ABSTRACT
The Co(II) complex of [Co(bpy)2(NO3)]Cl·3H2O, bpy = 2,2'-bipyridine, has been synthesised by the solution crystallisation method. The boron nitride nanosheets/silver nanoparticles/[Co(bpy)2(NO3)]Cl·3H2O nanoaggregate was prepared in order to better analyse the adsorption orientation of the cobalt complex on the surface of silver nanoparticles. The result of the surface-enhanced Raman scattering measurement indicates that the molecular plane of the cobalt complex presents a tilted orientation with respect to the surface of silver nanoparticles. The luminescence property of [Co(bpy)2(NO3)]+ two-dimensional arrangements within the layers of zirconium phosphate (ZrP) was also investigated. Steady-state luminescence spectra of the [Co(bpy)2(NO3)]+-exchanged ZrP materials show an increase in the luminescence intensity.
1. Introduction
Two-dimensional nanomaterials have attracted tremendous attention due to their unique properties and potential applications [Citation1–4]. Boron nitride nanomaterials are reported to be promising nanoscale building blocks of new functional composite materials. As recently demonstrated, BN nanosheet has been utilised as burgeoning support to disperse and stabilise metal nanoparticles [Citation5–10]. It is expected that the metal nanoparticles anchored on BN nanosheet potentially exhibit novel catalytic and optoelectronic properties.
The immobilisation of luminescent metal complexes in rigid matrixes has become an intense area of investigation because of its application in solar energy conversion, LED displays and oxygen sensing, among others [Citation11–15]. Zirconium phosphate (Zr(HPO4)2∙H2O, ZrP) has been used to immobilise tris(2,2'-bipyridyl)ruthenium(II) (Ru-(bpy)32+) resulting in interesting photophysical characteristics [Citation16].
Rational design and construction of cobalt(II) complexes have been greatly developed recently, not only because of their structural diversities, but also owing to their potential applications in gas storage, catalysis, fluorescence and magnetism materials [Citation17–20]. In this paper, we present the surface-enhanced Raman scattering (SERS) of [Co(bpy)2(NO3)]Cl·3H2O on BN/Ag-NPs nanoaggregates and the photophysical characterisation of the interaction among [Co(bpy)2(NO3)]+ ions intercalated in the ZrP lamellar lattice. We chose Ag-NPs as the metal nanoparticles to decorate the BN nanosheets. It has been demonstrated that Ag-NPs dispersed onto BN nanosheets show excellent SERS activity, compared with the corresponding Ag-NPs [Citation21]. On the basis of structural consideration, we present a proposed adsorption orientation of the cobalt complex on the surface of Ag-NPs and structure orientation for the arrangement of the [Co(bpy)2(NO3)]+ ions within the layers of ZrP. Our work may provide a general way to construct the metal complexes-two-dimensional nanocomposites for various applications.
2. Experimental
2.1. Synthesis of [Co(bpy)2(NO3)]Cl·3H2O
Three solutions were prepared: (a) 312 mg of CoCl2·6H2O was dissolved in 10 mL deionised water (b) 238 mg of 2,2'-bipyridine was dissolved in 10 mL ethanol absolute and (c) 89 mg of NaN(CN)2 was dissolved in 5 mL deionised water. Solution b was added to solution a drop by drop with constant stirring. After all of solution b had been added, solution c was then added to the mixture drop by drop with constant stirring. The reaction mixture was stirred for 3 hours, and let it sit. A fine croci cube grains precipitated out. After about 24 hours’ standing, however, the fine croci cube grains disappeared, and a fine orange short needle grains precipitated out. The orange grains were washed in centrifugal machine rapidly with 10 mL cold water and dried in air.
2.2. Preparation of BN/Ag-NPs
AgNO3 (60 mg) was dissolved in 10 mL distilled water and then added the solution slowly to the BN nanosheet suspension (0.2 mg/mL, 100 mL) with stirring. In order to fully blend, the solution was kept stirring at room temperature for another 24 hours. Then, a mixture of 60 μL N2H4·H2O (wt 50%) and 10 mL distilled water were added to the suspension with vigorous stirring. The mixed solutions were centrifuged for three times with distilled water and was collected the solid product. The solid product was kept in drying oven at constant temperature for further use.
