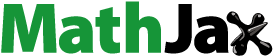
ABSTRACT
This paper describes a green one-pot synthesis of L-Serine (L-Ser) capped magnetite nanoparticles (Fe3O4 NPs) and its potential application for adsorption of RhB dye from aqueous solution. The surface property, structure, morphology and magnetic properties of as prepared L-Ser capped Fe3O4 NPs were characterised through UV-Visible spectroscopy, Fourier transform-infrared spectroscopy, X-Ray Diffraction (XRD), scanning electron microscope, transmission electron microscope (TEM) and vibrating sample magnetometer (VSM). The XRD results were indicated the formation of high crystalline spinel type Fe3O4 NPs. TEM images were shown the spherical shape of L-Ser capped Fe3O4 NPs with particle size of 5.9 nm. The VSM curve showed the superparamagnetic behaviour of L-Ser capped Fe3O4. A plausible interaction mechanism of L-Ser and Fe3O4 NPs was also investigated. L-Ser capped Fe3O4 NPs due to its large surface area and a strong magnetism was shown potential adsorption efficiency towards RhB dye from aqueous solution. The adsorption isotherm data fitted well with Langmuir isotherm model and the monolayer adsorption capacity (qe,exp) was found to be 6.82 mg/g at pH 7.4 and 300 K. The experimental kinetic data fitted very well with the pseudo-second-order model. The thermodynamic studies reveal that adsorption efficiency is critically dependent on temperature.
1. Introduction
In recent years, numerous industries have been troubling the water quality by release of various contaminants such as dyes, heavy metal ions and many other organic pollutants [Citation1–3]. Particularly, dyes because of their long time stability and enormous effluents have been released per year, which causes for adverse effect on human health such as pain, vomiting, skin irritation, severe headaches, acute diarrhoea, etc. [Citation3]. However, the conventional water treatment methods such as precipitation and flocculation are unsuccessful for the complete removal of dyes. Instead, adsorption is promising technology for an effective treatment of polluted water due to it is low-cost, simply designed, easy to handle, and provides sludge-free output [Citation4,Citation5]. Activated carbon has been long known for an effective colour removal adsorbent, but its expensive operation cost unable to address for a wide range of consumers [Citation6]. Hence developing of alternative adsorbents of less cost, ease of operation and simplistic design has been the focus of recent research.
In the last decades, many approaches have been studied for the development of low-cost and more effective adsorbents such as silica gel [Citation7], perlite [Citation8], lignite [Citation9], fly ash [Citation10], etc. Most of these adsorbents due to their highly porous in nature provide sufficient surface area for adsorption. Nanostructured materials due to its high surface area to volume ratio and can easily functionalise their surface by different molecules have been used for an efficient adsorption. Moreover, its unique structural, electrical, optical and magnetic properties can provide outstanding opportunities for the adsorption of dyes [Citation11,Citation12]. Notably, superparamagnetic nanoparticles as a result of easily manipulated by external magnetic field have been used for numerous applications including the environmental remediation [Citation13]. Superparamagnetic Fe3O4 NPs emerged as a potential candidate for advanced technological applications due to their biocompatibility, low toxicity and high magnetic saturations [Citation14]. However, Fe3O4 NPs below their critical particle size (∼20 nm) without surface capping could be easily aggregated during the course of chemical reaction [Citation15]. This phenomenon hampers to exploit the effectiveness of Fe3O4 NPs for their potential application.
In order to arrest aggregation of Fe3O4 NPs, surface capping or immobilisation using appropriate capping agents has been used such as polymers [Citation16] and hydrocarbons [Citation17] and others. However, these organic capping agents have a potential toxicity to the environment and public health. So, it is still a great challenge to develop biocompatible synthesis methods. More recently, amino acids have been showing a fascinating interest because of their biocompatibility and good capping capability towards Fe3O4 NPs [Citation18,Citation19]. Syntheses of Fe3O4 NPs with amino acids as a capping agent have often done in the absence of toxic organic solvents which increases the popularity of amino acids as capping materials [Citation19].
