Abstract
This paper presents experimental and theoretical determination of the effective thermal conductivity of various base fluids and nano TiO2 composition. Ultrasonically assisted sol–gel method was used for synthesising anatase TiO2 nanoparticles and dispersing them into base fluids using sonication for the synthesis of nanofluids. It is observed that thermal conductivity enhancement is significantly higher than that of base fluid. The thermal conductivity shows an increment with the addition of nanoparticles and confirms a 22% enhancement achievable in base fluids. The effect of base fluids is a complex idea and difficult to understand; lower base fluid viscosities were supposed to contribute higher in enhancement of thermal conductivity, but another important factor; i.e. fluid nanoparticles surface interaction, nanoparticles crystal type also contributes in enhancement. In the further study, as the sonication time increases; an improvement in the thermal conductivity of nanofluids is also observed. Except water-based nanofluids, all others show reasonably good agreement with the data predicted by Bruggeman model and the prediction is in the range of 5%. This study is important since it covers base fluids with a wide range of thermal conductivity and viscosity.
1. Introduction
Nowadays, greater power output for engines, smaller microelectronic devices and cooling of relative systems have become essential for industries. Energy production and its minimal losses are challenging tasks for researchers.[Citation1] Conventional fluids, like water and ethylene glycol, are the common and the best choice as heat transfer fluids, which have relatively poor thermophysical property; hence several methods have been proposed to enhance their heat transfer performance, such as addition of microsized metal particles in base fluids,[Citation2] and thermophysical properties.[Citation1–4] It has been accepted for a long time that the solid particles suspended in base fluid offer useful advantages in industrial fluid systems, together with heat transfer fluids, lubricant fluids, and magnetic fluids.[Citation4] The main idea behind this is that the solid materials have higher thermal conductivity compared to convectional fluids such as water, ethylene glycol, and thermophilic oils; but this system possesses quick settling of particles from suspension and clogging of micro channels.[Citation5] The addition of nanoparticles to these fluids creates nanofluids, which have the capability of the next-generation heat transfer fluids, and firstly it has been proposed by Choi.[Citation6] The advantages of nanofluids over those containing less than nanosized particles (micro particles or less) are their better stability and higher thermal conductivity.[Citation7] Nanoparticles are relatively close in size to the molecules of the base fluid and thus can realise very stable suspensions with little gravitational settling over long periods of time.[Citation8] The nanofluids containing small amounts of nanoparticles have shown enhanced thermal conductivity.[Citation9–17] Generally, there are two common techniques for the preparation of nanofluids: the single-step [Citation11] and the two-step method had been used.[Citation12] As compared to the single-step method, the two-step technique gives better results for oxide nanoparticles, whereas it is less successful in metal particles. Lots of data on thermal conductivity of nanofluids are available in the scattered form. Even for the same type of nanofluids, different results are reported by different groups. The reason behind this is that the thermal conductivities of nanofluids are dependent upon various factors like the temperature of fluid, particle size, the settlement time after nanofluids preparation, and the stability of nanofluids. Stability of nanofluids plays an important role in the measurement of thermal conductivity since it affects the accuracy of the measurement. Recently, the effect of CuO nanoparticle addition in water and ethylene glycol on thermal conductivity was demonstrated. The nanoparticles of 23 nm were used as a main thermal medium for heat transfer.[Citation18]
Applications of titanium dioxide (TiO2) are: mainly as a white pigment for paints and cosmetics; as a support in catalysis; and as a photo-catalyst.[Citation19] The application and performance of it are strongly influenced by the crystalline nature, the morphology, and the particle size.[Citation20] Recently, nanocrystalline TiO2 nanoparticles were used in the preparation of nanofluids for heat transfer application. An interest in nanocrystalline TiO2 particles generated because of their especially size-related properties; and therefore, many efforts have been made on the synthesis of nanocrystalline titanium dioxide and its uses in the preparation of nanofluids.[Citation20–22]
In view of the special properties of TiO2, it was thought desirable to study the applicability of TiO2 nanoparticles in fluid as nanoparticles for heat transfer applications.
