 100 : 179 – 183 .
- Liang , YY , Wang , HL , Casalongue , HS , Chen , Z and Dai , HJ . 2010 . TiO2 nanocrystals grown on graphene as advanced photocatalytic hybrid materials . Nano Res. , 3 : 701 – 705 .
- Zhang , YH , Tang , ZR , Fu , XZ and Xu , YJ . 2010 . TiO2-graphene nanocomposites for gas-phase photocatalytic degradation of volatile aromatic pollutant: Is TiO2-graphene truly different from other TiO2−carbon composite materials? . ACS Nano , 4 : 7033 – 7314 .
- Li , N , Liu , G , Zhen , C , Li , F , Zhang. , L and Cheng , HM . 2011 . Battery performance and photocatalytic activity of mesoporous anatase TiO2 nanospheres/graphene composites by template-free self-assembly . Adv. Funct. Mater. , 21 : 1717 – 1722 .
- Liu , JC , Bai , HG , Wang , YJ , Liu , ZY , Zhang , XW and Sun , DD . 2010 . Self-assembling TiO2 nanorods on large graphene oxide sheets at a two-phase interface and their anti-recombination in photocatalytic applications . Adv. Funct. Mater. , 20 : 4175 – 4181 .
- Bell , NJ , Yun , HN , Du , AJ , Coster , H , Smith , SC and Amal , R . 2011 . Understanding the enhancement in photoelectrochemical properties of photocatalytically prepared TiO2-reduced graphene oxide composit . J. Phys. Chem. C , 115 : 6004 – 6009 .
- Zhang , YP and Pan , CX . 2011 . TiO2/graphene composite from thermal reaction of graphene oxide and its photocatalytic activity in visible light . J. Mater. Sci. , 46 : 2622 – 2626 .
- Hu , HT , Wang , XB , Wang , JC , Wan , L , Liu , FM , Zheng , H , Chen , R and Xu , CH . 2010 . Preparation and properties of graphene nanosheets–polystyrene nanocomposites via in situ emulsion polymerization . Chem. Phys. Lett. , 484 : 247 – 253 .
- Zhou , M and Yu , Y . 2008 . Preparation and enhanced daylight-induced photocatalytic activity of C, N S-tridoped titanium dioxide powders . J. Hazard. Mater. , 152 : 1229 – 1236 .
- Rengifo-Herrera , JA , Mielczarski , E , Mielczarski , J , Castillo , NC , Kiwi , J and Pulgrain , C . 2008 . Escherichia coli inactivation by N, S co-doped commercial TiO2 powders under UV and visible light . Appl. Catal. B , 84 : 448 – 456 .