2.3. Preparation of BN/Ag-NPs/[Co(bpy)2(NO3)]Cl·3H2O
Thirty milligrams solid product of BN/Ag-NPs were taken and added to 2 mL DI water in a beaker. The BN/Ag-NPs suspension was dispersed with ultrasonic treatment for 15 min to fully mixing. Then, 10 mL [Co(bpy)2(NO3)]Cl-3H2O aqueous solution (5 mM) was added to the BN/Ag-NPs dispersion and the mixture was stirred for 2 hours. BN/Ag-NPs/[Co(bpy)2(NO3)]Cl·3H2O aggregation was obtained by centrifuging and drying.
2.4. Intercalation experiment
The synthesis of 10.4 Å hydrated phase of ZrP is reported elsewhere [Citation22]. The direct ion exchange of [Co(bpy)2(NO3)]+ can be acquired in aqueous solution using various ratios of concentration of 10.4 Å hydrated phase and [Co(bpy)2(NO3)]+, stirring the mixture at room temperature so that the ion exchange process can be more effective.
2.5. Characterisation
Single crystal data for the complex were collected on a Bruker APEX II diffractometer equipped with a graphite monochromator and Mo Kα (λ = 0.71073 Å, 296 K) radiation. The structure was solved by direct methods using SHELXS-97, which revealed the positions of almost all the non-hydrogen atoms. Morphological characterisations were performed by transmission electron microscopy (TEM, JEM-2010) operated at 200 kV. Fourier transform infrared spectroscopy (FTIR) was measured by using an Avatar 370 spectrometer (NicoletInstrument Corporation) working in the mid-infrared range from 400 to 4000 cm−1. X-ray diffraction (XRD, D/MAX-PC) analysis was conducted by employing Cu Ka radiation (λ = 1.5418 Å) in the scanning range of 2θ between 10° and 90° with the step size of 0.2° and the counting time of 8 minutes. Raman spectroscopy (excitation wavelength of 633 nm, He-Ne, LabRam-1B, Jobin Yvon) study was performed at room temperature. Steady-state fluorescence emission spectra of the supernatant were performed with a RF-5301 PC fluorescence spectrometer and Leica DMIRB analysis was conducted on U-LH100HG. In order to further demonstrate [Co(bpy)2(NO3)]Cl·3H2O reacting in the ion-exchanged, X-ray photoelectron spectroscopy (XPS, ESCALAB 250) with Al Ka radiation (hν = 1486.6 eV) was conducted.
3. Results and discussion
3.1. Crystal structure of [Co(bpy)2(NO3)]Cl·3H2O
[Co(bpy)2(NO3)]Cl·3H2O crystallises in the P-1 space group with two molecules per unit cell, as depicted in . The lattice parameters for the grown crystal are a = 9.042 Å, b = 9.613 Å, c = 13.008 Å, α = 91.377°, β = 101.854°, γ = 91.515° and the cell volume V = 1105.6 Å3. Analysis of single crystal structure reveals that the precursor ligand, N(CN)2−, is not involved in the construction of the final complex. Actually, the first croci cube grains were determined as Co(bpy)2[N(CN)2]2 by single crystal X-ray diffraction analysis [Citation23]. The orange [Co(bpy)2(NO3)]Cl·3H2O grains are obtained when Co(bpy)2[N(CN)2]2 grains are aged in aqueous solution. It can be speculated that under mild condition N(CN)2− undergoes the oxidation in situ to form NO3−.
3.2. Transmission electron microscope (TEM) and atomic force microscope (AFM) of BN/Ag-NPs
As the TEM image shown in , the BN nanosheet surfaces were decorated with near-spherical Ag-NPs with diameters in the range of about 20–150 nm. The BN nanosheets pile to some extent. Importantly, the Ag nanostructures were found associated with BN nanosheets, suggesting that they were attached to the nanosheets during growth.
An atom force micrograph of BN/Ag-NPs is shown in . The thickness of BN nanosheet was measured to be about 0.94 nm, larger than the value of 0.33 nm referred for the layer–layer distance of BN nanosheets [Citation9].
3.3. Raman and SERS spectra
shows the resonance and surface Raman spectra of [Co(bpy)2(NO3)]Cl·3H2O obtained from excitation at 633 nm. Large increases in the signal-to-noise ratio of the surface spectra are noted for the bands at 1599, 1565, 1489 and 1314 cm−1, respectively, which are assigned to the C−C or C−N stretching of pyridine ring ligand, in-plane vibration of C−H and stretching vibration of NO3− along with moderate increases for the bands of C−N (1327 cm−1), C−O (1037 cm−1), in-plane stretching of pyridine ring (1020 cm−1) and out-of-plane C−H vibration (767 cm−1) [Citation24–Citation26].
