ABSTRACT
Green synthesised nickel nanoparticles (NiGs) using Ocimum sanctum leaf extract have been stabilised with L-arginine (Arg–NiGs) and used as gene transfer vector for bacterial transformation and as catalyst for hydrolytic dehydrogenation. Effective binding of L-arginine with NiGs was confirmed by characterisation of Arg–NiGs. L-arginine coating on NiGs prevented the agglomeration of NiGs and reduced the size of the nanoparticles. Synthesised Arg–NiGs was conjugated with green fluorescent protein (GFP) inserted pUC 18 (pDNA–Arg–NiGs) and employed for transforming non-competent Escherichia coli DH5 α. Transformation efficiency of pDNA–Arg–NiGs was found to be higher (3.8 × 106 µg–1 DNA) than the CaCl2-mediated method (2.9 × 105 µg–1 DNA). Fluorescence micrograph of transformants showed enhanced uptake and transformation of pDNA–Arg–NiGs by bacterial cells. Electrophoretic analysis of expressed GFP confirmed that pDNA could retain its functional activity even after binding with Arg–NiGs. This biocompatible, efficient and economical method could be exploited for transforming non-competent bacterial cells. Arg–NiGs catalysed hydrolytic dehydrogenation of ammonia borane (AB) generated 198 mL of hydrogen in 8 min with 128 mg AB and 10 mL of catalyst at 35 °C. The hydrogen generation rate of Arg–NiGs catalysed AB dehydrogenation was determined to be 24.8 mol H2 min–1 with activation energy (Ea) of 34.05 kJ mol–1 and turnover frequency (TOF) of 12.30 mol H2 (mol Ni) –1 min–1. Arg–NiGs retained 89% of its initial catalytic activity even after 10 recycles, indicating its high durability in catalytic reactions. Kinetic studies of AB dehydrogenation by Arg–NiGs show the first-order kinetics with respect to catalyst concentration and zero-order kinetics with respect to AB concentration. This efficient and durable catalyst could be applied in hydrolytic dehydrogenation of AB for effective hydrogen storage.
Highlights
Functionalisation and stabilisation of green synthesised nickel nanoparticles with L-arginine.
Arg–NiGs as gene transfer vector for enhanced transformation of non-competent Escherichia coli DH5α.
Arg–NiGs as catalyst for enhanced hydrolytic dehydrogenation of ammonia borane.
Catalytic activity of durable Arg–NiGs relies on temperature and catalyst concentration.
1. Introduction
Nanoparticles are typically smaller than biomolecules and can provide unprecedented interactions with biomolecules both on the surface and inside the cell.[Citation1] Nanoparticles have been widely used in drug and gene delivery due to its unique physical and chemical properties.[Citation2] Devising efficient method for delivery of DNA vectors into Escherichia coli is utmost importance in cloning experiments involving construction of cDNA and genomic libraries.[Citation3] An efficient method can reduce the probability of losing DNA fragments during the transformation process.[Citation4] Recently, synthesis of polymeric nanoparticles from chitin and its derivatives have also found to possess many biomedical and environmental applications.[Citation5] But the metal and metal oxide nanoparticles were considered as promising tool for DNA and protein carriers.[Citation6]
Nickel nanoparticles have numerous advantages for the assembly of supramolecular complexes due to their magnetic and electrical conductivity properties.[Citation7] Nickel nanoparticles can efficiently capture histidine tagged protein and the isolated protein from nickel associate retains their structural and functional properties.[Citation8] Oligo nucleotides can bind reversibly with light coating on nickel nanoparticles through ionic and coordination bond formation.[Citation8] Transition metal nanoparticle catalysts due to their novel structure, electronic properties and magnetic performances are considered to be a favourable option.[Citation9] There is an increasing interest for use of first-row transition-metal-based nano catalysts, such as Fe, Co, Ni, etc., to catalyse the hydrolytic dehydrogenation reactions.[Citation10] Hydrogen, a globally accepted clean and eco-friendly fuel, needs a promising approach to develop a compact, safe and affordable means for its storage due to its high gravimetric and volumetric density.[Citation11] Ammonia borane (AB) is widely used as a safe storage medium for H2 due to the high capacity in ambient atmosphere, at room temperature and in the presence of a suitable catalyst; one mole of AB hydrolysis can generate three moles of hydrogen.[Citation12] Efficiency of transition-metal-based nano catalysts highly depends on the stability of the nanoparticles because of their high tendency to form agglomerates and their extreme reactivity towards water and oxygen.[Citation10] Therefore, finding suitable and effective protection agents to exert the potent catalytic ability of metal nanoparticles in aqueous solution or even in air is still a challenge to be faced.[Citation10] A general solution to stabilise or protect the metal nanoparticles is to protect them with some outer materials and the organic protecting compounds have relatively weak binding interaction with the nanoparticles which is vital for the catalytic application where surface catalytically active sites are urgently needed.[Citation13] Amino acids interact with surface of nanoparticles through the carboxyl groups and side chains which are exposed to the exterior.[Citation14] Arginine has side-chain functional groups that provide active group for interaction with enormous variety of biological molecules and ligands.[Citation15] Synthesis of magnetic nanoparticle with amino-acid coating could be done in a one pot aqueous reaction without any organic solvents.[Citation15] The processes of plant-mediated nanoparticle synthesis were significantly suitable for large-scale synthesis.[Citation16] Plant-mediated synthesis of nanoparticles were widely used in pharmaceutical and biomedical applications due to eco-friendly and cost-effectiveness.[Citation17] Ocimum sanctum is well known for its anti-stress, antioxidant, hepatoprotective, immunomodulating, anti-inflammatory, antibacterial, antiviral, antifungal, antipyretic, antidiuretic, antidiabetic, antimalarial and hypolipidemic properties.[Citation18] Escherichia coli DH5α is not naturally competent and can be made competent by various techniques like CaCl2 treatment and heat shock.[Citation19] Many techniques have been tried to improve the competence of E. coli. Recent approaches include glutathione-functionalised gold nanoparticles to mediate delivery of plasmid DNA into bacteria.[Citation20]
With the above considerations, we have synthesised and used L-arginine stabilised green synthesised nickel nanoparticles (Arg–NiGs) for transfer of green fluorescent protein (GFP) gene inserted pUC 18 into non-competent E. coli DH5α and for catalytic dehydrogenation of AB. The gene transfer efficiency and catalytic nature of Arg–NiGs were determined by bacterial transformation and kinetic studies, respectively. Transformation efficiency of pDNA–Arg–NiGs and expression of GFP in non-competent E. coli DH5α was assessed by fluorescence microscopy and electrophoretic analysis, respectively. Biocompatibility of Arg–NiGs was also checked by the growth curve of E. coli. The catalytic efficiency of Arg–NiGs was confirmed by determining activation energy (Ea), turnover frequency (TOF) and the rate of dehydrogenation reaction.