In the past research, no studies were carried out on a wide range of base fluids with the difference in thermal conductivity and viscosity simultaneously. In the present paper, a simple and research-friendly synthesis method of TiO2 nanoparticles with the results on the sonication time and concentration-dependent thermal conductivity of TiO2-based nanofluids is presented. Furthermore, the thermal conductivity was calculated using theoretical models (Maxwell and Bruggeman models) and compared with carefully measured experimental thermal-conductivity values, and the obtained deviation was discussed with possible reasons.
2. Experimental section
2.1. Reagents and chemical
All the chemicals used in this work were of analytical grade and used as received without further purification. The main precursor used for the titania nanoparticles synthesis was 99% pure titanium isopropoxide (Across Chemical, Belgium), isopropanol was used as a dilutant to precursor, ethylene glycol, paraffin oil (Fisher Scientific Chemicals) and lab synthesised double-distilled water were used as base fluids for nanofluids.
2.2. Physical characterisation
The X-ray diffraction (XRD) measurements were recorded using a (XPERT-PRO Panalytical) X-ray diffractometer equipped with a back monochromator operating at 40 kV and a copper cathode as the X-ray source (λ = 0.154 nm). The XRD patterns were recorded from 10 to 90 (2θ) with a scanning step of 0.017. Transmission electron microscopy (TEM, JEOL 2100F) was used for examining the size and morphology of the synthesised TiO2 nanoparticles. The sample was prepared by dispersing the powder TiO2 nanoparticle products in alcohol by ultrasonic treatment and keeping the suspension on a carbon film supported with copper grid.
2.3. Thermal property measurement
In the present study, the thermal conductivity was measured with the KD2 Pro thermal properties analyser (Decagon Devices, WA, USA), which operates on the transient hot wire method. The KD2 Pro consists of a handheld controller and sensors of 1.3 mm diameter and 60 mm length that should be inserted into the liquid medium. The single-needle sensor was used (KS-1). The sensor was integrated with its interior, a heating element, and a thermoresistor. It is connected to a microprocessor for controlling and conducting the measurements. The nanofluids sample was kept in a cylindrical glass container of 35 mm diameter and 80 mm height. This was necessary in order to provide a minimum of 15 mm of material parallel to the sensor in all directions.
The elimination of manual fittings of data is another advantage of KD2 Pro analyser. The calibration of the sensor was carried out every time before use by measuring the thermal conductivity of distilled water and glycerine.
2.4. Synthesis of TiO2 nanoparticles
The TiO2 nanoparticles are prepared with the sol–gel method using ultrasonication. The hydrolysis reaction between precursor solution and water with constant pH of 5 was carried out in the presence of high-intensity ultrasonication. The precursor solution was a mixture of 10 ml titanium isopropoxide, titanium isopropoxide (99% pure), and 30 ml isopropanol (99% pure) and mixed in 250 ml of double-distilled water. Constant pH water acts as reactant and hydrolysis catalyst for the reaction.
The gel preparation started when both solutions were mixed together under high-intensity ultrasonication with 20 KHz frequency and 1200 W sonicator (UP 1200 Chromtech Ltd., Taiwan). Hydrolysis reaction of titanium isopropoxide offered a turbid solution that heated up to 343 K for 18 hours. The resulting suspension was white–blue and opaque with high viscosity. The prepared precipitates were washed with ethanol and dried for 8 hours at 393 K. The reaction between titanium isopropoxide and water is very fast; and to control the size of nanoparticles, reaction is made slower with dilution of titanium isopropoxide with isopropanol. Similar synthesis process in the absence of sonication was demonstrated and presented by Mahshid.[Citation23]
2.5. Preparation of nanofluids
A measured amount of nanoparticles was mixed with water, ethylene glycol, and paraffin oil with the help of high-speed magnetic stirring and homogenised with ultrasonic processor (UP 1200 Chromtech Ltd., Taiwan) to synthesise nanofluids by the two-step method. The thermal conductivity of nanofluids was measured repetitively to ensure accuracy of the measurement. The samples were stable for few hours and no sedimentation and agglomeration of the particles in the samples were seen. To avoid the effect of micro-oscillation of nanoparticles due to ultrasonication, the thermal conductivity data were measured after 20 min of settlement time. Partial micro-oscillations of nanoparticles and fluid molecules may contribute to transport of higher thermal energy which results in enhancement of higher apparent thermal conductivity as it measured immediately after the ultrasonication.