The vibration modes of 2,2'-bipyridine ring are always enhanced significantly [Citation22]. In this case, the observed changes in the vibrations assigned to the NO3−stretching mode (1314 cm−1) and C−H in-plane mode (1489 cm−1) suggest that the oxygen and hydrogen atoms are interaction sites. These modes would be perpendicular to the silver surface and are totally symmetric modes, making them ideally suited for enhancement via the electromagnetic effect [Citation27]. The possible geometry of [Co(bpy)2(NO3)]Cl·3H2O molecule adsorbed on Ag-NPs surface with a sloping adsorption configuration is shown in .
3.4. Interactions among of [Co(2,2'-bipyridine)2(NO3)]+ ion-exchanged within zirconium phosphate
The diffraction patterns were measured in the wet sample to avoid dehydration of ZrP. The first-order diffraction peak gives the interlayer distance in this case of 10.4 Å (). [Co(bpy)2(NO3)]+-exchanged ZrP shows a distinctive diffraction peak at 15.9 Å which is indicative of intercalation. When the [Co(bpy)2(NO3)]+ ions exchanged into ZrP, it acts as a wedge. At high loading [Co(bpy)2(NO3)]+, cations serve as pillars keeping the ZrP layers apart and avoiding imperfections on the ZrP stacking. It is important to note that the peak at 10.4 Å exists both in the hydrated non-intercalated phase of 10.4 Å ZrP and in the intercalation product, implying that at this concentration both phases (non-intercalated and intercalated) are present.
In order to further characterise, the ion-exchanged product XPS analysis was performed, as shown in –. A series of binding energy peaks were observed, with Zr3d centred at 183.8, 186.1 and 192 eV, P2p at 134.5 eV, O1s at 532.7 and 532 eV and C1s at 284.6 eV from ZrP sample components, and with Zr3d centred at 182.85 and 185 eV, Cl2p at 199 and 201 eV, P2p at 133.4 eV, N1s at 400 eV, Co2p at 797 and 781.6 eV, O1s at 531.1 eV and C1s at 284.6 eV from ZrP/[Co(bpy)2(NO3)]Cl·3H2O sample components.
Compared to ZrP, Zr3d and P2p states of ZrP/[Co(bpy)2(NO3)]Cl·3H2O exist obvious shifts towards low energy, as shown in . The reason is that the formation of the intercalation product leads to the increase in ZrP interlayer distance to some extent, and further leads to the change in the chemical environment of Zr3+ and PO43− ions.
The presence of N1s (400 eV) and Co2p (797 and 781.6 eV) spectra indicates the formation of the intercalation product, as shown in .
In a solid, the energy level of molecular orbital becomes an energy band [Citation28]. A peak in a valence band spectrum can be regarded as a measure of the magnitude of the first ionisation potential and the density of states [Citation29,Citation30]. As shown in , the peak with the lowest binding energy of ZrP is centred at about 7.0 eV, and that of ZrP/[Co(bpy)2(NO3)]Cl·3H2O at about 5.4 eV. Compared to ZrP, the first ionisation potential of ZrP/[Co(bpy)2(NO3)]Cl·3H2O has a shift towards low energy. This is mainly because that the binding energy decreases with increasing the layer distance after the intercalation product forms.
The IR spectra for ZrP and ZrP/[Co(bpy)2(NO3)]Cl·3H2O are shown in . The appearance of new peaks or large displacements of the existing ones would indicate the changes in the structure of the intercalated molecule or the layered material. For ZrP, the bands at 3510 and 3160 cm−1 have been assigned to the free –OH, H-bonded –OH, intramolecular H-bonded –OH in chelate form and water of crystallisation, respectively [Citation31]. The weak band at 1620 cm−1 is assigned to water bending vibrations. The phosphate groups present bands around 1050 cm−1 are due to orthophosphate vibrations. The band at 1240 cm−1 is attributed to the deformation of the P-O-H bond and the one at 961 cm−1 is corresponding to the phosphate vibrations of the symmetric C3v group. The bands 597, 519 and 400 cm−1 are attributed to phosphate bending motions. In case of [Co(bpy)2(NO3)]+-exchanged ZrP, band at 1455 cm−1 is due to the bipyridine ligand vibrations and the band at 769 cm−1 is assigned to deformation vibrational frequency are expected. The most prominent difference is the vibrational modes of –OH of the ZrP band in the range between 3600 and 3000 cm−1, which is expected because of the heterogeneous environment induced as a consequence of [Co(bpy)2(NO3)]+ cations’ intercalation.
