ABSTRACT
A highly fluorescent solid state Schiff base compound (DBD) was synthesised using a green, solventless and fast approach in 30 sec. DBD shows almost no emission in THF solution, while it emits strong fluorescence in both dispersed nano-aggregates in the solution and as a powder, through a phenomenon known as aggregation-induced emission. Organic nanoparticles of DBD were prepared in aqueous solution using the nanoreprecipitation method with and without stabilizers. The nanoparticles exhibited strong, blue emission when the non-ionic surfactant, like triton X-100, was used as a stabilizer, and strong green emission when no surfactant or the cationic surfactant, like CTAB as a stabilizer was used. Using atomic force microscopy and dynamic light scattering, the sizes of the nanoparticles were found to be around 75 nm when prepared without surfactant, 50 nm when prepared using triton X-100, and 30 40 nm when prepared using CTAB. DBD nanoparticles show an emission maximum at 527 nm (pure green) in the cases of CTAB and no surfactant, while 400 nm emission is observed when triton X-100 is used. Finally, for practical applications, the new type of highly fluorescent organic nanoparticles (DBD) were chosen for on/off fluorescence switching nano sensor for detecting organic vapour.
1. Introduction
New materials with new unique properties are highly sought in the fields of nanomaterials and nanotechnology, as new properties can lead to new applications. Among the nanomaterials, fluorescence nanomaterials are of great interest for many technological applications, as they can be used as probe, sensors, tags and for bioimaging.[Citation1–3] Organic nanoparticles are a relatively new type of nanoparticle which has potential for such applications, because they are easily prepared, have properties that can be flexibly tailored using a variety of fluorophores with different characteristics, and they can be prepared in aqueous media, which enables their use in physiological conditions.[Citation4–8]
Forster and Kasper discovered that the concentration of a luminophore has a dramatic effect on emission, and it quenches the emission at high concentration, due to the formation of aggregates, in a process known as aggregation-caused quenching (ACQ),[Citation9,Citation10] in which the excited states of the aggregates decay via non-radiative routes. However, using of concentrated solution may not be satisfactory for practical applications; like bioprobes and bioimaging, as with increasing concentration, sensitivity is decreased, due to quenching ACQ.[Citation11] The same phenomenon happens in solid state, in which there is no solvent, and the luminophore molecules are in close proximity to one another. ACQ was a major obstacles of highly emission in solid state was one of the obstacles in higher concentration of the emitters due to quenching, until 2001. Tang reported a series of silole-based molecules that show no emission in solution but emit strongly when they aggregate, in a phenomenon called aggregation-induced emission (AIE).[Citation12–15] Tang et al. attributed the AIE effect to the inhibition of a radiationless deactivation route via restriction of intramolecular rotation.
Park et al.,[Citation16] one year later, came to almost same conclusion by preparation of 1-cyano-trans-1,2-bis(4_-methylbiphenyl)-ethylene (CN-MBE). CN-MBE is non-emissive in the solution, but in nanoparticles, it shows an aggregate-induced enhancement of emission.
Such findings have practical implications, enabling researchers to take advantage of the formation of aggregates, rather than avoiding it. Nanoparticles that show AIE and have efficient fluorescence may find many applications, like bioassays, bioimaging and quantitative analysis.[Citation17–22]
AIE has been seen in many ‘complicate’ organic structure, however, it has not been observed more often for small molecules, which led us to examine small molecules, like simple Schiff bases, using simple, green and fast synthetic methods. Moreover, interest in Schiff base or imine group synthesis has flourished for many years, largely due to its synthetic versatility. Schiff bases have a broad spectrum of applications, including industrial applications as a photostabilizer and as inhibitors for the photodegradation of polymers [Citation23]; and biological applications in areas such as anticancer, anti-HIV, antibacterial and antifungal.[Citation24] Conventional methods of Schiff base preparation possess some inherent impediments including long reaction time, tiresome work-up procedures and harsh conditions, while a solventless method is able to overcome all those drawbacks.[Citation25,Citation26]
In this work, solventless synthesis is used as a green, economical and fast approach to prepare the new Schiff base fluorophore N1,N4-bis(4-(dimethylamino)benzylidene)benzene-1,4-diamine (DBD), as shown in . The compound DBD produces no emission in solution, but it shows very strong emission in aggregated form (or solid state). The physical and chemical properties of the fluorphores and nanoparticles are characterised, and an organic vapour sensor based on fluorescence on/off switching is described as well.