3. Results and discussion
3.1. Physical characterisation
depicts the XRD pattern and can be readily compared to titanium dioxide (JCPDS files No. 21-1272), indicating anatase stable structure of TiO2 nanoparticles. The existence of any impurity in the compound other than titania is not observed in the average crystallite size of samples, which was calculated from the XRD. The size 5 nm of titania nanoparticles is calculated using the Scherrer formula.
represents the TEM image of a typical sample of the TiO2 nanoparticles. It shows the synthesised nanoparticle of spherical shape with a narrow size distribution. All the nanoparticles are very well separated with no clear aggregation. The diameter from the TEM image is in the range of 4–8 nm approximately.
3.2. Uncertainty calculation
The uncertainty of the experimentation was determined by the measurement errors of the variable's parameters, thermal conductivity, and weight of nanoparticles. Thermal conductivity was measured by a KD2 Pro thermal properties analyser. The weight of nanoparticles was measured by the precise electronic balance (AUW 2200 Shimadzu). The uncertainty of experimental results could be calculated as follows:(1)
The accuracy of the KD2 thermal properties analyser was 5%. The accuracy of precise electronic balance was 0.01 g. Therefore, the uncertainty of the experiment was less than 6.1%.
3.3. Effect of nanoparticles
In general, an experimental result shows that the thermal conductivity of base fluid increases with increasing vol.% of nanoparticles. shows the effect of percent variation of volume fractions of nanoparticles on the thermal conductivity of base fluids (water, ethylene glycol, and paraffin oil). It is important to note that in both the figures, nanoparticle concentration was expressed in a volume fraction of nanoparticles in the base fluids. In the figure, all the base fluids show enhancement in thermal conductivity with the addition of nanoparticles. To confirm the thermal conductivity data, repetitive experimentations were carried out.
Figure 3. The effective thermal conductivity (keff) as a function of volume fraction of nanoparticles.
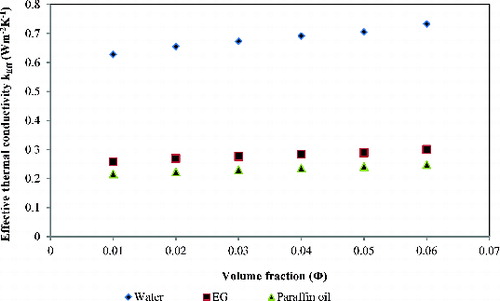
As shown in , the effective thermal conductivity of the nanofluids increases as the concentration of nanoparticles increases. The promising explanation for this effect is that the distance between nanoparticles decreases as the nanoparticle concentration increases. At the higher concentration, particle-to-particle interaction increases, which results in enhancement of thermal conductivity,[Citation4,Citation18] and this is one of the vital reasons for this effect to take place. In the case of TiO2 nanoparticles, interfacial property is also important since TiO2 and water interaction is strong and results in more –OH groups on the surface of nanoparticles. More interface attraction results in less thermal resistance. Moreover, various modes of heat transfer are responsible for this.
3.4. Effect of base fluids
In industrial application or engineering systems, pumping power is proportional to pressure drop in the system which is related to the fluid viscosity; as a result, the viscosity of base fluids is considered as critical as the thermal conductivity of base fluids. In terms of percentage enhancement in the thermal conductivity for TiO2-based nanofluids, it is found that TiO2–water shows highest percent enhancement in thermal conductivity as compared to other two nanofluids (TiO2–ethylene glycol and TiO2–paraffin oil). Our experimental results show contrary nature as demonstrated in [Citation24] on graphene oxide nanosheet. lists the viscosity and thermal conductivity of base fluids at working condition. enlists the comparison between nanofluids of 1 vol.% and 6 vol.% for the effective thermal conductivity and percent enhancement in thermal conductivity. The improvement in thermal conductivity for the TiO2–water nanofluids was found to be greater than the other two nanofluids (TiO2–ethylene glycol and TiO2–paraffin oil), while TiO2–ethylene glycol shows a lower enhancement in thermal conductivity than paraffin oil-based nanofluids. It can be said in the case of water that, on a fundamental level, the difference in viscosity between the fluids and hydrophilic nature of –OH group to TiO2 nanoparticles can account for this result. Low-viscosity fluids permit particles to more rapidly interact with one another, which leads to increase in Brownian motion of nanoparticles in fluids. This leads to more particle-to-particle interactions in a given period of time. Moreover, this results in more readily transfer of heat from the water to the particles than it was from ethylene glycol and paraffin oil to the particles.