Compared to the results of Yates [Citation32], who obtained an interlayer distance of 15.9 Å for Ru(bpy)32+-cocrystallised ZrP material, the diffraction patterns of the Co(bpy)2NO3+-exchanged ZrP complete agreement with those. Representation of two different zirconium phosphatephases is shown in . ZrP-type layers are made of nearly perfect planes of Zr atom shaving bridging O3PO−groups above and below each plane. It is obvious to know that inter-lamellar spacing of Co(bpy)2NO3+-exchanged ZrP (d = 15.85 Å) is much bigger than ZrP (d = 10.39 Å). It reveals that the direct ion Co(bpy)2NO3+ enters into ZrP framework and the dimension of Co(bpy)2NO3+ is about 5.46 Å.
Figure 11. Idealised representation of two different zirconium phosphatephases: ZrP and Co(bpy)2NO3+-exchanged ZrP.
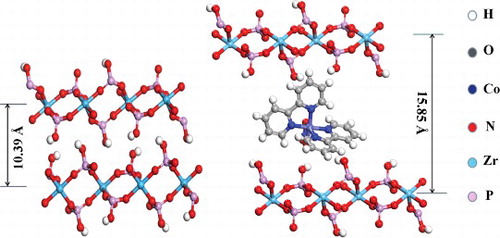
shows the luminescence spectra of [Co(bpy)2(NO3)]+-exchanged ZrP powders suspended in water (0.08% w/w). The luminescence spectra of [Co(bpy)2(NO3)]+-exchanged ZrP resemble that of [Co(bpy)2(NO3)]Cl·3H2O aqueous solution, only with a slight red shift of λmax from 416 nm in aqueous solution to 421 nm in the exchanged materials. The similarity of the λmax values is indicative of the polar microenvironment experienced by the [Co(bpy)2(NO3)]+ probes in the interlayer region, which is consistent with the highly hydrated state of the ZrP and with the ionic nature of the lattice. However, there is a significant increase in the luminescence intensity of [Co(bpy)2(NO3)]+-exchanged ZrP. The emission for [Co(bpy)2(NO3)]+-exchanged ZrP is about 100 times higher than that of [Co(bpy)2(NO3)]+ in solution. Similar luminescence phenomena have been observed in Ru(bpy)32+-exchanged ZrP and zeolite-entrapped Ru(bpy)32+ materials [Citation22,Citation33].
Figure 12. Fluorescence spectra excited at 367 nm. (A) [Co(bpy)2(NO3)]Cl·3H2O aqueous solution, 9 × 10−4 M; (B) [Co(bpy)2(NO3)]+-exchanged ZrP, 9 × 10−4 M; (C) [Co(bpy)2(NO3)]+-exchanged ZrP, 2 × 10−3 M.
![Figure 12. Fluorescence spectra excited at 367 nm. (A) [Co(bpy)2(NO3)]Cl·3H2O aqueous solution, 9 × 10−4 M; (B) [Co(bpy)2(NO3)]+-exchanged ZrP, 9 × 10−4 M; (C) [Co(bpy)2(NO3)]+-exchanged ZrP, 2 × 10−3 M.](/cms/asset/89bfffd2-4140-4a5b-8216-d63b7e4b3919/tjen_a_1301684_f0012_oc.jpg)
Formal descriptions of the low-lying excited states of inorganic complexes containing organic ligands can be derived from successive one-electron promotions. There are basically four orbital types expected, that is, dd states, dπ* states, ππ* states and πd states [Citation34]. In context of the luminescence complexes of the 3d elements, the dπ* states play a major role in the emission. dπ* states arise from the excitation of a metal (t2) electron to a π* antibonding orbital located on the ligand system. This process can be seen as a transfer of electronic charge from the central ion to the ligands, i.e. as an incipient oxidation of the metal ion (MLCT).