2. Experimental section
2.1. Synthesis of DBD
shows the synthetic scheme of DBD molecule. 1,4-Phenylenediamine (0.005 mol, 0.54 g) and 4-(dimethylamino)benzaldehyde (0.01 mol, 1.49 g) were dissolved in a minimum amount of hot methanol to produce solutions 1 and 2, respectively; and two drops of glacial acetic acid were added to solution 2. Followed by mixing both solutions with vigorous stirring until a yellow orange solid is obtained in 30 sec. The reaction progress was monitored by thin layer chromatography (TLC), using methanol: chloroform (1:9) as a solvent. The product was recrystallised from methanol to produce 93.4% yield. m.p. 286—287 °C; Rf = 0.66 ; IR (KBr) ѵmax(cm−1) = 2854 (C H aliphatic), 1595 (C = N), 1553 and 1525 (C = C aromatic), 1363 (C H bending), 1178 (C N); 1H-NMR (300 MHz, CDCl3, 25 C° ,TMS): δ = 8.39 (s, 1H; -CH = N), 7.85 (d, 2H; -N = CH C = CH), 7.16 (d, 2H; CH = C N CH3), 6.78 (d, 2H; CH = C N = CH), 3.09 (s, 6H; 2 CH3); MS (70 eV): m/z (%) for C24H26N4: 370 (100%) [M+], 355 (23%) [C23H23N4+], 239 (33%) [C15H17N3+], 185 (35%) [C12H16N2+], 105 (13%) [C7H7N+] and 77 (9%) [C6H5+].
2.2. Preparation of DBD nanoparticles
The bottom-up strategy, nanoreprecipitation, was used for fabrication of fluorescent organic nanoparticles in aqueous medium.[Citation5] A colloidal solution of DBD nanoparticles was prepared in an aqueous medium in the presence and absence of 1% of each surfactant. 300 μL of 1 mM of DBD/THF solution was injected rapidly with vigorous stirring to 10 mL of H2O (or H2O/surfactant) solution. The resultant nanoparticle solution was kept in dark place and at room temperature. The nanoparticles in water and in CTAB are stable for more than a month, while in triton X-100 is stable for hours.
2.3. Characterisation
Absorption spectra were recorded using a UV-Visible spectrometer (Agilent Technologies Cary UV-Vis, Santa Clara, CA, USA) and steady-state photoluminescence spectra were recorded on fluorescence spectrophotometer (Agilent Technologies, Cary Eclipse Fluorescence Spectrophotometer, Santa Clara, CA, USA). Size of the particles were measured based on dynamic light scattering principle using (DLS, Brookhaven 90 plus). Atomic force microscopy (AFM) images were taken using an AFM in contact mode (Bruker, multimode 8, Germany). Sample preparation for AFM was as follows: some drops of the freshly prepared DBD nanoparticle solution were put on the surface of a glass slide and left at room temperature to dry. Zeta potentials were measured using Zeta sizer (NanoBrookZetaPlus, Brookhaven, NY, USA).
3. Results and discussion
Schiff base of DBD was prepared within 30 sec with a high yield, using solventless method which is known as solid state synthesis, such a fast and green method has an advantage over other methods for more time saving and environment friendly approach. All the identifications have been used in order to identify the chemical structure. However, the mass spectra (ESI-MS) of the Schiff base exhibited a molecular ion peak at (m/z) 370, indicating the formation of the Schiff base. Many molecular ion fragments peak appeared as illustrated in , which represent the most stable fragments.
DBD in solution does not emit any fluorescence when is shined with UV light source, which may be attributed to intramolecular rotation in the solution, while when nanoparticles from DBD are formed, it emits strong emission, and this is what known as AIE.[Citation14,Citation15] AIE luminogens are opposite to ACQ fluorophores, which the higher the concentration in AIE luminogens lead to higher the emission intensity, consequently, lead to more potential applications of the luminogens.