2. Experimental
2.1. Materials
Lyophilised cultures of E. coli DH5α was procured from Microbial Type Culture Collection (MTCC), Indian Institute of Microbial Technology, Chandigarh, India. Luria Bertani (LB) medium was purchased from HiMedia Laboratories, Mumbai, India. Vector pUC18 and GFP gene were purchased from Genei, Bengaluru, India and all the other chemicals were purchased from Merck, New Delhi, India.
2.2. Synthesis and characterisation of L-arginine functionalised nickel nanoparticles
Ocimum sanctum leaf extract was synthesised according to our previous study.[Citation21] L-arginine functionalised NiGs were synthesised as reported by Alireza et al.[Citation15] with slight modifications. 2 mM Ni(NO3)2 was dissolved in 20 mL of O. sanctum leaf extract and stirred for 30 min at 60 ºC. After 30 min, 1 mM L-arginine (dissolved in 20 mL of O. sanctum leaf extract) was added to the above reaction solution and stirred for 3 h at 50 °C. L-arginine functionalised NiGs was recovered by centrifugation at 5000 rpm for 15 min, washed three times with distilled water and the resultant precipitate was dried in an oven at 40 °C overnight. The synthesised L-arginine functionalised NiGs were stable for 3 months without agglomeration.
Optical property, binding property, crystalline phase and morphology of Arg–NiGs and NiGs were characterised by ultraviolet–visible spectroscopy (UV−vis), Fourier transform infrared spectroscopy (FTIR), X-ray diffraction (XRD) and transmission electron microscope (TEM), respectively, as described in our previous study.[Citation21]
Thermal stability of both NiGs and Arg–NiGs was performed by thermogravimetric (TG) analysis using Shimadzu TGA-Q500 instrument. About 4−6 mg sample was used for each operation and heated from room temperature to 550 °C at a heating rate of 10 °C min–1 under nitrogen atmosphere (50 mL/min).[Citation22]
2.3. Preparation of recombinant plasmid
Recombinant plasmid was constructed by inserting GFP gene into pUC 18 plasmid as described earlier by Tang et al.[Citation23] with minor modifications. The pUC18 vector was dephosphorylated to reduce the probability of self-relegation. The plasmid pUC18 was digested with Bam H1 and mixed with 2.5 U phosphatase followed by addition of 1× Tris buffer (100 mM NaCl, 50 mM Tris-HCl, 10 mM MgCl2, 1 mM DTT, pH 7.9, 25°C). The contents were incubated at 37 ºC for 1.5 h and then deactivated at 65 ºC for 20 min. 90 fmol of GFP gene was ligated into 30 fmol of dephosphorylated pUC18 vector with 1 µL T4 DNA ligase in ligase buffer at 37 ºC for 16 h. The ligated sequence of GFP–pUC18 was confirmed with 1% agarose gel electrophoresis in which DNA sample was dissolved in Trisborate buffer (89 mM Tris base, 2.5 mM disodium EDTA, and 8.9 mM boric acid) and mixed with 5 µL tracking dye (bromophenol blue (0.07%), and glycerol (33%) in water). Samples were electrophoresed at 120 V for 2 h and the DNA bands were visualised after staining with ethidium bromide (0.4 µg mL–1).
2.4. Preparation of pDNA and Arg–NiGs conjugate
pDNA and Arg–NiGs conjugate was prepared as reported by Olga et al.[Citation20] Briefly, pDNA–Arg–NiGs conjugate was prepared in suspensions containing 5 µg of Arg–NiGs, 0.1 µg of pDNA and 25% of glycerol in a total volume of 20 µL followed by incubation for 3 h with gentle occasional stirring. The integrity of pDNA in prepared suspensions was evaluated in 1% agarose gel electrophoresis. The synthesised pDNA–Arg–NiGs conjugate was stable under 0.01 M phosphate buffer for 7 days.[Citation8]
2.5. Transformation of non-competent Escherichia coli by pDNA–Arg–NiGs
Transformation of non-competent E. coli was carried out as described previously by Saptarshi and Keka.[Citation24] 1 µL of pUC18 DNA (from stock of 1 ng L–1) was mixed with 50 µg mL–1 Arg–NiGs for 2 h at 37 °C. Escherichia coli DH5α was cultured on LB broth to 105 colony forming units (cfu) per mL (OD 600 nm = 0.1) and 1 mL culture was centrifuged at 1000 rpm for 2 min. Supernatant was discarded and 20 µL of pDNA–Arg–NiGs was added to the pellet followed by 980 µL LB broth and incubated in an orbital shaker at 37 °C for 7 h. After 3 h incubation, 100 µL of bacterial culture was drawn for every 1 h and plated on fresh LB agar with 100 µg ampicillin per mL. Plates were further incubated at 37 °C for 24 h. After 24 h, the transformed colonies with GFP exhibiting green fluorescence were observed using fluorescence microscope (Olympus). The efficiency of Arg–NiGs-mediated transformation of non-competent E. coli DH5α was compared with the conventional CaCl2-mediated transformation. Colonies were counted on the basis of cfu µg–1 DNA under UV light and the transformation efficiency (TE = Number of colonies observed /amount of DNA in µg) was determined.[Citation24]
2.6. Isolation of plasmid DNA from transformants
Plasmid DNA was isolated from transformed E. coli by alkaline lysis method.[Citation25] After checking the plates for transformed colonies, portion of individual colonies were scrapped using sterile pipette tip and inoculated in 3 mL of LB broth containing 6 µL of 5% ampicillin and was incubated in an orbital shaker at 37 °C for 2 h. 1 mL of the culture was then inoculated in 200 mL of LB broth containing 400 µL of 5% ampicillin and was incubated overnight in an orbital shaker at 37 °C. 1 mL of the overnight cultures was centrifuged at 5000 rpm for 10 min and the supernatant was drained. The cells were resuspended in 100 µL of solution A (23 mM Tris HCl, 50 mM glucose, 100 mg lysozyme in 10 mL water) and incubated in ice for 30 min. 200 µL of solution B (0.2 M NaOH, 1% sodium dodecyl sulfate (SDS) in 100 mL water) was added to the above contents, vortexed and incubated in ice for 5 min. Then, 150 µL of solution C (3 M Sodium acetate, pH 4.8 in 1 L water) was added, vortexed and incubated in ice for 60 min. The chromosomal DNA was separated by centrifuging at 5000 rpm for 10 min. To the supernatant 1.5 mL of 100% ethanol was added, incubated for 30 min and then centrifuged at 15000 rpm for 10 min to collect the plasmid DNA. The plasmid was isolated from transformed bacterial cells and compared with commercially available pUC18 vector in 1% agarose gel electrophoresis. The schematic representation of formation of pDNA–Arg–NiGs conjugate and its transformation are shown in
2.7. Analysis of expressed protein by SDS–PAGE
Expressed GFP protein in transformed E. coli cells were analysed following the protocol of Zhou et al.[Citation26] 1.5 mL of each overnight bacterial culture was centrifuged at 10,000 rpm for 5 min and the pellets were re-suspended in 250 µL of TE buffer. 10 µL of lysozyme was added to the resuspended solution and incubated at –70 °C for 20 min. The tubes were then thawed and centrifuged at 10,000 rpm for 10 min. The supernatant was size fractionated using a Sephadex G–75 column, swelled in 0.05 mol L–1 Tris–HCl buffer (pH 8.0) for 24 h at 37 °C. 200 mL of swelled gel was packed into XK 26/40 column by gravity slurry-sedimentation method.[Citation26] The column was connected to AKTA purifier chromatographic system and equilibrated with 0.05 mol L–1 Tris-HCl buffer (pH 8.0). Samples were injected to column at flow rate of 1.0 mL min–1 and GFP peak was evaluated by its absorbance at 488 nm and the fraction containing expressed GFP was collected. Crude proteins and purified GFP isolated from recombinant bacteria (transformed by Arg–NiGs and CaCl2) were used for sodium dodecyl sulfate polyacrylamide gel electrophoresis (SDS–PAGE) analysis.