At low loading levels, the structure of the ion-exchanged [Co(bpy)2(NO3)]+ is the same as that in solution, indicating that the ZrP framework does not perturb the molecule. However, the microenvironment experienced by the [Co(bpy)2(NO3)]+ probes in the interlayer region has changed in contrast with solution. [Co(bpy)2(NO3)]+ cations are in the state of free arrangement in the solution. The collision among the cations leads to the energy loss. In this case, the cations are usually in the relatively stable excited states. In the interlayer region with ionic nature of the lattice, the cations are in the more ordered states. The ‘masking’ effect of ZrP framework needs to be responsible for the decrease in the energy loss caused by the mutual collision among cations. The exited states of chromophore may have high energy and low stability, giving rise to the increase in luminescence intensity of [Co(bpy)2(NO3)]+ two-dimensional arrangements within the layers of zirconium phosphate.
4. Conclusions
The boron nitride nanosheets/silver nanoparticles/[Co(bpy)2(NO3)]Cl·3H2O nanoaggregate was prepared. According to the SERS analysis, we can infer that the adsorption orientation of [Co(bpy)2(NO3)]Cl·3H2O molecule plane exhibits an inclined orientation with respect to the surface of Ag-NPs.
The intercalation of [Co(bpy)2(NO3)]+ within the layers of ZrP influences its photophysical properties. Steady-state luminescence spectrum of the [Co(bpy)2(NO3)]+-exchanged ZrP materials shows an increase in the luminescence intensity as compared to the aqueous solution of [Co(bpy)2(NO3)]Cl·3H2O. This effect is attributed to the change in the microenvironment of the emissive excited state in the interlayer region with ionic nature of the lattice.
References
- Yang GH, Zhu CZ, Du D, et al. Graphene-like two-dimensional layered nanomaterials: applications in biosensors and nanomedicine. Nanoscale. 2015;7:14217–14231.
- Tang Q, Zhou Z. Graphene-analogous low-dimensional materials. Prog Mater Sci. 2013;58:1244–1315.
- Geim AK. Graphene: status and prospects. Science. 2009;324:1530–1534.
- Zhang YB, Ta YW, Stormer HL, et al. Experimental observation of the quantum Hall effect and Berry's phase in grapheme. Nature. 2005;438:201–204.
- Roy AK, Park B, Lee KS, et al. Boron nitride nanosheets decorated with silver nanoparticles through mussel-inspired chemistry of dopamine. Nanotechnology. 2014;25:445603–445609.
- Singhal SK. Gold-nanoparticle-decorated boron nitride nanosheets: structure and optical properties. Part Part Syst Char. 2013;30:445–452.
- Shen H. Facile in situ synthesis of silver nanoparticles on boron nitride nanosheets with enhanced catalytic performance. J Mater Chem A. 2015;3:16663–16669.
- Roy AK, Park SY, In I. Mussel-inspired synthesis of boron nitride nanosheet-supported gold nanoparticles and their application for catalytic reduction of 4-nitrophenol. Nanotechnology. 2015;26:105601–105609.
- Lin Y, Bunker CE, Shiral Fernando KA, et al. Aqueously dispersed silver nanoparticle-decorated boron nitride nanosheets for reusable, thermal oxidation-resistant surface enhanced Raman spectroscopy (SERS) devices. ACS Appl Mater Inter. 2012;4:1110–1117.
- Lee CH, Qin SY, Savaikar Madhusudan A, et al. Room-temperature tunneling behavior of boron nitride nanotubes functionalised with gold quantum dots. Adv Mater. 2013;25:4544–4548.
- Kim YH, Lee HJ, Dutta PK. Zeolite-mediated photochemical charge separation using a surface-entrapped ruthenium-polypyridyl complex. Inorg Chem. 2003;4:4215–4222.
- Ogawa M, Nakamura T, Mori J, et al. Luminescence of tris(2,2'-bipyridine)ruthenium(II) cations ([Ru(bpy)3]2+) adsorbed in mesoporous silica. J Phys Chem B. 2000;104:8554–8556.
- Sykora M, Kincaid JR. Photochemical energy storage in a spatially organised zeolite-based photoredox system. Nature. 1997;387:162–164.
- Nakato T, Sakamoto D, Kuroda K, et al. Synthesis of two types of intercalation compounds of K4Nb6O17 with tris(2,2'-bipyridyl) metal complex ions. Bull Chem Soc Jpn. 1992;65:322–328.
- Kamat PV, Gopida KR, Mukherjee T, et al. Spectral differences between enantiomeric and racemic Ru(bpy)32+ on layered clays: probable causes. J Phys Chem. 1991;95:10009–10018.