3.1. Size and morphology
AFM and DLS were used to obtain the size and morphology of the formed nanoparticles. Based on the AFM image, shown in , the size of the DBD nanoparticles in water, without surfactant, DBD/H2O, is about 75 nm. DBD/H2O is stable for almost a month with any noticeable flocculation or agglomeration, as confirmed by its UV-Vis and fluorescence measurements. Zeta potential measurement of DBD/H2O was about −25 mV (Supporting Information). In order to decrease the size of the nanoparticle and increase monodispersity, two common surfactants were used, triton X-100 and CTAB. Triton X-100 showed the opposite effect, decreasing the stability of the nanoparticles (zeta potential was −19 mV, Supporting Information) and increasing the size of the nanoparticles to around 90 100 nm. the acidity of triton X-100 might affect the stability and accelerate the particle agglomeration. However, CTAB reduces the size of nanoparticles to 50 nm, and the particles have better stability (more than one month) and enhanced luminescence as well.
3.2. Spectroscopy
AIE was observed when DBD in THF solution was mixed with water. shows a photograph of the non-emissive solution of DBD in THF and the highly emissive nanoparticles in water. Photophysical characterisation of DBD organic nanoparticles was performed using steady-state absorption and photoluminescence spectroscopy. shows the absorption spectra for DBD in THF solution, and as nanoparticles in aqueous media. summarizes the spectroscopic data of DBD as nanoparticles and in solution.
Figure 4. (a) Photograph showing DBD in solution (dissolved in THF) and DBD as nanoparticles (in aqueous solution). (b) Normalised absorption spectra of DBD in THF solution and as nanoparticles with and without surfactants. (c) Normalised photoluminescence spectra. DBD in THF gives almost no emission. (d) Comparing emission spectra of DBD/H2O in HAC to DBD/H2O triton.
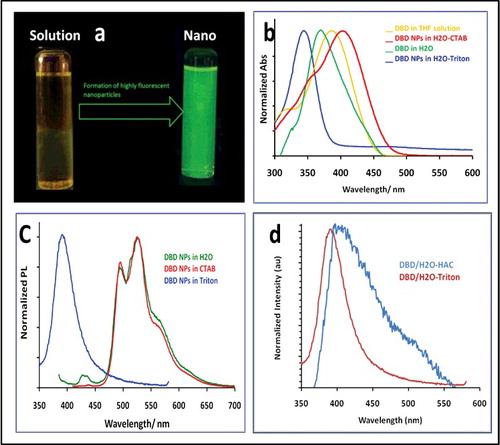
The absorption spectrum of DBD in THF is centred at 385 nm (yellow solution, ). The nanoparticles in solution show different behaviour, and up to our knowledge, we have observed unseen phenomena of size-dependent optical properties. DBD nanoparticles in just water, DBD/H2O (when there is no surfactant in solution) shows an absorption spectrum with maximum wavelength at 375 nm ((a)), the nanoparticles solution absorption spectrum was 10 nm blue-shifted comparing with DBD in solution, such a blue shift usually assigned for H-aggregates, based on Kasha's model of exciton theory.[Citation27] Both DBD/H2O and DBD/H2O–CTAB nanoparticles solutions give a well-resolved photoluminescence spectra at 495 and 527 nm with a wavelength maxima at 527 nm ((b)). The absence of emission in the solution and strong emission in aggregate form indicates that the molecules experience a restricted intermolecular rotation in the aggregated state. In case of DBD/H2O–CTAB, the absorption spectrum at 400 nm which is red-shifted by 10 nm relative to that of DBD in solution, and the strong photoluminescence at 525 nm, suggest that DBD/H2O–CTAB forms J-type aggregates. The planarised conjugated backbone and the restricted molecular rotation in the J-aggregates are possible origin of the largely emissions.[Citation28]
A change in the optical properties of organic nanoparticles can also come from the particle size-dependent intermolecular interactions between chromospheres and/or change of lattice state, due to the change of surface area, i.e. not from the Mie scattering.[Citation29] However, the excitation and absorption spectra for all the nanoparticles are not exactly superimposable, which happens when a sole species exists in different forms in the ground state phenomena that have been seen in aggregates.[Citation30] These factors complicate the interpretation of the optical properties of the organic nanoparticles.