In this paper, we report a facile green synthesis of L-Ser capped Fe3O4 NPs via a co-precipitation of Fe3+ and Fe2+ salts in the presence of L-Ser as a capping agent. The as synthesised L-Ser capped Fe3O4 NPs were characterised through using UV-Visible, Fourier transform-infrared (FTIR), X-ray diffraction (XRD), scanning electron microscope/energy dispersive X-ray spectroscopy (SEM/EDS), transmission electron microscope (TEM) and vibrating sample magnetometer (VSM). The plausible interaction between L-Ser and Fe3O4 surface was investigated based on FTIR and UV-Visible spectroscopic techniques. Furthermore, the adsorption efficiency of L-Ser capped Fe3O4 NPs was investigated using RhB under various experimental parameters such as concentration of dye, contact time and temperature. The adsorption kinetics, thermodynamics and isotherms of RhB by using L-Ser capped Fe3O4 NPs were also investigated.
2. Experimental
2.1. Materials
Iron (III) chloride hexahydrate (FeCl3.6H2O) and Iron (II) sulphate heptahydrate (FeSO4.7H2O) used for synthesis of Fe3O4 NPs were purchased from Merck, India and used as without further purification. L-Ser and NH3 (25%) were received from HiMedia, India. All other reagents were analytical graded and used without further purification. Milli-Q water was used throughout the whole synthesis process.
2.2. Synthesis of L-Serine capped Fe3O4 NPs
Syntheses of Fe3O4 NPs were carried out through single-pot co-precipitation reaction in the presence of L-Ser as a capping agent. In a typical synthesis, 2:1 molar ratio of metal salts Fe+3 (0.54 g FeCl3.6H2O) and Fe+2 (0.278 g FeSO4.7H2O) was added in 250 mL round-bottomed flask, respectively, and the reaction was kept for one hour under constant string in atmospheric nitrogen at 80 °C. Then, 5 mL 2 M of L-Ser was added to the reaction mixture and then 5 mL ammonia solution (25%) was rapidly injected into reaction mixture. The reaction was further allowed to proceed for 2 hours at 80 °C under constant stirring. Then finally, the black precipitate was obtained and separated by external magnet, washed several times with Milli-Q water, and then dried in vacuum at room temperature. Similarly, L-Ser capped Fe3O4 NPs were also prepared by varying the concentration of L-Ser while all other reaction parameters were kept the same.
2.3. Characterisation
The UV-Visible absorption spectra were recorded using a Shimadzu 2450 – SHIMADZU spectrometer. FTIR spectra were recorded using a SHIMADZU-IR PRESTIGE-2 spectrometer. Powder samples were mixed thoroughly with KBr and pressed into thin pellets. The powder XRD patterns were obtained by PANalytical X'pert pro diffractometer at 0.02ο/sec scan rate with Cu-kα1 radiation (1.5406 Aο, 45 kV, 40 mA). Transmission electron microscopy images were obtained (TEM model FEI TECNAI G2 S-Twin) at an accelerating voltages of 120 and 200 kV. SEM images were acquired JEOL-JSM6610 LV equipped with an electron probe-micro analyser. Magnetic property measurement was carried out at room temperature (300 K) using VSM (Lakeshore 665, Coeur D Alene, ID, USA).
2.4. Batch mode adsorption studies
Batch adsorption efficiency of L-Ser capped Fe3O4 NPs experiments was carried out using RhB as a model dye at room temperature (300 K). In a typical experiment, 50 mg of L-Ser capped Fe3O4 NPs was added in 50 mL of known concentration of dye solution. The pH of solution was adjusted by NaOH (0.1 M) or HCl (0.1 M). The flasks were stirred for the specified time period and samples from each flask were withdrawn at the desired time of reaction until adsorption equilibrium was achieved. The L-Ser capped Fe3O4 NPs were collected by an external magnet. The residual dye concentration was determined by UV-Visible spectrophotometer by measuring the absorbance at a wavelength of maximum absorbance of RhB (554 nm). The amount of RhB adsorbed, q (mg/g) on L-Ser capped Fe3O4 NPs was calculated by(1)
(1) where Cο is the initial RhB concentration (mg/L), C is RhB concentration (mg/L) in solution after adsorption, V is the volume (L) of the aqueous solution and m is the mass (g) of L-Ser capped Fe3O4 NPs. Common adsorption isotherms and kinetic models were used to demonstrate the adsorption process.