- Angel Martí A, Jorge Colón L. Photophysical characterization of the interactions among tris(2,2'-bipyridyl)ruthenium(II) complexes ion-exchanged within zirconium phosphate. Inorg Chem. 2010;49:7298–7303.
- Yuan SW, White D, Mason A, et al. Improving hydrogen adsorption enthalpy through coordinatively unsaturated cobalt in porous polymers. Rapid Commun. 2012;33:407–413.
- Cheng Z, Yang BQ, Yang MP, et al. Benzimidazolylacetate metal complexes as catalysts for carbonylation reactions. Reac Kinet Mech Cat. 2013;110:331–342.
- Qin L, Li YH, Ma PJ, et al. Exploring the effect of chain length of bridging ligands in cobalt(II) coordination polymers based on flexible bis(5,6-dimethylbenzimidazole) ligands: synthesis, crystal structures, fluorescence and catalytic properties. J Mol Struct. 2013;1051:215–220.
- Coronado E, Galán-Mascarós JR, Gómez-García CJ, et al. Molecule-based magnets formed by bimetallic three-dimensional oxalate networks and chiral tris(bipyridyl) complex cations. The series [ZII(bpy)3][ClO4][MIICrIII(ox)3] (ZII = Ru, Fe, Co, and Ni; MII = Mn, Fe, Co, Ni, Cu, and Zn; ox = oxalate dianion). Inorg Chem. 2001;40:113–120.
- Yang SS, Zhang ZC, Zhao J, et al. High surface enhanced Raman scattering activity of BN nanosheets–Ag nanoparticles hybrids. J Alloy Compd. 2014;583:231–236.
- Martí AA, Colón JL. Direct ion exchange of tris(2,2'-bipyridine)ruthenium(II) into an alpha-zirconium phosphate framework. Inorg Chem. 2003;42:2830–2832.
- Huo SH, Zhang ZC, Ma QL. Surface enhanced Raman scattering on SiO2/Ag nanoparticles aggregate and preparation of nitrogen-doped carbon dots by pyrolysis of Co(2,2’-bipyridine)2(dicyanamide)2. J Exp Nanosci. 2016;11:669–680.
- Nag A, Schnick W. Synthesis, crystal structure and thermal behavior of gadolinium dicyanamide dihydrate Gd[N(CN)2]3 2 H2O. Anorg Z Allg Chem. 2006;632:609–614.
- Kashman Y, Neeman I, Lifshitz A. Six new C17-olefinic and acetylenic oxygenated compounds from avocado pear. Israel J Chem. 1969;7:173–176.
- Madarász J, Varga PP, Pokol G. Evolved gas analyses (TG/DTA–MS and TG–FTIR) on dehydration and pyrolysis of magnesium nitrate hexahydrate in air and nitrogen. J Anal Appl Pyrolysis. 2007;79:475–478.
- Persaud I, Grossman WEL. Surface-enhancement Raman scattering of triphenylmethane dyes on colloidal silver. J Raman Spectrosc. 1993;24:107–112.
- Jin LP, Wang DX, Huang LB. Studies on XPS inner shell and valence band spectra of certain crown ether compounds. Chin J Org Chem. 1986;6:439–442.
- Wang DX, Da YX, Wang LB, et al. Studies on the XPS vibration and its valence spectrum of O – nitroacetanilide derivatives. Acta Chim Sinica. 1987;45:77–79.
- Ji HX, Wang DX, Xu GZ, et al. Studies on XPS and valence band spectra of substituted cyclopentadiene titanium, zirconium and hafnium compounds. Chin J Chem Phys. 1992;5:55–59.
- Williams DH, Fleming I. Spectroscopic methods in organic chemistry. Tokyo: McGraw-Hill Book Co Ltd; 1987.
- Yates RC, Kuznicki SM, Lloyd LB, et al. Tris(2,2′-bipyridine) ruthenium(II) electron-transfer in a layered zirconium phosphate lattice. J Inorg Nucl Chem. 1981;43:2355–2358.
- Turbeville W, Robins DS, Dutta PK. Zeolite-entrapped tris(2,2'-bipyridene)ruthenium(II): intermolecular structural and dynamic effects. J Phys Chem. 1992;96:5024–5029.
- Crosby GA. Spectroscopic investigations of excited states of transition-metal complexes. Acc Chem Res. 1975;8:231–238.