Abstract
Simple and low cost solution synthesis method was used to synthesise ZnO nanorods. Dodecyl benzene sulfonate was used to control the growth process and monodispersed nanorods with diameters in the range of 25 to 44 nm were obtained. X-ray diffraction, transmission electron microscopy and high resolution transmission electron microscopy measurements demonstrate the structure and morphology of the products. Laser power- and temperature-dependent photoluminescence (PL) experiments confirm the good ultraviolet emission characteristics. Short exciton lifetime feature relevant to thin nanorods was examined by time-resolved PL. Good optical quality and the size characteristics of the obtained products are discussed.
1. Introduction
The natural properties of direct wide band gap and large exciton binding energy make ZnO have more prospects in many application fields, particularly in terms of optical processes.[Citation1–3] One-dimensional (1D) nanostructured ZnO materials attracted more attention as they are fundamental building blocks of potential nanodevices and considerable attempts to synthesise ZnO 1D nanocrystals have been made, such as: laser vapourisation, thermal evaporation, physical vapour deposition, molecular beam epitaxy by heating Zn powders containing catalyst nanoparticles, etc. In these methods high temperatures and catalysts are needed, but growth without catalysis has also been achieved using low-temperature chemical vapour deposition, metal-organic chemical vapour deposition (for reviews, see Ref. [Citation4–9]). In addition to these techniques, it is well known that preparation of ZnO via chemical solution routes provides a promising option for large-scale production of nanomaterials which is a simpler crystal-growth technology, resulting in a potentially lower cost for ZnO-based nanodevices.[Citation10–14] Although high yield syntheses of ZnO 1D nanocrystals have been achieved, simultaneous control of their morphology, surface structure, and monodispersed distribution at comparative mild synthesising conditions is still a significant challenge. In this paper, we present an improved microemulsion method of growth of monodispersed and well-proportioned ZnO nanorods; the method presents a significant advance in bringing down the average diameter to the regime of less than 30 nm and the aspect ratio is more than 40 by using dodecyl benzene sulfonic acid sodium salt (DBS) as the modifying and protecting agent. To our knowledge, few reports have reported the successful synthesis of regular ZnO nanorods with the narrowed diameter distribution and with good optical properties by using microemulsion method.
2. Experiments
2.1. Nanocrystal synthesis
We employed the improved microemulsion method which is a low-temperature, environmentally mild, solution-based approach for the preparation of ZnO nanorods, and does not depend on the substrate used in deposition. Typical synthesis process is as follows: 20 ml of 10−3 mol/l of ZnAc2·H2O in benzene was put into a 250 ml flask, and then dodecyl benzene sulfonate was employed as surfactant with the ratio as R = Zn:DBS to 0.005 (mol ratio). Addition of hydrazine monohydrate (50% Vol. AR) with fourfold diluted by ethanol was manipulated drop-wise to the well-stirred mixture at room temperature with simultaneous vigorous agitating. In order to reach a complete reaction for getting precursors, the stirring process must last for at least 1.5 h after finishing the dropping. The resulting precursor-containing mixture was subsequently heated to boiling point of xylene for refluxing. After maintaining for 6 h, the milk-white suspension was obtained and centrifuged to separate the precipitate, which was rinsed with absolute ethanol and distilled water for several times. This was followed by evaporating volatile solvent in vacuum at 80 ℃, finally, the white products were yielded.
2.2. Physical measurements
Morphological and crystallographic properties of the as-grown samples were characterised by using transmission electron microscopy (TEM, HITACHI H-8100), high resolution transmission electron microscopy (HRTEM, JOEL 2010F), and X-ray diffraction (XRD, Rigaku D/max 2200V, Cu-Ka).
The optical properties of the produced ZnO nanorods were examined by laser power-dependent and temperature-dependent photoluminescence (PL) which were carried out by using a pulsed Nd:YAG laser (Tempest 300: 266 nm, 5 ns pulsewidth, 10 Hz pulse repetition rate). Time-resolved PL was excited by the femtosecond (fs) time-resolved Spectra Physics mode-locked Ti: Sapphire laser (266 nm, 150 fs pulse width, 1000 Hz repetition rate), and the excitation intensity was 47 μJ/cm2. A photon-counting streak camera (Hamamatsu C2909) was used for the measurements. The time resolution of the system is about 10 ps.