This trend is different in DBD/H2O–triton, the absorption spectrum shows a maximum at 347 nm, which is blue-shifted by 50 nm relative to that of DBD in solution. However, the emission centred at 400 nm, which represents a 53 nm stokes shift. DBD/H2O triton is less stable and shows low emission intensity. Both findings, the blue shift and low quantum yield are features of H-aggregates, based on Kasha's model.[Citation31] Photophysical properties of nanoparticles are very influenced by surface chemistry of the particles.[Citation32,Citation33] The blue shift of DBD/H2O-triton could also be attributed to the mild acidic properties of triton solution. To test this, the DBD/H2O particles were prepared in acetic acid, HAc(pH ∼6). The emission maximum of DBD/H2O–HA was shifted from 525 to ∼400 nm, i.e. similar to the emission spectrum of DBD/H2O triton ((d)). Therefore, the surface structure plays a substantial role in determining the emissions of the different DBD nanoparticles.
4. Application as organic vapour sensor
The enhancement of fluorescence through the AIE effect in the absence of solvent was used to prepare an organic vapour sensor utilising on/off fluorescence switching.[Citation34–36] When illuminated by 365 nm UV light, DBD nanoparticles on a TLC plate exhibit bright green emission, due to AIE, when there is no organic vapour. Exposing the plate to chloroform vapour visibly decreases the emission intensity (). The effect is reversible, making DBD a suitable material for an organic vapour sensor.
5. Conclusions
In this work, a green and economical, solventless method was described for the preparation of a Schiff base molecule that does not produce emission in the solution, but shows strong emission upon aggregation. The nanoparticles in aqueous solution produce strong emission and are stable for a month in the presence of CTAB. Based on the high fluorescence of the nanoparticles in the solid state, an organic vapour sensor was proposed. The high stability and emission intensity of the DBD nanoparticles in water make them good candidates for bioimaging and bioprobes.
01_DBD_Suppl_mat_KMO.docx
Download MS Word (1 MB)Acknowledgments
The authors would like to thank Genome Center at Koye University for their help with the fluorescence measurements. Thanks to Mr Omed Al-Jaff for helping with AFM measurements.
Disclosure statement
No potential conflict of interest was reported by the authors.
Table 1. Photophysical behaviour of DBD in solution and DBD nanoparticles.
References
- Burda C, Chen X, Narayanan R, et al. Chemistry and properties of nanocrystals of different shapes. Chem Rev.2005;105:1025–1102.
- Murray CB, Kagan CR, Bawendi MG. Synthesis and characterization of monodisperse nanocrystals and close-packed nanocrystal assemblies. Annu Rev Mater Sci. 2000;30:545–610.
- Peng X, Wickham J, Alivisatos AP. Kinetics of II-VI and III-V colloidal semiconductor nanocrystal growth: “Focusing” of size distributions. J Am Chem Soc. 1998;120:5343–5344.
- Horn D, Rieger J. Organic nanoparticles in the aqueous phase—theory, experiment, and use. Angew Chem Int Ed. 2001;40:4330—4361.
- Kasai H, Nalwa HS, Oikawa H, et al. A novel preparation method of organic microcrystals. Jpn J Appl Phys. 1992;31:L1132.
- Kasahi H, Nalwa HS, Okada S, et al. In handbook of nanostructured materials and nanotechnology. New York (NY): Academic Press; 2000.
- Seko T, Ogura K, Kawakami Y, et al. Excimer emission of anthracene, perylene, coronene and pyrene microcrystals dispersed in water. Chem Phys Lett. 1998;291:438–444.
- Li S, He L, Xiong F, 1998. Enhanced fluorescent emission of rrganic nanoparticles of an intramolecular proton transfer compound and spontaneous formation of one-dimensional nanostructures. J Phys Chem B. 2005;108:10887–10892.
- Valeur B. In Molecular fluorescence, principles and applications. Wiley-VCH Verlag GmbH; 2001. Chapter 4, ISBN 3-527-60024-8 (Electronic).
- Kim S, Ohulchanskyy TY, Pudavar HE, et al. Organically modified silica nanoparticles co-encapsulating photosensitizing drug and aggregation-enhanced two-photon absorbing fluorescent dye aggregates for two-photon photodynamic therapy. J Am Chem Soc. 2007;129:2669–2675.
- Borisov SM, Wolfbeis OS. Optical biosensors. Chem Rev. 2008;108:423–461.
- Luo J, Xie Z, Lam JWY, et al. Aggregation-induced emission of 1-methyl-1,2,3,4,5-pentaphenylsilole. Chem Commun. 2001:1740–1741.
- Tang BZ, Zhan X, Yu G, et al. Efficient blue emission from siloles. J Mater Chem. 2001;11:2974–2978.
