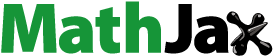
Abstract
In this study, tin nanoparticles were green synthesized using the aqueous extract of Calendula officinalis. Different techniques such as FE-SEM, XRD, FT-IR, and EDS analysis were used to characterize SnNPs@ C. officinalis. A 21.87 nm was obtained for the crystal size of the tin nanoparticles using XRD analysis. The FE-SEM images show a spherical morphology for SnNPs@ C. officinalis with a range size of 21.63–64.07 for the synthetic nanoparticles. The anti-breast cancer effects of biologically synthesized SnNPs@ C. officinalis against breast cancer cell lines were assessed. The anti-breast cancer properties of the SnNPs@ C. officinalis could significantly remove the MCF7, Hs 319.T, and MCF10 cancer cell lines in a time and concentration-dependent manner by MTT assay. The antioxidant activity of SnNPs@ C. officinalis was determined by the DPPH method. The SnNPs@ C. officinalis showed the highest antioxidant activity according to the IC50 value. It seems the anti-human breast cancer effect of recent nanoparticles is due to their antioxidant effects.
1. Introduction
Calendula officinalis is one of the famous medicinal plants from Asteraceae family [Citation1]. The plant is known as an herbal medicinal product according to European Medicines Agency since 2008 [2]. The plant has been used as a potent agent to cure several diseases in different traditional medicines around the world. C. officinalis is used as a sufficient remedy to treat inflammatory, diaphoretic, analgesic, antiseptic, healing wounds, minor burns [Citation2,Citation3]. The ability of the plant to treat various illnesses is contributed to the presence of diverse secondary metabolites in the plant such as phenolic, flavonoids, saponins, carotenes, and mucilage that have been reported previously [Citation4–6]. In the recent years, the C. officinalis extract has used for preventing many cancers [Citation2–7]. The reasons for the clinical trials failure to achieve the desired multilayer results are complex and intertwined. Unfortunately, therapeutic agents (chemotherapy, biology, and nanotechnology) are selective and effective in targeting in vitro cancer cells, and even in proportionate animal specimens [Citation8–11]. However, failing in clinical trials is not a rule but a rule. This is because the biological distribution of therapeutic agents can be a fundamental factor in these fractures. Inadequate concentration at unwanted concentration and target sites elsewhere, leads to dose-limiting poisoning [Citation9,Citation10]. The biological distribution of drug agents is mainly controlled by the drugs’ ability to penetrate natural barriers. Strategy for adding targeting sections to therapeutic nanoparticles to improve location Specification to date, despite 30 years of effort in pharmaceutical companies and many laboratories, it has not yet been able to produce clinically approved drugs. This failure is because the addition of molecular agents increases the targeting of cognitive characteristics. However, it does so in the face of much more incredible difficulty in managing biological barriers [Citation12–17]. Nanoparticles, especially metal nanoparticles and metal oxides, have been widely used by medical consumers and manufacturers. The mechanism of nanoparticle-induced toxicity against cancer cells is the producing of reactive oxygen species (ROS) [Citation18–20]. Excessive production of ROS can lead to oxidative stress, disruption of regular physiological maintenance, and oxidation regulation. These effects in turn lead to DNA damage, unregulated cell signaling pathways, changes in cell evolution, cytotoxicity, apoptotic death, and the onset of cell death [Citation19, Citation21–23]. Critical-deterministic factors can affect the production of ROS. These critical-deterministic factors include shape, size, nanoparticle surface area, particle surface electricity, surface-forming groups, Particle solubility, metal ion emission from nanomaterials and nanoparticles, optical activation, model of cell reactions, inflammatory effects, and ambient pH [Citation18,Citation19, Citation21–23]. As a result, increasing industrial knowledge in the field of scalable nanoparticle synthesis, along with the design of multifunctional nanoparticles, will dramatically change the strategies of microenvironmental preparation and therapeutic-diagnostic nanoparticles for cancer treatment [Citation24–31].