Expressed GFP were analysed by SDS–PAGE electrophoresis.[Citation27] 5% acrylamide in 0.125 M Tris-HCl (pH 6.8) with 1% SDS was used as stacking gel and applied over separating gel containing 8.75% acrylamide in 0.375 M Tris-HCl (pH 8.8) with 1% SDS. Electrophoresis was performed at 150 V for 30 min at 37 °C using electrode buffer containing 10 mM Tris-glycine buffer (pH 8.7) with 1% SDS. The protein bands in the gel were visualised after staining with Coomassie brilliant blue stain (60 mg L–1 Coomassie brilliant blue R-250 in 10% acetic acid in water).
2.8. Bacterial cytotoxicity study
Biocompatibility of Arg–NiGs on E. coli DH5α was monitored by the growth curve.[Citation24] 105 cfu mL–1 of E. coli was grown in 50 mL LB broth with varying concentrations of nanoparticles (0, 25, 50 and 100 µg mL–1). The plates were incubated at 37 °C and shaken at 180 rpm. After incubation, the optical density (OD) at 600 nm was serially monitored at every 3 h interval till 24h.
2.9. Catalytic hydrolysis of ammonia borane by Arg–NiGs
2.9.1. Catalytic hydrolysis of ammonia borane
Catalytic activity of Arg–NiGs was determined as reported by Onder et al.[Citation28] 10 mL of aqueous dispersion of NiGs or Arg–NiGs catalyst was transferred into a two-necked round-bottom reaction flask. One neck of the flask was connected to a water-filled gas burette inverted in a water-filled vessel to monitor the volume of H2 generated by hydrolysis of AB, while the other neck was fitted with an inlet to introduce AB. 128 mg (4 mmol) of AB was added into the catalyst solution at 35 °C and stirred at 800 rpm. The volume of hydrogen gas generated was assessed by measuring the displacement of water level at 1 min interval. The reaction started after AB was added to the flask and the generation of hydrogen is described by the following equation [Citation29]:
The schematic representation of catalytic dehydrogenation of AB by Arg–NiGs is shown in . The catalytic activity was determined in terms of turnover frequency (TOF = mol H2/mol catalyst × time).[Citation30]
2.9.2. Recyclability test of Arg–NiGs for hydrolytic dehydrogenation of AB
Recyclability test for hydrolytic dehydrogenation of AB by Arg–NiGs was performed according to Qilu et al. [Citation31]. First cycle of catalytic dehydrogenation reaction was performed using 1.0 mmol L–1 catalyst and 500 mmol L–1 AB in 10 mL aqueous medium at 35 °C. 3.0 mol H2 (mol AB) –1 was generated after completion of first cycle of AB dehydrogenation. The catalyst was separated from reaction solution by centrifugation at 10,000 rpm for 5 min and then washed several times with water. Washed catalyst was re-dispersed in 10 mL water in the flask and another equivalent of 256 mg AB (8 mmol) was added to the reaction system. Then the released hydrogen gas was again observed by gas burette and the reactions were repeated for 10 times at 35 °C. After the recycle tests, the reaction solution was centrifuged at 10,000 rpm for 5 min to separate the catalyst and then washed several times with water and dried at 40 °C overnight in vacuum oven. The rate of AB hydrolysis by Arg–NiGs in recycle test was evaluated from volume of H2 generated per min.
2.9.3. Kinetic study of hydrolytic dehydrogenation of AB by Arg–NiGs
The rate of hydrolysis of AB by Arg–NiGs was performed by kinetic studies as reported by Onder et al. [Citation28]. The rate of hydrogen generated from Arg–NiGs catalysts was based on the concentration of NiGs nanoparticles, without considering the weight of arginine. The activation energy for NiGs and Arg–NiGs catalysed AB hydrolysis was determined from the slope of the Arrhenius plot at varying temperature (25, 35, 45 and 55 °C) at 4.5 mmol L–1 catalyst amount and 200 mmol L–1 AB concentration. A series of experiments were performed by varying AB concentration (200, 300, 400 and 500 mmol L–1) and catalyst amount (2.5, 4.5, 6.5 and 8.5 mmol L–1) at 35 °C with constant concentrations of catalyst (4.5 mmol L–1) and AB (200 mmol L–1), respectively, to evaluate the effect of AB concentration and catalyst amount on catalytic dehydrogenation of AB.
3. Results and discussion
3.1. Characterisation of L-arginine functionalised nickel nanoparticles
The UV–vis spectrum of Arg–NiGs showed the peak at 390 nm ((a)), while NiGs ((a)) recorded the peak at 395 nm as reported in our previous study.[Citation21] By conjugating L-arginine with NiGs, the absorption peak was shifted from 395 to 390 nm indicating blue shift. The broadness of the bandwidth indicated the smaller size of the nanoparticles. The blue shift in peak position along with broadening of peak in UV–vis spectra of Arg–NiGs shows the smaller particle size of Arg–NiGs as compared to NiGs. This size reducing effect of Arg–NiGs could be due to the prevention of nanoparticles agglomeration by L-arginine functionalisation. Sunatkari et al. [Citation32] have also reported the size reducing effect of increasing loading concentration of L-arginine (1–5 mM) on gold nanoparticles that shifted the resonance absorption peak from 520 to 513 nm. The results were also in good agreement with the findings of Li et al. [Citation33] who reported that the blue shift in the SPR peak position, accompanied by broadening of peak, is mainly due to decrease in size of gold nanoparticles.