3. Result and discussion
The UV-Visible spectra of uncapped and L-Ser capped Fe3O4 NPs are shown in . UV-Visible spectrum of both L-Ser capped and uncapped Fe3O4 NPs shows a broad absorption peaks which originates primarily from the absorption and scattering of light by Fe3O4 NPs [Citation20]. This characteristic peak was observed for both uncapped and L-Ser capped Fe3O4 NPs around 350 nm. The strong absorption peak at 200 nm for L-Ser capped Fe3O4 NPs is due to L-Ser (Electronic supplementary information [ESI], Figure S1); and it indicates effective capping of Fe3O4 NPs using L-Ser.
The phase structure and crystalline nature of uncapped and L-Ser capped Fe3O4 NPs were investigated using powder XRD. The XRD pattern of both uncapped and L-Ser capped Fe3O4 NPs is shown in , in which all peaks may be indexed to the inverse spinel phase of Fe3O4 (JCPDS card No. 19-0629). The XRD pattern shows peaks at 2θ = 30.77°, 35.82°, 43.4°, 57.52° and 63.02° corresponding to the planes of (220), (311), (400), (511) and (440). No peaks associated from other impurities were observed. The sharp and intense peaks indicate high crystallinity of the as synthesised Fe3O4 NPs. The average crystalline size of uncapped and L-Ser capped Fe3O4 NPs was estimated using Scherer formula [Citation21] and found to be 32.6 and 10.5 nm for uncapped and L-Ser capped Fe3O4 NPs, respectively. This attributed to the L-Ser act as a protective material for the growing of Fe3O4 NPs during the synthesis process and which is in accordance to the reported literatures [Citation22].
The structural information of as prepared Fe3O4 NPs was investigated through FTIR spectra. The FTIR spectra of uncapped and capped Fe3O4 NPs are presented in . Uncapped Fe3O4 NPs have two prominent peaks at 1629 and 3424 cm−1 which ascribed to the O-H bending and stretching vibrations respectively and indicated that the water molecule is coordinated with unsaturated Fe atom in uncapped Fe3O4 surface [Citation22]. FTIR spectrum of L-Ser is shown in Figure S2, ESI which is in a good agreement with previously reported data [Citation23] and it has two peaks at 1602 and 1407 cm−1 which were due to asymmetric and symmetric stretching frequencies of COO−, respectively. FTIR of L-Ser capped Fe3O4 NPs ((b)) shows that the peaks at 1598 and 1345 cm−1 were assigned to the va(COO-) and vs(COO−) stretching vibrations of carboxyl group of L-Ser, respectively [Citation24]. The energy differences between these asymmetric and symmetric stretching frequencies of carboxyl groups in L-Ser capped Fe3O4 give hint about the nature of interaction of carboxyl group with the metal surface. Hence, the energy differences between the asymmetric and symmetric stretching frequencies (Δνa−s = 1598 − 1345 cm−1 = 223 cm−1) were observed in the range of the bidentate complexing of carboxyl group with unsaturated Fe cation [Citation24]. The strong peaks at 585 and 412 cm−1 were associated to the intrinsic Fe–O vibrations of L-Ser capped Fe3O4 NPs [Citation25]. The FTIR confirms that the L-Ser was coated on the surface of Fe3O4 NPs.
Morphology of L-Ser capped Fe3O4 NPs was investigated using SEM and TEM. SEM images clearly showed that the L-Ser capped Fe3O4 NPs have nearly spherical shape (). EDS spectra ((d)) confirm that the presence of Fe and O atoms with 82% and 16% weight value, respectively, which were near to the theoretical percent weight values of pure magnetite. TEM images of as prepared L-Ser capped Fe3O4 NPs dried powder dispersed in dimethyl sulfoxide are depicted in and clearly have the spherical morphology with average particle size of 5.9 nm and standard deviation 1.58 nm of L-Ser capped Fe3O4 NPs. A selected area electron diffraction pattern obtained from the particles is given in (c) and in good agreement with the characteristic electron diffraction pattern of Fe3O4 NPs of spinel structure [Citation26]. (d) shows the particle size distribution of L-Ser capped Fe3O4 NPs.
Figure 4. Representative SEM images (a–c) of L-Ser capped Fe3O4 and (d) EDS spectrum and the relative percent weight analysis (inset) of L-Ser capped Fe3O4 NPs.