Table 1. Thermal conductivity and kinematic viscosity data of base fluids at operation condition.
Table 2. Thermal conductivity and enhancement in the effective thermal conductivity comparison expressed as a percent for TiO2–water, TiO2–ethylene glycol, and TiO2–paraffin oil nanofluids at 1% and 6% volume fractions.
A potential reason for the enhancement could be related to the interfacial properties of the nanoparticles and base fluid. Interfacial property effects have been shown to enhance the thermal conductivity of nanofluids. For example, the fluid layer at molecular level and the thickness around the nanoparticles also influenced the thermal conductivity of nanofluids.[Citation25] The liquid at the nanoparticles surface is well structured than the bulk liquid. The well-ordered nature of layer around nanoparticles permits a higher thermal conductivity as compared to the bulk fluids. Some researchers represented this ordered layer of fluid molecules as an interfacial shell, and considered that the phonons are responsible for the efficient transfer of energy within this shell. As the thickness of the interfacial shell increases, there is a corresponding increase in the volume of the interface, which results in greater heat conduction.[Citation26] The viscosity of the fluid and the interfacial thickness of the particle–fluid interface affect the thermal conductivity of nanofluids.
The above discussion supports few conclusions on thermal conductivity and interfacial shell distance for water, ethylene glycol, and paraffin-based system; water has the highest thermal conductivity and interfacial shell distance than paraffin oil. Consequently, the effect of TiO2 nanoparticles on thermal conductivity is greater for water than for paraffin oil suspension. The ethylene glycol suspension has low thermal conductivity enhancement.
3.5. Effect of sonication time
shows the effect of ultrasonication time on the percent enhancement in thermal conductivity of suspension of nanoparticles and base fluid. As the sonication time increases, percent enhancement also occurred till the sonication time of 60 min. Nevertheless, thermal conductivity of nanofluids started decreasing after 60 min. The enhancement in thermal conductivity is due to the increase in sonication time from 0 min to 60 min. The Brownian motion of nanoparticles and intermolecular interaction between particles and fluids in the nanofluids increase after the optimum time of 60 min. Clustering of nanoparticles starts, which is accountable for the decrement in primary nanoparticles for the heat transport and thermal conductivity. It is believed that heat transfer is a surface phenomenon and the surface of nanoparticles is used for the thermal energy interaction. When the particles get agglomerated, the effective surface area to the volume ratio decreases, resulting in the reduction of the effective transfer area of particles causing a decrease in the thermal conductivity of the fluid. shows that for water-based nanofluids, effective thermal conductivity increases until the optimum time and then shows decreasing trends.
3.6. Theoretical model prediction
The Maxwell model was the first model developed to determine the effective thermal conductivity of liquid–solid suspensions,[Citation1](2) where kb is the thermal conductivity of the base fluid, kp is the thermal conductivity of the particle, and
denotes the volume fraction or the concentration of the dispersed particles.
This model is useful to statistically homogeneous and low volume-fraction liquid–solid suspensions. This model works for mixture of randomly dispersed, uniformly sized, spherical and non-interacting particles. A model related to Maxwell's model is the Hamilton and Crosser (H–C) model.[Citation27] The H–C model is a modification of Maxwell's original model, which can be used to predict the thermal conductivities of suspensions containing large, non-spherical particles.