Figure 2. Characterisation of Arg–NiGs and NiGs [Citation21]: UV–vis spectrum (a), FTIR spectrum (b) XRD pattern (c) and thermogravimetric (TG) curves (d).
![Figure 2. Characterisation of Arg–NiGs and NiGs [Citation21]: UV–vis spectrum (a), FTIR spectrum (b) XRD pattern (c) and thermogravimetric (TG) curves (d).](/cms/asset/f360bc34-06eb-4d6e-89a9-819fc2de2470/tjen_a_1204670_f0002_oc.jpg)
FTIR spectrum of both Arg–NiGs and NiGs [Citation21] in (b) shows the presence of peaks to at 3163, 2348, 2282 and 723 cm–1 which is characteristic to O–H stretching carboxylic acid, C–N or C–C triple bond, and C–N rock in alkanes, respectively. Furthermore, Arg–NiGs showed three additional intense peaks at 1713, 1633 and 1402 cm–1 characteristic to C–O stretch due to carboxylic group, N–H bending due to amine groups and O–H bending due to carboxylic group, respectively, indicating the binding of L-arginine with Arg–NiGs. The functional groups of L-arginine such as N–H, C–O and O–H could have strongly bound to nickel nanoparticles like capping and stabilising agent by preventing particle agglomeration in aqueous medium as reported previously by Mallikarjuna et al. [Citation34] for silver nanoparticles. In addition, Tetsuo et al. [Citation10] reported that the presence of NH2, CO and OH functional groups with decreased peak intensity in L-arginine stabilised nickel nanoparticles was due to binding of L-arginine to nickel nanoparticles.
(c) shows five distinct XRD peaks for Arg–NiGs and NiGs [Citation21] at 2θ values of 37.32°, 44.82°, 47.92°, 63.11° and 72.97°. The highest intensity of the peak was observed at 2θ value of 44.82° for both NiGs and Arg–NiGs. Their corresponding miller indices (111) and (200) confirmed that the samples were face-centred-cubic (fcc) nickel. The average particle size of NiGs and Arg–NiGs determined by Debye Scherrer equation was found to be 30 nm [Citation21] and 28 nm, respectively. Broadening of XRD peaks was observed in Arg–NiGs when compared to NiGs indicating reduction in size of nanoparticles by arginine coating. This could be due to the smaller size of L-arginine stabilised nanoparticles. Our results were in concurrence with Alireza et al. [Citation15] who have reported that the smaller size of the L-arginine stabilised iron–oxide nanoparticles had broad peaks in XRD pattern with 2θ values of 30º, 35.5º, 43º, 57º and 63º.
The TG curves of Arg–NiGs and NiGs ((d)) show few stabilising and destabilising interaction in accordance with the temperature region. Both NiGs and Arg–NiGs had minor weight loss between 100 to 300 °C and major weight loss between 350 to 410 °C. Arg–NiGs recorded a 20 °C delay in the weight loss when compared to NiGs. The second major weight loss of 25% and 20% occurred at 350 and 370 °C for NiGs and Arg—NiGs, respectively, indicating higher thermal stability of Arg–NiGs. Above 490°C, constant weights have been observed for both NiGs and Arg–NiGs. The minor weight loss between 100 and 300 °C for both Arg–NiGs and NiGs could be due to the decomposition of adsorbed amino acids and thermal dehydration of nanoparticles, respectively, while the second major weight loss above 350 °C could be due to the dehydration of organic residues and nanoparticles. The results are in agreement with Rahmani and Ghamamy [Citation35] who reported that the first degradation of nickel (II) chloride nanoparticles in TG curve from 60 °C to 100 °C is attributed to the removal of water from the surface, the second degradation from 100 °C to 190 °C is due to the dehydration and removal of organic residues and the third degradation from 510 °C to 780 °C resulted in additional mass loss of about 45%. Sheena et al. [Citation36] have also reported that the weight loss near 300 °C in TG curve of nickel oxide nanoparticles could be due to the decomposition of nickel carbonate.
TEM micrograph of Arg–NiGs in (a) shows that the shape of the nanoparticle was almost spherical with particle size ranging between 10 and 28 nm, while the particle size of the NiGs ((b)) ranged from 12 and 36 nm as reported in the previous study.[Citation21] This result indicated that the size of Arg–NiGs was significantly reduced when compared to NiGs. Moreover, NiGs showed a certain extent of particles agglomeration [Citation21] but Arg–NiGs did not show any particle agglomeration. Smaller size of Arg–NiGs (25 nm) than NiGs in TEM micrograph could be due to the prevention of agglomeration of nanoparticles by L-arginine coating on NiGs. Reduced particle diameter of L-arginine functionalised NiGs in TEM images indicated that L-arginine could be used to synthesise well-dispersed NiGs as previously reported in a similar study with nickel nanoparticles by Tetsuo et al. [Citation10].
Figure 3. Transmission electron micrograph of Arg–NiGs (a) and NiGs (b) [Citation21].
![Figure 3. Transmission electron micrograph of Arg–NiGs (a) and NiGs (b) [Citation21].](/cms/asset/0079262a-afcd-47ff-b35d-672969dbf938/tjen_a_1204670_f0003_oc.jpg)
3.2. Characterisation of pDNA–Arg–NiGs conjugate
UV–vis spectrum of pDNA–Arg–NiGs conjugate () shows the presence of two peaks at 260 and 390 nm. The peak at 360 nm is specific for Arg—NiGs, while the absorption peak at 260 nm is specific for pDNA molecule. The presence of two peaks characteristic of pDNA and Arg–NiGs shows the effective binding of Arg–NiGs with pDNA. The results were in agreement with Rabia et al. [Citation37] who reported that the UV–vis spectrum of pUC 18 plasmid conjugated with dinuclear cobalt (III) complex showed the peak at 256 nm due to binding of complexes to double stranded DNA through non-covalent interaction.