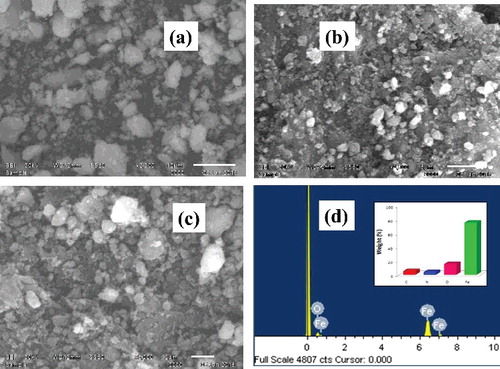
Figure 5. (a,b) Representative TEM images of L-Ser capped Fe3O4 NPs, (c) selected area electron diffraction patterns and (d) size distribution histogram.
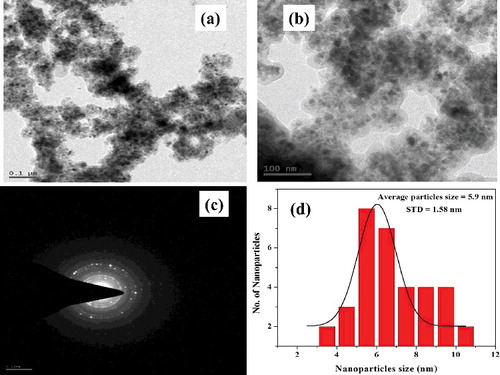
The room temperature magnetisation curves of L-Ser capped Fe3O4 NPs are depicted in . The magnetisation curve shows the absence of hysteresis loop and zero coercivity at room temperature indicating that the superparamagnetic behaviour of L-Ser capped Fe3O4 NPs. The saturation magnetisation (Ms) was found to be 85 emu/g, and smaller than bulk Fe3O4 of 92 emu/g [Citation25]. The decrease in the Ms value of L-Ser capped Fe3O4 NPs could be ascribed to size and surface effects induced by the nanoscale particle size of L-Ser capped Fe3O4 NPs. Moreover, the nonmagnetic capping agent (L-Ser) adsorbed on the surface of Fe3O4 NPs and thus resulted in decrease of Ms value.
3.1. Plausible interaction mechanism of L-Ser on to Fe3O4 surface
In general, amino acids are amphiprotic in nature and their charge critically depends on pH of a solution and thus, very small variations in pH could change in the charge of amino acid. At isoelectric point (pI) (pH = 5.68), L-Ser predominantly exists as zwitterion form (as shown in ). When the pH of solution is less than pI, then L-Ser exists as cationic form (-NH3+, -COOH), while it is present in anionic form (-NH2, -COO−) at higher pH value. Therefore, at high pH value, L-Ser behaves as strong nucleophile and able to form a bond with an electron deficient species. Nanoparticles due to their high surface to volume ratio, coordinative unsaturated surface species undergo either defects or chemical interaction to gain their stability. Fe3O4 NPs are inverse spinel structure where Fe3+ occupied both Td and half of Oh sites and Fe2+ half of Oh of the unit cell. However, Fe3+/Fe2+ found on surface Fe3O4 NPs not fully bound to adjacent oxygen atoms and undergo interaction with L-Ser to fulfil their stability. L-Ser at pH > 9.15, predominantly exists in its anionic form (COO− and NH2) which is strong nucleophile and could be form a bond with Fe3+/Fe2+ ().
Figure 7. Plausible interaction mechanisms of L-Ser to Fe3O4 NPs through (a) carboxyl bi-dentate, (b) carboxyl mono-dentate and (c) amino groups.
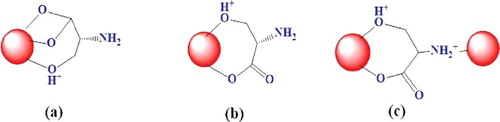
Carboxylate ions have two possible interaction modalities to Fe3+/Fe2+ either uni- or bi-dentate as shown in (a,b); however, FTIR analysis was ascribed the bi-dentate [Citation24]. The amino group of L-Ser ((c)) through nitrogen atom could coordinate to Fe3+ of Fe3O4 NP. The alcoholic terminal also has plausibly made a bond with unsaturated Fe atom of Fe3O4 NPs.