3. Results and discussions
3.1. Crystallographic properties and morphology
A representative XRD pattern of the as-synthesised products is shown in . All the marked diffraction peaks can coincidently be indexed to the known hexagonal standard ZnO (Joint Committee on Powder Diffraction Standard, card no. 36-1451). No characteristic diffraction peaks from other phases or impurities are detected. The extreme peak intensity along (100) direction and the comparative weak diffraction intensity of (002) show the selective directional growth.
(a) shows the TEM micrograph of a typical single nanorod and its top inset is the large scope of nanorods. (b) gives the lattice-resolved HRTEM of a selected area. It is very obvious that the produced sample has monodispersed distribution with the diameters in the scope of 25 to 44 nm and the lengths are about 1.3 μm. Lattice-resolved HRTEM image shows that the as-grown ZnO nanorods are single crystalline in nature and the crystal growth is along the [001] orientation. Furthermore, we can see the two side wall of each nanorod is smooth and straight. The uniform diameter along its entire length indicates the growth anisotropy and will be strictly maintained throughout the growth process. We suggested that surfactant DBS is beneficial to the transport and orderly stacking of the crystal growth units and the mechanism is analogous to nanorods formation by ‘oriented attachment’ of nanoparticles with the assistance of microemulsion droplets, similar to previous reports by Zhang et al.[Citation12] The droplets in microemulsion-based synthesis play an important role in confining the size and shape of the crystal nucleus at the nucleation stage and will have significant effects on the size and shape of the final products.
3.2. Photoluminescence
The power-dependent PL spectra are given in . The ultraviolet (UV) peak positions, the maximum positions of the visible emission bands and the emission intensity ratios of the UV and visible emission bands relevant to different laser power density are given in its inset, respectively.
Figure 3. Laser power-dependent PL. The insets show the UV peak positions, the maximum intensity positions of the visible emissions, and the intensity ratios of UV to visible emissions.
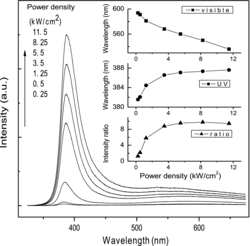
As shown in , each of the laser power-dependent PL spectrum consists of a strong UV emission band located at several nanometers more than 380 nm and a broad emission band centred in the range of 500–600 nm. The UV emission bands are the near band-edge transition of ZnO due to excitonic effects and the full width at half maximum (FWHM) is less than 20 nm at low excitation laser power. The defect-related visible emissions in ZnO may originate from various defect levels in the nanorods and will induce the so-called red/yellow/green luminescence, which is commonly attributed to radiative recombination of oxygen vacancy defects, surface defects, etc.[Citation15–17] From the inset in , we observe redshift of the UV peak from 381.6 to 387.6 nm due to laser heat effects with increasing the laser power, which is relevant to crystal lattice vibration and the interaction between electron and phonon. While, in the visible region, the maximum positions of the visible emission bands persist blueshift. The centre of visible emission band changes from about 590 nm to about 530 nm when the excitation power density increases from 0.25 to 11.5 kW/cm2. The blueshift of the visible emission band with the increase of laser power implies that the emissions are likely from donor–acceptor (D–A) pairs because one of the characteristics of D–A recombination is a shift to higher energy as the excitation intensity increases.[Citation18,19] It may be induced by the shift of carrier position from deep level state to shallow level state which is caused by heating activation, and the increase of combine energy makes the visible emission bands persist blueshift.
In addition, we observe from the ratio curve that the relative intensity of the UV emission to that of the visible emission is increased as the laser power increased, and at last tends to be a constant value, nearly about 10. This may owe to the effective exciton PL in the synthesised ZnO nanorods at high excitation energy due to the increase of the exciton density, and the nonradiative transition probability relevant to green emission will increase due to the laser heat effect. However, it should be noted that as the excitation intensity increases, the exciton density will come to a saturated value.[Citation3,Citation20,Citation21] The competition result is that the ratio of the UV emission intensity to that of the visible emission tends to be a constant value.