The formation of pDNA–Arg–NiGs conjugate was confirmed by electrophoresis of the samples on agarose gel (). GFP gene, pUC 18, GFP–NiGs and pDNA–NiGs exhibited electrophoretic mobility and formed bands at 736 bp (lane 2), 2686 bp (lane 3), 3422 bp (lane 5) and 3500 bp (lane 6), respectively. pUC 18 restricted with Bam H1 in lane 4 () showed bands at 2506 bp and 180 bp. pDNA–Arg–NiGs conjugate was retained around the sample well due to a small increase in the size of pDNA after conjugation with the Arg–NiGs. Electrophoretic mobility of pDNA–NiGs (lane 6 in ) indicated inefficient binding of NiGs with pDNA without arginine coating. Retaining of pDNA–Arg–NiGs conjugate around the sample well in the present study could be due to the change in the charge and size of the pDNA after conjugation with Arg–NiGs. These observations were in accordance with Xiao–Xiao et al. [Citation38] who reported that the amino-modified silica nanoparticles complexed with plasmid DNA did not move towards the positive electrode on electrophoresis due to charge and larger size of the complexes. The data showed that arginine coating on NiGs is necessary for efficient binding of pDNA and hence Arg–NiGs was used for further transformation experiments.
3.3. pDNA–Arg–NiGs conjugate-mediated bacterial transformation
Fluorescent images of E. coli cells transformed by pDNA–Arg–NiGs and CaCl2-mediated methods ((a) and (b)) show a profound increase in the number of transformants in pDNA–Arg–NiGs transformation as compared with CaCl2 transformation. The transformation efficiency of Arg–NiGs and CaCl2-mediated transformation was found to be 3.8 × 106 and 2.9 × 105 cfu µg–1 DNA, respectively. This is in concurrence with Saptarshi and Keka [Citation24] who reported higher transformation efficiency of surface-functionalised gold nanoparticles (4.2 × 107 µg–1 DNA) than CaCl2-mediated transformation (2.3 × 105 µg–1 DNA) in transformation of non-competent E. coli. The increase in transformation efficiency of Arg–NiGs in the study could be due to the enhancement of membrane permeability in the presence of arginine functionalised NiGs. Amir et al. [Citation3] have reported that the exposure of smaller concentrations of surface active molecules resulted in reversible changes in cell membrane and enables successful plasmid transfer. This result demonstrates the advantage of Arg–NiGs over conventional method and arginine functionalised nickel nanoparticles could have facilitated the permeation of DNA across the cell membrane while retaining the intact cell morphology.
Figure 6. Fluorescent micrographs of transformed Escherichia coli by Arg–NiGs method (a) and CaCl2 method (b).
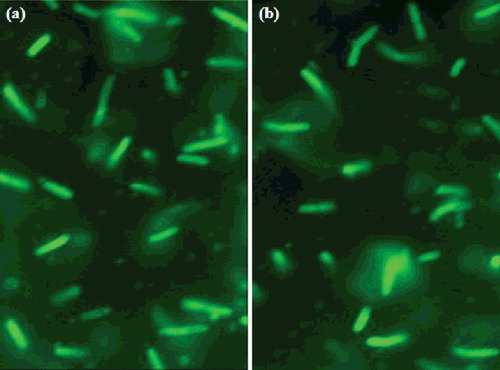
The electrophoretic analysis ((a)) of GFP–pDNA plasmid isolated from both pDNA–Arg–NiGs and CaCl2 transformed E. coli shows bands at 3422 bp (lane 3 and 4) and the commercially available pUC 18 vector showed band at 2686 bp. This result provides evidence for the interaction of pDNA with Arg–NiGs which does not affect pDNA function in E. coli. Therefore, electrophoretic analysis of GFP–pDNA from transformed E. coli indicated effective uptake of pDNA–Arg–NiGs by E. coli cells which depends on size and shape of the Arg–NiGs. Chithrani et al. [Citation39] have also reported that the size and shape of nanoparticles play an important role in uptake of gold nanoparticles into mammalian cells.
Figure 7. Electrophoretic analysis of pDNA isolated from transformed Escherichia coli (a) and GFP protein fractions extracted from transformed Escherichia coli (b).
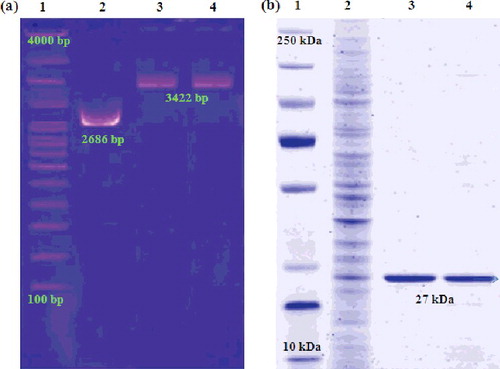
Analysis of crude and expressed GFP proteins isolated from E. coli transformed by Arg–NiGs and CaCl2-mediated methods ((b)) confirmed higher levels of GFP expression in Arg–NiGs-mediated transformation. The data showed that the interaction of pDNA with Arg–NiGs does not affect the integrity and function of GFP. In a similar study, Olga et al. [Citation20] have also reported that isolated plasmid vector pEGFP-C1 from pEGFP-C1–nickel nanoparticles complex retained their transfection ability in E. coli and resulted in the same level of GFP expression as the initial plasmid vector.
3.4. Bacterial cytotoxicity study
Growth curves of E. coli DH5α () with varied concentrations of Arg–NiGs (5–20 µg mL–1) did not show any cytotoxicity. Growth of untreated and Arg–NiGs treated E. coli DH5α showed a similar growth after 24 h incubation indicating biocompatibility of Arg–NiGs. Hence, the synthesised Arg–NiGs were found to be suitable for bacterial transformation experiments as they were not toxic to E. coli at the tested concentrations. The advantage of the current protocol is that no cytotoxicity of NiGs was observed against E. coli at tested NiGs concentrations. Similarly, Saptashi and Keka [Citation24] have also reported that the dry weight of untreated and glutathione functionalised gold nanoparticle (25–100 µg mL–1) treated E. coli cells remain unaltered (0.72 ± 0.02 g L–1).
3.5. Catalytic activity of Arg–NiGs for hydrolytic dehydrogenation of AB
(a) shows the plots of volume of hydrogen generated versus time in dehydrogenation of AB catalysed by NiGs and Arg–NiGs. The inset in (a) shows the TOF values of both the catalysts. It is evident from (a) that the generation of 198 mL of hydrogen by NiGs and Arg–NiGs was completed in 11 min and 8 min, respectively. The inset in (a) confirmed that Arg–NiGs possess higher TOF (9.8 mol H2 (mol Ni)–1min–1) than NiGs (7.09 H2 (mol Ni)–1min–1) at room temperature (35 °C). This could be due to the presence of relatively disperse Arg–NiGs particles without agglomeration. TOF value of Arg–NiGs catalyst (9.8 mol H2 (mol Ni)–1min–1) was found to be lower than most of the reported nickel-based nano-catalysts () in earlier studies,[Citation40–46] indicating the superior catalytic performance of Arg–NiGs. Increased hydrolysis of AB was observed in Arg–NiGs catalysed dehydrogenation when compared to NiGs which is due to prevention of agglomeration of NiGs by arginine coating that increased the specific surface area of nanoparticles for catalysis. This result was in concurrence with the previous study of Limin et al. [Citation47] who reported that the accumulation of nickel nanoparticles decreases the surface area and lower catalytic activity of nickel in nickel nanoparticles supported on carbon.