- Hong Y, Lam JWY, Tang BZ. Chem Commun. Aggregation-induced emission: phenomenon, mechanism and applications. 2009:4332–4353.
- Hong Y, Lam JWY, Tang BZ. Aggregation-induced emission. Chem Soc Rev. 2011;40:5361–5388.
- An BK, Kwon SK, Jung SD, et al. Enhanced emission and its switching in fluorescent organic nanoparticles. J Am Chem Soc. 2002;124:14410–14415.
- Zhang X, Wang K, Liu M, et al. Polymeric AIE-based nanoprobes for biomedical applications: recent advances and perspectives. Nanoscale. 2015;7:11486–11508.
- Mahtab F, Hong Y, Liu J, et al. Fabrication of fluorescent silica nanoparticles hybridized with AIE luminogens and exploration of their applications as nanobiosensors in intracellular imaging. Chem Eur J. 2010;16:4266–4272.
- Tong H, Hong Y, Dong YQ, et al. Color-tunable, aggregation-induced emission of a butterfly-shaped molecule comprising a pyran skeleton and two cholesteryl wings. J Phys Chem B. 2007;111:2000–2007.
- Mahtab F, Yu Y, Lam JWY, et al. Fabrication of silica nanoparticles with both efficient fluorescene and strong magnetization and exploration of their biological applications. Adv Funct Mater. 2011;21:1733–1740.
- Zhang X, Zhang X, Tao L, et al. Aggregation induced emission-based fluorescent nanoparticles: fabrication methodologies and biomedical applications. J. Mat Chem B. 2014;2:4398–4414.
- Zhang X, Zhang X, Wang S, et al. Surfactant modification of aggregation-induced emission material as biocompatible nanoparticles: facile preparation and cell imaging. Nanoscale. 2013;5:147–150..
- Ebrahimi HH, Hadi JS, Al-Ansari HS. A new series of Schiff bases derived from sulfa drugs and indole-3-carboxaldehyde: synthesis, characterization, spectral and DFT computational studies. J Mol Struct. 2013;1039:37–45.
- Li L, Li Z, Wang K, et al. Design, synthesis, and biological activities of aromatic gossypol Schiff base derivatives. J Agric Food Chem. 2014;62:11080–11088.
- Tanaka K, Toda F. Solvent-free organic synthesis. Chem Rev. 2000;100:1025–1074.
- Schmeyers J, Toda F, Boy J, et al. Quantitative solid–solid synthesis of azomethines. J Chem Soc Perkin Trans. 1998;2:989–994.
- Kasha M, Rawls HR, El-Bayoumi MA. The exciton model in molecular spectroscopy. Pure Appl Chem. 1965;11:371–392.
- Silva GL, Ediz V, Yaron D, et al. Experimental and Computational investigation of unsymmetrical cyanine dyes: understanding torsionally responsive fluorogenic dyes. J Am Chem Soc. 2007;129:5710–5718.
- Xiao D, Xi L, Yang W, et al. Size-tunable emission from 1,3-diphenyl-5-(2-anthryl)-2-pyrazoline nanoparticles. J Am Chem Soc. 2003;125:6740–6745.
- Valeur B B. Molecular fluorescence: Principles and applications. Weinheim: Wiley-VCH Verlag GmbH; 2001.
- Spano FC. Excitons in conjugated oligomer aggregates, films, and crystals. Ann Rev Phys Chem. 2006;57:217–243.
- Jameson DM. In Introduction to fluorescence. Taylor & Francis Group, LLC; 2014.
- Alexander P, Demchenko E. In: Alexander P, editor. Advanced fluorescence reporters in chemistry and biology II, molecular constructions, polymers and nanoparticles. Berlin:Springer-Verlag; 2010. Chapter 1.
- Wan Q, Wang K, Du H, et al. A rather facile strategy for the fabrication of PEGylated AIE nanoprobes. Polym Chem. 2015;6:5288–5294.
- Wan Q, He C, Wang K, et al. Preparation of ultrabright AIE nanoprobes via dynamic bonds. Tetrahedron. 2015;71:8791–8797.
- Wan Q, Wang K, He C, et al. Stimulus responsive cross-linked AIE-active polymeric nanoprobes: fabrication and biological imaging application. Polym Chem. 2015;6:8214–8221.