Temperature-dependent PL spectra of the ZnO nanorods are presented in . The UV emissions of free exciton shift from 368 to 380 nm as the temperature increased from 20 to 300 K due to gap shrinkage effect,[Citation22] and the FWHM broadened from 4.5 to 20 nm mainly due to the exciton–phonon scattering process.[Citation23] The PL intensity of the near band emissions decreases rapidly with the increase of temperature mainly due to thermal quenching.[Citation24] Only markedly enhanced near-band-edge UV PL and rarely defect-related green emission were observed in all the temperature-dependent PL spectra, which demonstrate the high crystal quality of our products. We carry out a curve-fitting analysis of the temperature dependence of the free exciton transition energy by Varshni's formula [Citation25,26]: , where
is comparable to the bandgap energy at 0 K,
represents the
limit of the gap entropy
and
is approximately the Debye temperature at low temperature. The fitting parameters are
= 3.385,
= 7.12 × 10−4,
= 740 K. The curve plotted by the formula closely fits the experimental values, as shown in the inset in . These results are in reasonable agreement with the previous reports.[Citation25]
3.3. Time-resolved photoluminescence
The exciton lifetime is an important parameter related to material quality and device performance that can be measured by time-resolved PL spectroscopy, as shown in . All the measurements were done at room temperature. Generally, the PL decay rate of a semiconductor can be analysed by double exponential fitting: . Here
and
are the fast decay time and the slow decay time, respectively. A1 and A2 are the decay constants. Normally, the fast decay most probably comes from the effective non-radiative recombination, while the slowly decaying component is related to the radiative lifetime of the free exciton. Our time-resolved PL result shows that the decay fits well to a biexponential function with a fast component 20 ps and a slower component 260 ps. Non-radiative recombination gives rise to a fast relaxation of the excited carriers, while the slow decay term is usually due to the radiative recombination of free or localised excitons. The fast decay term dominant in our sample is induced by the effective non-radiative recombination, i.e. the capture of excitons and trapping of carriers by deep centres at defects and/or impurities. Size dependence of time-resolved PL spectra of ZnO nanorods has been investigated and it was found that the decay time decreases as the rod size decreases, which was attributed to the increase in radiative rate of exciton-polaritons.[Citation27] Additional sources of the non-radiative recombination may be caused by Auger effect and multiphonon scattering, etc.[Citation28]
4. Conclusions
We have successfully synthesised single crystalline ZnO nanorods by using an improved microemulsion method. The result shows that DBS as the modifying and protecting agent plays a key role to control the microstructures of ZnO nanorods. In general, nanostructures prepared by solution methods typically exhibit yellow–orange luminescence, while significant improvements of the UV-to-visible emission ratios have been observed in our products, which means that the defect density is relatively low including both the internal and the surface of our products. The different evolution process of the UV emission part and visible emission part with the change of laser power and temperature are examined and analysed, and the time-resolved PL shows the size effect of the produced products. The high quality of the synthesised ZnO nanorods indicates further potential applications in UV nanodevices.
Acknowledgements
C.P. Li thanks the financial support of National Natural Science Foundation of China under grant no. 51272285 and the ‘Twelfth Five-Year’ Science and Technology Research Project of Jilin Provincial Department of Education under grant no. 2013-520.
References
- Özgür Ü, Alivov YaI, Liu C, Teke A, Reshchikov MA, Doğan S, Avrutin V, Cho S-J, Morkoç H. A comprehensive review of ZnO materials and devices. J Appl Phys. 2005;98:041301-1–041301-103.
- Huang MH, Mao S, Feick H, Yan HQ, Wu YY, Kind H, Weber E, Russo R, Yang PD. Room-temperature ultraviolet nanowire nanolasers. Science 2001;292:1897–1899.
- Djuršić AB, Ng AMC, Chen XY. ZnO nanostructures for optoelectronics: material properties and device applications. Prog Quantum Electron. 2010;34:191–259.
- Klingshirn C, Fallert J, Zhou H, Sartor J, Thiele C, Maier-Flaig F, Schneider D, Kalt H. 65 years of ZnO research – old and very recent results. Phys Status Solidi B. 2010;247(6):1424–1447.
- Wang ZL. Zinc oxide nanostructures: growth, properties and applications. J Phys: Condensed Matter. 2004;16:R829–R858.
- Heo YW, Norton DP, Tien LC, Kwon Y, Kang BS, Ren F, Pearton SJ, LaRoche JR. ZnO nanowire growth and devices. Mater Sci Eng R 2004;47(1):1–47.
- Sun XH, Lam S, Sham TK, Heigl F, Jürgensen A, Wong NB. Synthesis and synchrotron light-induced luminescence of ZnO nanostructures: nanowires, nanoneedles, nanoflowers, and tubular whiskers. J Phys Chem B. 2005;109:3120–3125.
- Wang XL, Yang F, Yang W, Yang X. A study on the antibacterial activity of one-dimensional ZnO nanowire arrays: effects of the orientation and plane surface. Chem Commun. 2007;42:4419–4421.
- Fang F, Zhao DX, Zhang JY, Shen DZ, Lu YM, Fan XW, Li BH, Wang XH. Growth of well-aligned ZnO nanowire arrays on Si substrate. Nanotechnology 2007;18(23):235604–235612.