Figure 9. Hydrogen generated from AB hydrolysis catalysed by NiGs and Arg–NiGs (inset shows TOF values) (a), recycle tests for Arg–NiGs (inset shows reaction rates) (b), temperature effect on AB hydrolysis by NiGs (c) and Arg–NiGs (inset shows Ea and Arrhenius plot) (d).
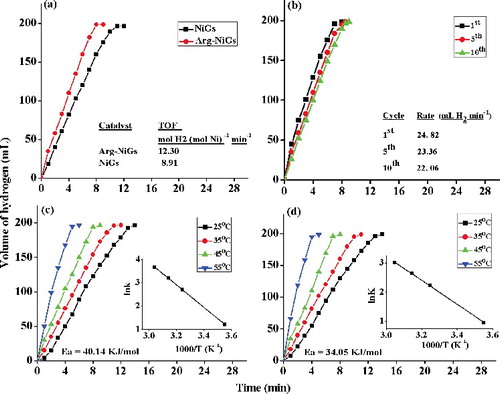
Table 1. Comparison of catalytic activity of Arg–NiGs and various nickel-based nano-catalyst used for hydrolytic dehydrogenation of ammonia borane.
3.6. Recyclability of catalyst
Reusability of catalyst is important for practical application [Citation32]. (b) shows the recyclability tests for Arg–NiGs in AB hydrolysis. The inset in (b) shows the rate of hydrogen generated at first, fifth and tenth recycles. Catalytic activity of Arg–NiGs in hydrolysis of AB after first, fifth and tenth recycles were found to be 24.8, 22.2 and 22.1 mL H2 min–1, respectively. Arg–NiGs retains about 89% of their initial catalytic activity even after tenth recycles of AB hydrolysis and this higher durability of Arg–NiGs in catalytic reactions could be due to the prevention of agglomeration by arginine coating that resulted in availability of active site during recycling process. These results were in agreement with Qilu et al. [Citation31] who reported that aggregated copper nanoparticles catalyst resulted in loss of activity site in recycling process for AB hydrolysis.
3.7. Kinetic study of hydrolytic dehydrogenation of ammonia borane by Arg–NiGs
(c) and (d) shows the plots of volume of hydrogen generated versus time for hydrolysis of AB by NiGs and Arg—NiGs, respectively, at different temperatures (25, 35, 45 and 55 °C) at constant concentration of AB and catalyst. The inset in (c) and (d) shows the Arrhenius plots of lnk versus the reciprocal absolute temperature (T–1) in kelvin. It is evident from (c) and (d) that the hydrogen generation rate by NiGs and Arg–NiGs increased significantly with increase in temperature (25–55 °C) in the presence of 200 AB and 4.5 mmol L–1 catalyst. The rate constant of the reaction was determined from the slope of the linear fitting region in each plot. The activation energy (Ea) determined from the slope of the plot was found to be 40.14 KJ mol–1 and 34.05 KJ mol–1 for NiGs and Arg—NiGs, respectively. Relatively lower activation energy values of Arg–NiGs (34.05 KJ mol–1) for hydrolysis of AB compared to NiGs indicated its better catalytic activity. As a result, the L-arginine effectively stabilised and improved the catalytic activity of nickel nanoparticles. L-arginine coating on NiGs enhanced the catalytic dehydrogenation of AB at different temperatures by reducing activation energy and increasing hydrogen generation. Similarly, Tetsuo et al. [Citation10] have also reported 2.2, 2.7 and 3.6 times increase in hydrogen generation rates on increasing L-arginine concentration from 12, 23 and 35 mg, respectively, in L-arginine stabilised nickel-catalysed reaction than bare nickel-catalysed reaction for hydrolysis of AB.
(a) shows the plots of volume of hydrogen generated versus time in hydrolytic dehydrogenation of different concentrations of AB (200–500 mmol L–1) by 4.5 mmol L–1 Arg–NiGs at 35 °C. (b) shows the plot of rate of hydrogen generated versus concentration of AB (both on logarithmic scales) and the inset in (b) shows the slope value of the plot obtained by linear fitting. The volume of hydrogen generated at 200, 300, 400 and 500 mmol L–1 AB concentration was found to be 124, 198, 230 and 278 mL in 8, 8, 10 and 12 min, respectively. The slope of the straight line ((b)) was determined to be 0.307 which is close to 0, indicating that the hydrolysis of AB was zero-order kinetics with respect to substrate concentration. The zero-order kinetics observed for catalytic hydrolysis of AB at varied AB concentration in the study is in concurrence with findings of Metin et al. [Citation48,Citation49] with monodisperse nickel nanoparticles and polymer-stabilised cobalt (0) nanoclusters, respectively. The results were also in close agreement with Limin et al. [Citation47] who stated that the AB hydrolysis by nickel nanoparticles supported on carbon was zero order with respect to AB concentration as the slope of the plot between hydrogen generation rates versus AB concentration was 0.32.
Figure 10. Hydrogen generated from hydrolysis at varied concentration of AB (a), plots of hydrogen generation rate versus AB concentration (b), hydrogen generated at varied Arg–NiGs dosage (c) and plots of hydrogen generation rate versus Arg–NiGs amount (d).
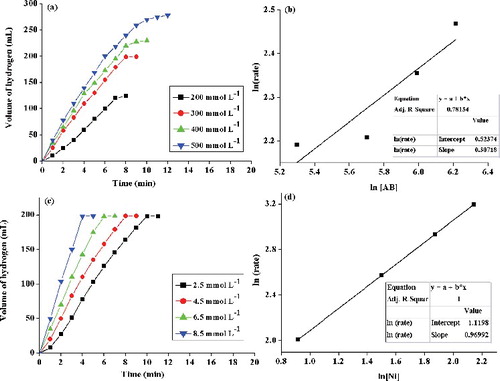
(c) shows the plot of volume of hydrogen generated versus time at different concentrations of catalyst (2.5, 4.5, 6.5 and 8.5 mmol L–1) on hydrolysis of 200 mmol L–1 AB at 35 °C. (d) shows the plot of hydrogen generated versus the concentration of nickel [Ni] (both on logarithmic scales). The inset in (d) shows the slope value of the plot by linear fitting. It is evident from (c) that 198 mL of hydrogen was generated in 10, 8, 7 and 5 min with increasing catalyst amount of 2.5, 4.5, 6.5 and 8.5 mmol L–1, respectively. (d) shows that the rate of hydrogen generation increased linearly with catalyst amount. The slope 0.96 was very close to one (unity), indicating that the hydrolysis of AB catalysed by Arg–NiGs was first-order kinetics with respect to catalyst concentration. The first-order dependence on catalyst [Ni] concentration for AB hydrolysis was also reported previously by Onder et al. [Citation28] and Limin et al. [Citation47] in catalytic dehydrogenation of AB by nickel nanoparticles with slope values of 0.98 and 0.94, respectively, in a plot of hydrogen generation rate versus concentration of the catalyst on logarithmic scale.