Recently, many models have been developed to account for the enhanced thermal conductivities shown by nanofluids and compared with the basic models. One such basic model was developed by Bruggeman.[Citation28] Few researchers found good prediction of thermal conductivity of nanofluids with Bruggeman model,(3) where
(4) where
is the volume fraction of particles, and kb and kp are the thermal conductivities of the base fluid and the particle, respectively.
compares the values of the effective thermal conductivity determined experimentally with those predicted by the previously described theoretical models. The comparison for TiO2–water is shown in , whereas those for TiO2–ethylene glycol can be seen in , and TiO2–paraffin oil in . In the models, a value of base fluid thermal conductivity is determined by KD2 Pro and it is stated in and same value is used for models prediction of the effective thermal conductivity of nanofluids.
clearly illustrates that none of the models accurately predict the experimentally determined values of thermal conductivity for water-based nanofluids. Nevertheless for ethylene- and paraffin oil-based nanofluids, experimental data were nearby to model prediction. The increments in thermal conductivities predicted by the Maxwell and Bruggeman models are linear with the increments in volume fractions of nanoparticles, and their values are lower than the experimentally determined values. These models neglect the effects associated with the nanoparticles–fluid interface and the size of the nanoparticles (i.e., they consider only the thermal conductivities of the base fluid and particles). In the case of paraffin oil and ethylene glycol base nanofluids, Bruggeman model prediction of thermal conductivity has found good agreement with the experimental results. For TiO2–water nanofluids, it can be seen from the figure that the experimental thermal conductivity is about 8% higher than that of the theoretical predictions by the Maxwell model and 6% higher than that of the theoretical predictions by the Bruggeman model for a sample of 6% volume fraction nanoparticles in distilled water.
The comparison of experimental results and the theoretical predictions of these traditional models shows that the effective thermal conductivity of water-based nanofluids was under-predicted by all these models. This is probably because the previously discussed models do not account for various parameters such as particle size, Brownian motion of nanoparticles, nanolayering around nanoparticles, and the effect of nanoparticles clustering, which are important in nanofluids.
There are other factors, such as interactions between solid particles, interactions between solid particles and the base fluid, motion of the nanoparticles,[Citation22] and so on. Of course, the type of system, the technique used for thermal conductivity measurements, the method of preparation, and the properties of the nanofluids also quantitatively influence the experimental data and their difference compared to the model predictions.
4. Conclusion
Experimental investigation on the effect of sonication time and theoretical comparison of the effective thermal conductivities of water, ethylene glycol, and paraffin oil-based nanofluids containing TiO2 nanoparticles are presented. TiO2 nanoparticles having an average diameter of 5 nm with variation in volume concentrations from 1% to 6% nanofluids were prepared using a two-step method under sonication and without any surfactant addition. Water-based nanofluids provide the highest thermal conductivity enhancement of 22% as compared to other base fluids. The viscosity of the suspending fluid is an important but complex factor in the enhancement of thermal conductivity. In addition, for different nanofluids, particle agglomeration, sedimentation, interfactional properties, and affection of TiO2 toward water, and micro-oscillation due to sonication might play different roles on sonication time dependent characteristics of the thermal conductivity of nanofluids during preparation. The thermal conductivity data were generated by using a model for comparison with experimental data, which show that ethylene glycol and paraffin oil nanofluids thermal conductivity were in good agreement with those of the Bruggeman model.
Acknowledgements
The authors gratefully acknowledge financial funding from MHRD (INDIA). Dr Gokhale (NCL Pune) and his team are acknowledged for their technical assistance on TEM analysis.
References
- Maxwell JC. A treatise on electricity and magnetism. 2nd ed. Vol. 1, Oxford: Clarendon; 1881.
- Choi SUS. Degradation effects of dilute polymer solutions on turbulent friction and heat transfer behavior. J. Non-Newtonian Fluid Mech. 1992;41(3):289–307.
- Westphalen D. Heat transfer enhancement. Am. Soc Heat Refrigerating Air Cond Engineers J. 2006;48:68–71.
- Patel HE. Thermal conductivities of naked and monolayer protected metal nanoparticle based nanofluids: manifestation of anomalous enhancement and chemical effects. Appl Phys Lett. 2003;83:2931–2933.