3.2. Adsorption studies
In general, adsorption efficiency is highly dependent on surface characteristics, size distribution and extent of functional groups on adsorbent [Citation12]. Nanosize distribution of L-Ser capped Fe3O4 NPs along with the presence of active functional groups (COO− and NH2) on its surface develops better adsorption for RhB dye. In the present study, it was obtained that L-Ser capped Fe3O4 NPs have shown better adsorption efficiency than uncapped Fe3O4 ((a)). At adsorption equilibrium (180 min) the percent removal (%) efficiency of L-Ser capped Fe3O4 NPs ((b)) at 300 K, pH = 7.4, were found to be 85.5%, 65.6% and 45.4% for concentration of 5, 10 and 15 ppm of RhB, respectively.
3.3. Adsorption kinetics
Adsorption kinetics of RhB by L-Ser capped Fe3O4 NPs was investigated by Lagergren pseudo-first-order (EquationEquation (2(2)
(2) )) and pseudo-second-order equation (EquationEquation (3
(3)
(3) )) models. The first-order kinetic model of Lagergren is applied for fast adsorption of any solute from solution and the process mainly controlled by diffusion [Citation27]:
(2)
(2) where K1 is the rate constants of the pseudo-first-order and qe denotes the amount of dye adsorption at equilibrium.
Instead, the pseudo-second-order model proposes that chemiadsorption is the rate-limiting step and that adsorption occurs on localised sites where no interactions between adsorbates take place [Citation28].(3)
(3) where K2 is pseudo-second-order kinetic models.
The adsorption kinetics analysis of RhB on to L-Ser capped Fe3O4 Nps was investigated by two models as shown in . A correlation coefficient value (R2) as shown in obtained from pseudo-second-order kinetic model much closer to unity than that of pseudo-first-order model. Hence, the adsorption kinetics of RhB onto L-Ser capped Fe3O4 NPs was found to follow pseudo-second-order kinetics, and the rate-determining step is predominantly chemiadsorption [Citation29]. The maximum adsorption efficiency of L-Ser capped Fe3O4 NPs towards RhB was calculated from pseudo-second-order kinetics (qcal) found to be good agreement to experimental (qexp) (). The pseudo-second-order kinetics further predicts that adsorption proceed through chemiadsorption process, in which the adsorption process predominantly affected by electrostatic interaction between the cationic dyes and the L-Ser capped Fe3O4 NPs.
Figure 9. (a) Pseudo-first-order and (b) pseudo-second-order plots for adsorption of RhB on L-Ser capped Fe3O4 NPs at varying initial concentration of RhB (error bar represents 5% error).
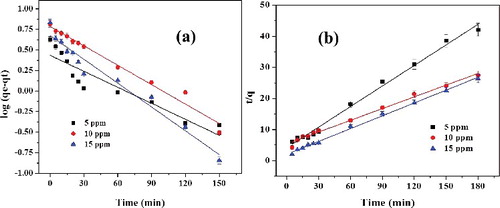
Table 1. The pseudo-first-order and pseudo-second-order kinetics parameters of RhB adsorption on L-Ser capped Fe3O4 NPs.
3.4. Adsorption isotherm
In order to develop a suitable adsorption system for RhB on to L-Ser capped Fe3O4 NPs, Langmuir, Freundlich and Temkin adsorption isotherm models were investigated and their corresponding curves are depicted in . The Langmuir [Citation30] equation can be expressed in a linearised form:(4)
(4) where qe (mg/g) is the equilibrium amount of dye adsorption, Qo (mg/g) refers to the maximum adsorption capacity of L-Ser capped Fe3O4 NPs and bL (L/mg) is the Langmuir equilibrium constant related to the enthalpy of the process.
Figure 10. (a) Effect of RhB concentration on amount of dye adsorbed on L-Ser capped Fe3O4 NPs (qt), (b) Langmuir isotherm, (c) Freundlich isotherm (d) Tempkin isotherm models.