4. Conclusions
The NiGs were stabilised by L-arginine coating, which prevented the agglomeration and caused size reducing effect on nanoparticles with average particle size between 10 and 28 nm. The synthesised Arg–NiGs show higher thermal stability than NiGs in TG and DSC analysis. Effective binding of pDNA with Arg–NiGs was confirmed by UV–vis and electrophoretic analysis of pDNA–Arg–NiGs conjugate. Amino-acid stabilised nickel nanoparticles synergistically increased the efficiency of plasmid transformation into non-competent E. coli. Fluorescent micrograph indicated higher transformation efficiency (3.8 × 106 µg–1 DNA) and GFP expression by Arg–NiGs conjugate in non-competent E. coli as compared to conventional CaCl2 method (2.9 × 105 µg–1 DNA). The nanotoxicity and electrophoretic analysis shows biocompatibility, increased uptake of pDNA–Arg–NiGs and retaining of functional activity of pDNA in E. coli cells. This non-toxic and highly functional pDNA–Arg–NiGs conjugate could be exploited in the development of biosensors, transgenic animals and gene therapy. Synthesised Arg–NiGs were used as catalyst for hydrolytic dehydrogenation of AB. Arg–NiGs showed higher catalytic activity with 198 mL of hydrogen generation in 8 min with TOF of 12.30 mol H2 (mol Ni)–1 min–1 and activation energy of 34.05 KJ mol–1. Arg–NiGs retained 89% of their initial catalytic activity even after 10 recycles indicating its durability as catalyst. Kinetic studies show that hydrolytic dehydrogenation of AB by Arg–NiGs was zero order with respect to AB concentration and first order with respect to catalyst concentration. This economical and efficient catalyst is promising to replace noble metals for dehydrogenation of AB.
Disclosure statement
No potential conflict of interest was reported by the authors.
References
- Cai W, Gao T, Hong H, et al. Application of gold nanoparticles in cancer nanotechnology. J Nanotech Sci Appl. 2008;1:17–32.
- Avnesh K, Sudesh KY, Subash C. Biodegradable polymeric nanoparticles based drug delivery systems. Coll Surf B Biointer. 2010;75:1–18.
- Amir AS, Abolfazl B, Mostafa HM, et al. Fe3O4 nanoparticles engineered for plasmid DNA delivery to E. coli. J Nanopart Res. 2014;16:2521–2530.
- Sheng Y, Mancino V, Birren B. Transformation of E. coli with large DNA molecules by electroporation. Nucleic Acids Res. 1919;23:1990–1996.
- Solairaj D, Rameshthangam P, Srinivasan P. Adsorption of methylene blue, bromophenol blue and Coomassie brilliant blue by a-chitin nanoparticles. J Adv Res. 2016;7:113–124.
- Midoux P, Pichon C, Yaouanc JJ, et al. Chemical vectors for gene delivery: a current review on polymers, peptides and lipids containing histidine or imidazole as nucleic acids carriers. Br J Pharmacol. 2009;157:166–178.
- Ramirez-Meneses E, Betancourt I, Morales F, et al. Super paramagnetic nickel nanoparticles obtained by an organometallic approach. J Nanopart Res. 2011;13:365–374.
- Pozmogova G, Chuvilin A, Smirnov I, Zaitseva M, Tatarinova O, Govorun V. Preparation and properties of associates of nickel nanoparticles with ssDNA and proteins. Nanotechnol Russ. 2008;3:391–396.
- Yuwen Y, Fei Z, Hualan W, et al. Catalytic hydrolysis of ammonia borane by cobalt nickel nanoparticles supported on reduced graphene oxide for hydrogen generation. J Nanomater. 2014. doi:10.1155/2014/294350.
- Tetsuo U, Qiang X, Yoshiyuki K. Effect of L–arginine on the catalytic activity and stability of nickel nanoparticles for hydrolytic dehydrogenation of ammonia borane. J Power Sour. 2012;216:363–367.
- Zhang JS, Zhao Y, Akins DL, et al. Calorimetric and microscopic studies on the noncatalytic hydro thermolysis of ammonia borane. Indus Eng Chem Res. 2011;50:10407–10413.
- Wang J, Qin YL, Liu X, et al. In situ synthesis of magnetically recyclable graphene-supported Pd@Co core–shell nanoparticles as efficient catalysts for hydrolytic dehydrogenation of ammonia borane. J Mater Chem. 2012;22:12468–12470.
- Guo Y, Wang L, Yang L, et al. Optical and photocatalytic properties of arginine-stabilized cadmium sulfide quantum dots. Mater Lett. 2011;65:486–489.
- Durmus Z, Kavas H, Toprak MS, et al. L-lysine coated iron oxide nanoparticles: synthesis, structural and conductivity characterization. J Alloys Compd. 2009;484:371–376.
- Alireza E, Younes G, Sara RA, et al. Impact of amino-acid coating on the synthesis and characteristics of iron-oxide nanoparticles (IONs). Bull Korean Chem Soc. 2012;33:3957–3961.
- Shankar SS, Rai A, Ahmad A, et al. Rapid synthesis of Au, Ag, and bimetallic Au core-Ag shell nanoparticles using Neem (Azadirachta indica) leaf broth. J Colloid Interface Sci. 2004;275:496–502.
- Chitra J, Rameshthangam P, Solairaj D. Screening antimicrobial activity of nickel nanoparticles synthesized using Ocimum sanctum leaf extract. J Nanopart. 2016. doi: 10.1155/2016/4694367.
- Schabes-Retchkiman P, Canizal G, Herrera-Becerra R, et al. Biosynthesis and characterization of Ti/Ni bimetallic nanoparticles. Opt Mater. 2006;29:95–99.
- Cohen SN, Chang ACY, Hsu L. Nonchromosomal antibiotic resistance in bacteria: genetic transformation of E. coli by R-factor DNA. Proc: Natl Acad Sci. 1972;69:2110–2114.