- Choi SUS, France DM, Knodel BD. Impact of advanced fluids on costs of district cooling systems. Proceedings of the 83rd Annual Conference of the International District Heating and Cooling Association; 1992 Jun 13–17; Danvers, MA, p. 343.
- Choi SUS. Enhancing thermal conductivity of fluid with nanoparticles: developments and application of non-Newtonian flows. San Francisco, CA: ASME International Mechanical Engineering Congress & Exposition; 1995.
- Wang XQ. Heat transfer characteristics of nanofluids: a review. Int J Therm Sci. 2007;46(1):1–19.
- Zerinc SO. Enhanced thermal conductivity of nanofluids: a state-of-the-art review. Microfluid Nanofluid. 2010;8:145–170.
- Lee S. Measuring thermal conductivity of fluids containing oxide nanoparticles. Trans ASME. 1999;121:280–289.
- Lee S. Measuring thermal conductivity of fluids containing oxide nanoparticles. ASME J Heat Transf. 1999;121:280–289.
- Wang XB. Thermal conductivity of nanoparticle–fluid mixture. J Thermophys Heat Transf. 1999;13:474–480.
- Xuan Y. Heat transfer enhancement of nanofluids. Int J Heat Fluid Flow 2000;21:58–64.
- Eastman JA. Anomalously increased effective thermal conductivities of ethylene glycol-based nanofluids containing copper nanoparticles. Appl Phys Lett. 2001;78:718–720.
- Xie H. Thermal conductivity of suspensions containing nanosized SiC particles. Int J Thermophys. 2002;23:571–580.
- Wong KFV. Transport properties of alumina nanofluids. Nanotechnology 2008;19:345–702.
- Murshed SMS, Leong KC, Yang C. Investigations of thermal conductivity and viscosity of nanofluids. Int J Therm Sci. 2008;47(5):560–568.
- Li CH. Experimental investigation of temperature and volume fraction variations on the effective thermal conductivity of nanoparticle suspensions (nanofluids). J Appl Phys. 2006;99:084314–084318.
- Khedkar RS. Influence of CuO nanoparticles in enhancing the thermal conductivity of water and mono-ethylene glycol based nanofluids. Int Commun Heat Mass Transf. 2012;39:665–669.
- Gao Y. TiO2 nanoparticles prepared using an aqueous peroxotitanate solution. Ceram Int. 2004;30:1365–1368.
- Murshed SMS. Enhanced thermal conductivity of TiO2 water based nanofluids. Therm Sci. 2005;44:367–373.
- Duangthongsuk W. Measurement of temperature-dependent thermal conductivity and viscosity of TiO2-water nanofluids. Exp Therm Fluid Sci. 2009;33:706–714.
- Zhu H. A novel one-step chemical method for preparation of copper nanofluids. J Colloid Interface Sci. 2004;227:100–103.
- Mahshid S, Ghamsari SM, Askari M, Afshar N, Lahuti S. Synthesis of TiO2 nanoparticles by hydrolysis and peptization of titanium isopropoxide solution. Semiconductor Phys, Quant Electron Optoelectron. 2006;9(2):65–68.
- Yu W, Xie H, Chen W. Experimental investigation on thermal conductivity of nanofluids containing graphene oxide nanosheets. J Appl Phys. 2010;107(9):094317–094317-6.
- Keblinski P. Mechanisms of heat flow in suspensions of nano-sized particles (nanofluids). Heat Mass Transf. 2002;45:855–863.
- Moghadassi AR, Masoud Hosseini S, Henneke DE. Effect of CuO nanoparticles in enhancing the thermal conductivities of monoethylene glycol and paraffin fluids. Ind Eng Chem Res. 2010;49:1900–1904.
- Hamilton RL, Crosser OK. Thermal conductivity of heterogeneous two-component systems. Ind Eng Chem Fundamen. 1962;1(3):187–191.
- Bruggeman DAG. Berechnung verschiedener physikalischer konstanten von heterogenen substanzen I. dielektrizitatskonstanten und leitfahigkeiten der mischkorper aus isotropen substanzen [Calculation of various physical constants of heterogeneous substances III. The elastic constants of the quasi-isotropic mixing body isotropic substance]. Annalen der Physik 1935;14:636–679.