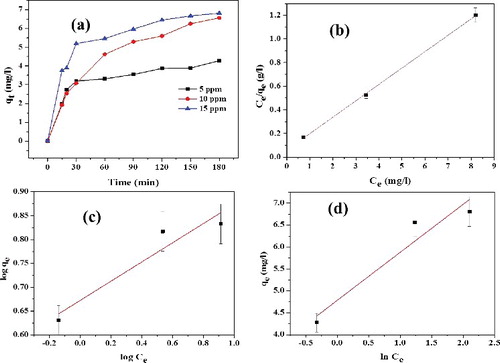
Hall et al. [Citation31] correlate the characteristics of Langmuir isotherm constant (bL) with a dimensionless factor RL (EquationEquation (5(5)
(5) )) to describe the enthalpy of adsorption process:
(5)
(5)
The value of separation factor RL indicates the nature of the adsorption process as: RL > 1 (unfavourable), RL = 1 (linear), 0 < RL < 1 (favourable) and RL = 0 (irreversible). In this study, RL was obtained between 0.03 and 0.08 () and hence the values of RL computed are <1, indicating that the adsorption process is favourable.
Table 2. Langmuir, Freundlich and Tempkin adsorption isotherms’ parameters for the adsorption of RhB onto L-Ser capped Fe3O4 NPs.
The Freundlich [Citation32] isotherm can be applicable for modelling the adsorption of dye on heterogeneous surfaces and the linearised form of isotherm is expressed as(6)
(6) where KF (mg/g) is Freundlich constant representing the adsorption capacity at unit equilibrium concentration that is defined as the adsorption or distribution coefficient and 1/n is related to the adsorption intensity. The value of Freundlich constant ‘n’ is greater than 1.0, as shown in (d), indicating that RhB favourably adsorbed on L-Ser capped Fe3O4 NPs.
Tempkin [Citation33] isotherm is based on the assumption that the heat of adsorption would decrease linearly with the increase of coverage of adsorbent is expressed as(7)
(7) where aT and bT are Tempkin constants.
The adsorption isotherms described by these three models for RhB adsorption by L-Ser capped Fe3O4 NPs are shown in (b–d), and the parameters and R2 values calculated from the three models are shown in . The regression coefficients (R2) obtained from their values of Langmuir model (R2 = 0.99), Freundlich model (R2 = 0.85) and Tempkin model (R2 = 0.87) were obtained, indicating that the data is best fit to the Langmuir isotherm, and thus the RhB adsorption on L-Ser capped Fe3O4 NPs is monolayer adsorption. The maximum monolayer adsorption capacity (Qo) was found to be 7.19 mg/g and it has competitive adsorption efficiency compared with common adsorbent (ESI ).
3.5. Adsorption thermodynamics
The effect of temperature on the adsorption efficiency of L-Ser capped Fe3O4 towards RhB at initial concentration of 10 ppm and pH = 7.4 is presented in . The experiments were carried out at 290, 300, 310 and 320 K and as shown in (a), the adsorption capacity increases from 6.8 to 8.3 mg/g with the increase of temperature from 290 to 320 K. It is apparent that the adsorption of RhB on the L-Ser capped Fe3O4 is an endothermic process, implying a chemical adsorption process [Citation34]. The energy changes associated with the adsorption process were also investigated by evaluating thermodynamic parameters: standard Gibbs energy change (ΔG°), enthalpy change (ΔH°) and entropy change (ΔS°). The thermodynamic parameters for the adsorption process were obtained using EquationEquations (8(8)
(8) ) and Equation (9
(9)
(9) ):
(8)
(8) where bL is Langmuir isotherm constant obtained from EquationEquation (4
(4)
(4) ), T is the temperature and R is the gas constant, respectively. The ΔH° and ΔS° values were determined experimentally from the slope and intercept of van't Hoff plots, i.e. ln bL versus 1/T ((b)) and the calculated values are presented in . The standard Gibbs energy change (ΔG°) can be calculated using EquationEquation (9
(9)
(9) ):
(9)
(9)
Figure 11. Effect of temperature on (a) adsorption efficiency and (b) van't Hoff plot (5% error bar) for adsorption of RhB (10 ppm) onto of L-Ser capped Fe3O4 NPs.
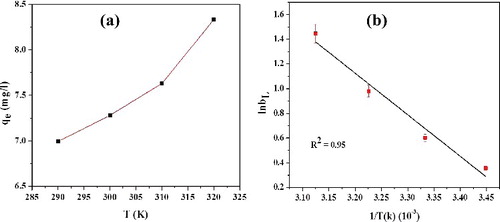
The negative value () of ΔG° at various temperatures remarks the spontaneous nature of adsorption of RhB onto L-Ser capped Fe3O4 NPs, and amount of dye adsorbed (qe) at equilibrium increases with increasing temperature. Positive value of ΔH° clearly shows that the adsorption process of RhB onto L-Ser capped Fe3O4 is endothermic. The positive ΔS° confirms the randomness of the system and it results an increase in the rate of diffusion of RhB molecules across the surface of the L-Ser capped Fe3O4 NPs rises, which leads to an increase in the adsorption efficiency [Citation35].