- Olga N, Tatarinova, Igor PS, Irina VS, et al. DNA complexes with Ni nanoparticles: structural and functional properties. J Nanopart Res. 2012;14:1211–1219.
- Chitra J, Rameshthangam P, SolaiRaj D. Green synthesis of nickel nanoparticles using Ocimum sanctum and their application in dye and pollutant adsorption. Chin J Chem Eng. 2015;23:1307–1315.
- Sheng-Cong L, Han-Ning X, Yu-Ping L. Thermal analysis and degradation mechanism of polyacrylate/ZnO nanocomposites. Polym Degrad Stabil. 2005;87:103–110.
- Tang J, Liang S, Zhang J, et al. pGreen-S: a clone vector bearing absence of enhanced green fluorescent protein for screening recombinants. Anal Biochem. 2009;388:173–174.
- Saptarshi C, Keka S. Surface-functionalized gold nanoparticles mediate bacterial transformation: a nanotechnological approach. Biotechnol Lett. 2014;36:265–271.
- Bimboim HC, Doly J. A rapid alkaline extraction procedure for screening recombinant plasmid DNA. Nucleic Acid Res. 1979;7:1513–1523.
- Zhou X, Shi Q, Xing X, et al. Rapid purification of enhanced green fluorescent protein from Escherichia coli. Chin J Chem Eng. 2006;14:229–234.
- Laemmli UK. Cleavage of structural proteins during the assembly of the head of bacteriophage T4. Nature. 1970;227:680–685.
- Onder M, Saim O, Shouheng S. Monodisperse nickel nanoparticles supported on SiO2 as an effective catalyst for the hydrolysis of ammonia borane. Nano Res. 2010;3:676–684.
- Aranishi K, Jiang HL, Akita T, et al. One-step synthesis of magnetically recyclable Au/Co/Fe triple-layer core-shell nanoparticles as highly efficient catalysts for the hydrolytic dehydrogenation of ammonia borane. Nano Res. 2011;4:1233–1241.
- Feyyaz D, Salim C, Saim O, et al. Dihydrogen phosphate stabilized ruthenium (0) nanoparticles: efficient nanocatalyst for the hydrolysis of ammonia-borane at room temperature. Materials. 2015;8:4226–4238.
- Qilu Y, Zhang-Hui L, Zhujun Z, et al. One-pot synthesis of core-shell Cu@SiO2 nanospheres and their catalysis for hydrolytic dehydrogenation of ammonia borane and hydrazine borane. Sci Rep. 2014;4:7597–7605.
- Sunatkari ALhttp://article.sapub.org/10.5923.j.nn.20150502.02.html - Aff1, Talwatkar SS, Tamgadge YS, Muley GG. Synthesis, characterization and optical properties of L-arginine stabilized gold nanocolloids. J Nanosci Nanotechnol. 2015;5:30–35.
- Li Z, Li Y, Qian XF, et al. Simple method for selective immobilization of silver nanoparticles. Appl Surf Sci. 2005;250:109–116.
- Mallikarjuna K, Narasimha G, Dillip G, et al. Green synthesis of silver nanoparticles using Ocimum leaf extract and their characterization. Digest J Nanomater Biostruct. 2011;6:181–186.
- Rahmani Z, Ghamamy SH. Synthesis and characterization of nickel (II) chloride nanoparticles with the study of their thermal behavior. Int J Nano Dimens. 2015;6:401–407.
- Sheena PA, Priyanka KP, Aloysius NS, et al. Effect of calcination temperature on the structural and optical properties of nickel oxide nanoparticles. Nanosys Phys Chem Maths. 2014;5:441–449.
- Rabia E, Bozidar C, Miroslava V et al. Synthesis, characterization, cytotoxic activity and DNA binding properties of the novel dinuclear cobalt (III) complex with the condensation product of 2-acetylpyridine and malonic acid dihydrazide. J Inorg Biochem. 2011;105:1196–1203.
- Xiao-Xiao H, Kemin W, Weihong T, et al. Bioconjugated nanoparticles for DNA protection from cleavage. J Amer Chem Soc. 2003;125:7168–7169.
- Chithrani BV, Ghanzani AA, Chan WCW. Determining the size and shape dependence of gold nanoparticle uptake into mammalian cells. Nano Lett. 2006;6:662–668.
- Yang L, Cheng G, Su J, et al. In situ synthesis of graphene supported Ag@CoNi core–shell nanoparticles as highly efficient catalysts for hydrogen generation from hydrolysis of ammonia borane and methylamine borane. J Mater Chem A. 2013;1:10016–10023.
- Yang L, Luo W, Cheng G. Graphene-supported Ag-based core–shell nanoparticles for hydrogen generation in hydrolysis of ammonia borane and methylamine borane. ACS Appl Mater Interfaces. 2013;5:8231–8240.
- Feng W, Yang L, Cao N, et al. In situ facile synthesis of bimetallic CoNi catalyst supported on graphene for hydrolytic dehydrogenation of amine borane. Int J Hydro En. 2014;39:3371–3380.
- Xu Q, Chandra M. Catalytic activities of non-noble metals for hydrogen generation from aqueous ammonia-borane at room temperature. J Power Sour. 2006;163:364–370.
- Wen M, Sun BL, Zhou B, et al. Controllable assembly of Ag/C/Ni magnetic nanocables and its low activation energy dehydrogenation catalysis. J Mater Chem. 2012;22:11988–11993.
- Yan JM, Zhang XB, Han S, et al. Synthesis of longtime water/air-stable Ni nanoparticles and their high catalytic activity for hydrolysis of ammonia-borane for hydrogen generation. Inorg Chem. 2009;48:7389–7393.
- Umegaki T, Yan JM, Zhang XB, et al. Preparation and catalysis of poly (N-vinyl-2-pyrrolidone) (PVP) stabilized nickel catalyst for hydrolytic dehydrogenation of ammonia borane. Int J Hydro En. 2009;34:3816–3822.
- Limin Z, Tianran Z, Zhanliang T, et al. Ni nanoparticles supported on carbon as efficient catalyst for hydrolysis of ammonia borane. Nano Res. 2014;7:774–781.
- Metin O, Ozkar S. Hydrogen generation from the hydrolysis of ammonia–borane and sodium borohydride using water soluble polymer-stabilized cobalt (0) nanoclusters catalyst. Energy Fuels. 2009;23:3517–3526.
- Metin O, Mazumder V, Ozkar S, et al. Monodisperse nickel nanoparticles and their catalysis in hydrolytic dehydrogenation of ammonia borane. J Amer Chem Soc. 2010;132:1468–1469.