In has been a continuous trend to develop functionalized nanomaterials at an advanced level to improve their physicochemical, catalytic, and biological properties. In this study, we report the biogenic synthesis of Sn NPs using C. officinalis levees extract as a green reducing and stabilizing agent. The as-synthesized SnNPs@ C. officinalis exhibited excellent potential for the treatment of breast cancer
2. Materials and methods
2.1. Preparation and extraction of aqueous extract
To obtain the aqueous extract of the leaves of C. officinalis, 15 g of the dried plant leaves was macerated in 200 mL of the boiling demonized distilled water. After three hours, the extract was filtered and evaporated under low pressure. The concentrated crude extract was put in an oven at 50 °C for 24 h. The extract was a powder in brown color.
2.2. Green synthesis and chemical characterization of SnNPs@ C. officinalis
The green synthesis of tin nanoparticles was run according to a previous study [Citation32]. A 25 mL of C. officinalis extract (2 g in 15 mL of deionized water) was added to 150 mL of SnCl2.2H2O (0.1 M). Then, the reaction mixture was refluxed for 55 min at 90 °C. After that time, the tin nanoparticle was formed. The color-changing from yellow-green to pale yellow indicated the formation of tin nanoparticles. The obtained SnNPs were washed three times with water and ethanol, and then centrifuged at 12,000 rpm for 15 min. Finally, the residue was dried in an oven at 50 °C. The synthesized nanoparticles as a yellow powder were kept in a vial for chemical characterization and biological activity evaluation.
2.3. DPPH assay protocol
In the recent study, 1 ml of DPPH methanol solution was added to 3 ml of nanoparticles and the resulting mixture was stirred vigorously. The test tubes were placed in a dark place for 30 min. Then, the absorbance at the wavelength of 517 nm was read. It should be noted that in the control sample, the nanoparticles was replaced with 3 ml of methanol. Finally, the DPPH radical's inhibition percentage was calculated with this formula [Citation33–37]:
DPPH is a free radical that changes color in the presence of substances with antioxidant properties and captures electrons. Yellow to purple color change is the basis of antioxidant properties. Solutions with different concentrations (10–1000 μg/ml) were prepared from phenolic powder and BHT synthetic antioxidant in methanol solvent [Citation33–37].
2.4. MTT Assay protocol
In this study, the anticancer effects of SnNPs@C. officinalis against the breast cancer cells (MCF7, Hs 319.T, and MCF10 ) were investigated. These cells in DMEM culture medium (Gibco, USA) with 10% FBS (Gibco, USA) and penicillin/streptomycin (100 μL/100 μg/ml) in an incubator containing 5% Carbon dioxide with 90% humidity was stored at 37 °C. Then, when about 80% of the flask was filled, cell passage was performed and about 5 × 104 cells (per square centimeter) were placed in 24 house bacterial petri dishes in the usual environment. The cells were treated with different concentrations of nanoparticles 24 h later and kept in this condition for 3 days. The survival rate of cultured cells was prepared with different concentrations. In this experiment, cells were cultured at 3 × 104 cells/well in 24-well plates and kept in an incubator at 37 °C for 24 h. Then the old culture medium was taken out of the wells, and the cells were treated with different concentrations of nanoxidro. This test was performed on the first, second, and third days after exposing the cells to the compounds; thus, at the appropriate time after culturing the cells in plates of 24 cells, the culture medium was removed and about 300 μl of fresh medium containing 30 μl of MTT solution was added to each cell. After 3 and 4 h of incubation at 37 °C, MTT solution is removed, and 200 μl (Dimethyl Sulfoxide, Merck, USA, 100%) DMSO is added to each house. Then, the sample absorption was read at 570 wavelengths using ELISA rider (Expert 96, Asys Hitch, Ec Austria). This experiment was repeated 3 times and each time, four wells were considered for each nano oxide concentration. Cell survival percentage was evaluated by the following formula [Citation38]:
2.5. Statistical analysis
The results were evaluated as Mean ± SE using the SPSS software version 12 and statistical tests of variance of completely randomized block design. Drawing graphs in Excel software was performed, and the significance level of the differences was considered p < 0.01.