- Lv YZ, Li CP, Guo L, Wang QX, Wang RM, Xu HB, Yang SH, Ai XC, Zhang JP. Nanostructured stars of ZnO microcrystals with intense stimulated emission. Appl Phys Lett. 2005;87:163103-1–163103-;3.
- Zhang J, Sun LD, Jiang XC, Liao CS, Yan CH. Shape evolution of one-dimensional single-crystalline ZnO nanostructures in a microemulsion system. Crystal Growth Des. 2004;4:309–313.
- Guo L, Ji YL, Xu HB, Simon P, Wu ZY. Regularly shaped, single-crystalline ZnO nanorods with wurtzite structure. J Am Chem Soc. 2002;124:14864–14865.
- Fang X, Li JH, Zhao DX, Li BH, Zhang ZZ, Shen DZ, Wang XH, Wei ZP. Structural and photoluminescence properties of aligned Sb-doped ZnO nanocolumns synthesized by the hydrothermal method. Thin Solid Films. 2010;518(20):5687–5689.
- Fang X, Li JH, Zhao DX. Phosphorus-doped p-type ZnO nanorods and ZnO nanorod p-n homojunction LED fabricated by hydrothermal method. J Phys Chem C. 2009;113(50):21208–21212.
- Vanheusden K, Warren WL, Seager CH, Tallant DR, Voigt JA, Gnade BE. Mechanisms behind green photoluminescence in ZnO phosphor powders. J Appl Phys. 1996;79:7983–7990.
- McCluskey MD, Jokela SJ. Defects in ZnO. J Appl Phys. 2009;106(7):071101–071113.
- Dutta S, Chattopadhyay S, Sarkar A, Chakrabarti M, Sanyal D, Jana D. Role of defects in tailoring structural, electrical and optical properties of ZnO. Prog Mater Sci. 2009;54(1):89–136.
- Zheng MJ, Zhang LD, Li GH, Shen WZ. Fabrication and optical properties of large-scale uniform zinc oxide nanowire arrays by one-step electrochemical deposition technique. Chem Phys Lett. 2002;363:123–128.
- Zacks E, Halperin A. Dependence of peak energy of pair-photoluminescence band on excitation intensity. Phys Rev B 1972;6:3072–3075.
- Xiong GN, Xu L, Liu JY, Li L, Yu H, Zhu H. Study of luminescent materials under high energy excitation. J Chin Rare Earth Soc. 2001;19(6):494–496.
- Shi WS, Cheng B, Zhang L, Samulski ET. Influence of excitation density on photoluminescence of zinc oxide with different morphologies and dimensions. J Appl Phys. 2005;98(8):083502–083506.
- Yang CL, Wang JN, Ge WK, Guo L, Yang SH, Shen DZ. Enhanced ultraviolet emission and optical properties in polyvinyl pyrrolidone surface modified ZnO quantum dots. J Appl Phys. 2001;90(9):4489–4493.
- Zhang XT, Liu YC, Zhi ZZ, Zhang JY, Lu YM, Shen DZ, Xu W, Fan XW, Kong XG. Temperature dependence of excitonic luminescence from nanocrystalline ZnO films. J Luminescence. 2002;99:149–154.
- Youn CJ, Jeong TS, Han MS, Kim JH. Optical properties of Zn-terminated ZnO bulk. J Cryst Growth. 2004;261(4):526–532.
- Li JW, Yang LW, Zhou ZF, Chu Paul K, Wang XH, Zhou J, Li LT, Sun CQ. Bandgap modulation in ZnO by size, pressure, and temperature. J Phys Chem C. 2010;114:13370–13374.
- Shan W, Walukiewicz W, Iii AgerJW, Yu KM, Zhang Y, Mao SS, Kling R, Kirchner C, Waag A. Pressure-dependent photoluminescence study of ZnO nanowires. Appl Phys Lett. 2005;86(15):153117-1–153117-3.
- Matsumoto T, Kato H, Miyamoto K, Sano M, and Zhukov EA. Correlation between grain size and optical properties in zinc oxide thin films. Appl Phys Lett. 2002;81(7):1231–1233.
- Li CP, Guo L, Wu ZY, Ren LR, Ai XC, Zhang JP., Lv YZ, Xu HB, Yu DP. Photoluminescence and time-resolved photoluminescence of star-shaped ZnO nanostructures. Solid State Commun. 2006;139:355–359.