Table 3. Thermodynamic parameters for the adsorption of RhB onto L-Ser capped Fe3O4 NPs.
4. Conclusion
In summary, a facile green synthesis of superparamagnetic L-Ser functionalised Fe3O4 NPs was successfully developed by using a biocompatible capping agent, L-Ser. UV-Visible and FTIR results reveal the effective surface capping of Fe3O4 NPs by using L-Ser. Pure phase and good crystalline nature of L-Ser capped Fe3O4 NPs were confirmed by XRD. SEM and TEM techniques indicate spherical morphology of L-Ser capped Fe3O4 NPs. VSM showed the superparamagnetic nature of L-Ser capped Fe3O4 NPs. The adsorption behaviour of L-Ser capped Fe3O4 NPs towards RhB was followed by a pseudo-second-order kinetic model, suggesting chemiadsorption. The adsorption mechanism best fit to Langmuir model which indicates the formation of a monolayer covering of the adsorbate at the outer surface of the adsorbent. The maximum adsorption capacity (qe,exp) was found to be 6.82 mg/g at pH 7.4 and temperature 300 K. Studies on thermodynamic parameters reveal that the adsorption process is endothermic and spontaneous. Furthermore, the adsorption process was shown a positive response with an increasing temperature from 290 to 320 K.
tjen_a_1279354_sm3754.pdf
Download PDF (184 KB)Acknowledgments
The authors thank the Government of Ethiopia and Department of Inorganic & Analytical Chemistry, Andhra University to support this research work. The authors also thank Advanced Analytical Laboratory, Andhra University for their support in carrying out this research work regarding SEM-EDS, FT-IR and XRD analysis.
Disclosure statement
No potential conflict of interest was reported by the authors.
References
- Gao HC, Sun YM, Zhou JJ, et al. Mussel-inspired synthesis of polydopamine-functionalized graphene hydrogel as reusable adsorbents for water purification. ACS Appl Mater Interf. 2013;5:425–432.
- Mahony TO, Guibal E, Tobin JM. Reactive dye biosorption by Rhizopus arrhizus biomass. Enzyme Microb Technol. 2002;31:456–463.
- Gupta VK, Mohan D, Sharma S. Removal of basic dyes (Rhodamine B and Methylene Blue) from aqueous solutions using bagasse fly ash. Sep Sci Technol. 2000;5:2097–2113.
- Baek MH, Ijagbemi CO, O SJ, et al. Removal of Malachite Green from aqueous solution using degreased coffee bean. J Hazard Mater. 2010;176:820−828.
- Ozcan AS, Ozcan A. Adsorption of acid dyes from aqueous solutions onto acid activated bentonite. J Colloid Interf Sci. 2004;276:39–46.
- Lakouraj MM, Norouzian RS, Balo S. Preparation and cationic dye adsorption of novel Fe3O4 supermagnetic/thiacalix[4]arene tetrasulfonate self-doped/ polyaniline nanocomposite: kinetics, isotherms, and thermodynamic study. J Chem Eng Data. 2015;60:2262–2272.
- Mohamed MM. Adsorption properties of ionic surfactants on molybdenum-modified silica gels. Colloid Surf A. 1996;108:39–48.
- Alkan M, Dogan M. Adsorption of copper (II) onto perlite. J Colloid Interf Sci. 2001;243:280–291.
- Allen SJ, Mckay G, Khader KZ. Equilibrium adsorption isotherms for basic dyes onto lignite. J Chem Tech Biotechnol. 1989;45:291–302.
- Dogan M, Alkan M. Removal of methyl violet from aqueous solution by perlite. J Colloid Interf Sci. 2003;267:32–41.
- Keyhanian F, Shariati S, Faraji M, et al. Magnetite nanoparticles with surface modification for removal of methyl violet from aqueous solutions. Arab J Chem. 2011;9:S348–S354
- Tana KB, Vakilib M, Horria BA, et al. Adsorption of dyes by nanomaterials: recent developments and adsorption mechanisms. Sep Purif Technol. 2015;150:229–242.
- Mak SY, Chen DH. Fast adsorption of methylene blue on polyacrylic acid-bound iron oxide magnetic nanoparticles. Dyes Pigm. 2004;61:93–98.
- Qin H, Wang CM, Dong QQ, et al. Preparation and characterization of magnetic Fe3O4 – chitosan nanoparticles loaded with isoniazid. J Magn Mater. 2015;381:120–126.
- Zhao G, Feng J, Zhang Q, et al. Synthesis and characterization of Prussian blue modified magnetite nanoparticles and its application to the electrocatalytic reduction of H2O2. Chem Mater. 2005;17:3154–3159.
- Prabha G, Raj V. Preparation and characterization of polymer nanocomposites coated magnetic nanoparticles for drug delivery applications. J Magn Magn Mater. 2016;408:26–34.
- Rezaeifard A, Jafarpour M, Naeimi A, et al. Aqueous heterogeneous oxygenation of hydrocarbons and sulfides catalyzed by recoverable magnetite nanoparticles coated with copper(II) phthalocyanine. Green Chem. 2012;14:3386–3394.
- Schwaminger SP, García PF, Merck GK, et al. Nature of interactions of amino acids with bare magnetite nanoparticles. J Phys Chem C. 2015;119:23032–23041.
- Tie SL, Lin YQ, Lee HC, et al. Amino acid-coated nano-sized magnetite particles prepared by two-step transformation. Colloids Surf A. 2006;273:75–83.
- Ahmad S, Riaz U, Kaushikand A, et al. Soft template synthesis of super paramagnetic Fe3O4 nanoparticles a novel technique. J Inorg Organomet Polym. 2009;19:355–360.
- Patterson A. The Scherrer formula for X-ray particle size determination. Phys Rev. 1939;56:978–982.
- Ebrahiminezhad A, Ghasemi Y, Amini SR, et al. Impact of amino-acid coating on the synthesis and characteristics of iron-oxide nanoparticles (IONs). Bull. Korean Chem. Soc. 2012;33:3957–3962.
- Barth A. The infrared absorption of amino acid side chains. Prog Biophys Mol Biol. 2000;74:141–173.
- Wang Z, Zhu H, Wang X, et al. Yang one-pot green synthesis of biocompatible arginine-stabilized magnetic nanoparticles. Nanotechnology. 2009;20:465606.
- Cornell RM, Schwertmann U. The iron oxides: structures, properties, reactions, occurrences and uses. Weinheim: Wiley-VCH; 2003.
- Basavaiah K, Kumar PY, PrasadaRao AV. A facile one-pot synthesis of polyaniline/magnetite nanocomposites by micelles-assisted method. Appl Nanosci. 2013;3:409–415.
- Lagergren S. Kungliga Suenska Vetenskapsakademiens. Zur theorie der sogenannten adsorption gelˆster stoffe. Vol. 24. Handlingar; 1898; p. 1–39.
- Ho YS. Adsorption of heavy metals from waste streams by peat [PhD dissertation]. Birmingham: Univ. Birmingham; 1995.
- Ho YS, McKay G. Pseudo-second order model for sorption processes. Proc Biochem. 1999;451: 451–465.
- Langmuir I. The constitution and fundamental properties of solids and liquids. Part I. Solids. J Am Chem Soc. 1916;38:2221–2295.
- Hall KR, Eagleton LC, Axerivos A, et al. Pore and solid diffusion kinetics in fixed bed adsorption under constant pattern conditions. Ind Eng Chem Fundamen. 1966;5:212–223.
- Freundlich HMF. Over the adsorption in solution. J Phys Chem. 1906;57:385–471.
- Tempkin MI, Pyzhev V. Kinetics of ammonia synthesis on promoted iron catalyst. Acta Phys Chem (USSR). 1940;12:327–356.
- Mohammadi A, Lakouraj MM, Barikani M. Preparation and characterization of p-tert-butyl thiacalix[4]arene imbedded flexible polyurethane foam: an efficient novel cationic dye adsorbent. React Funct Polym. 2014;83:14–23.
- Ai L, Zhang C, Liao F, et al. Removal of methylene blue from aqueous solution with magnetite loaded multi-wall carbon nanotube: kinetic, isotherm and mechanism analysis. J Hazard Mater. 2011;198:282–290.