3. Results and discussion
3.1. Chemical characterization of SnNPs@C. officinalis
3.1.1. FT-IR analysis
FT-IR spectroscopy is well known as a good qualitative technique to characterize green synthetic metallic nanoparticles. The peaks from 400 to 700 cm−1 are typically related to metal-oxygen bonds. The peaks after 1000 cm−1 are usually correlated to the different functional groups of organic compounds that play capping and reducing roles in synthesizing of metallic nanoparticles. shows the FT-IR spectra of SnNPs@C. officinalis. The peaks at wavenumbers of 430,597 and 661 cm−1 belong to the Sn-O bond. A previous study has reported the peaks for green synthetic tin nanoparticles with a bit of difference in wavenumbers [Citation39]. There peaks such as those at 1031 cm−1 (for the stretching vibration of C–O), 1413to 1722 cm−1 (for the stretching vibration of C = C and C = O s), 3336(for the stretching vibration of O–H), and 2927 cm−1 (for the stretching vibration of O–H and C–H) correlate to the different functional groups of organic compounds that are known as the plant secondary metabolites [Citation4–6].
3.1.2. UV–Visible spectroscopy analysis
The UV–Visible spectrum of SnNPs@C. officinalis is presented in . The spectrum shows the surface Plasmon resonance (SPR) of tin nanoparticles. The formation of SnNPs@C. officinalis was observed. There was an advanced SPR band at the wavelength of 222 nm that confirmed the formation of SnNPs@C. officinalis. This band has been reported previously for green synthesis of tin nanoparticles [Citation40].
3.1.3. EDS analysis
The EDS analysis of metallic nanoparticles is another qualitative technique for the characterization evaluating of metallic nanoparticles. The method reveals the elemental analysis of the nanoparticles. exhibits the EDS analysis of SnNPs@C. officinalis. The signals at 0.51Kev for SnLα, 5.97 for SnKα, and 6.09 for SnKβ approve the presence of tin in the nanoparticles. These signals for the green synthetic of tin nanoparticles have been reported previously [Citation39]. The presence of the signals for carbon and oxygen confirms the linkage of organic compounds to the surface of SnNPs@C. officinalis.
3.1.4. SEM analysis
The morphology and particle size are two characteristics of metallic nanoparticles that are evaluated using the FE-SEM technique. The FE-SEM images of SnNPs@C. officinalis are presented if . The images show a spherical morphology for SnNPs@C. officinalis with particles size in the range of 21.63 to 64.07 nm. SnNPs@C. officinalis similar to other metallic nanoparticles such as tin, tin, zinc, and nickel, which have been green synthesized previously, tended to aggregate [Citation32, Citation40–44]. A range size of 1 to 70 nm for the green synthesized tin nanoparticles has been reported in the literature [Citation32, Citation39,Citation40, Citation45,Citation46].
3.1.5. XRD analysis
XRD is known as the most popular technique to evaluate the crystallinity of the metallic nanoparticles. The XRD pattern SnNPs@C. officinalis is shown in . The result confirms the formation of SnNPs@C. officinalis with small crystal size and well crystallizing. The 2θ values for different planes are well matched to the standard database of ICDD PDF card no. 96-412-4668. The signals at 2θ values of 26.62, 33.93, 51.79, 54.82, 61.98, 64.69, 65.67 and 71.19 are indexed as (111), (110), (021), (003), (022), (013), (122) and (220) planes. Similar data have been reported for green synthetic tin nanoparticles previously [Citation39]. An 18.13 nm was obtained for the crystal size of SnNPs@C. officinalis using Scherer’s equation.
Regarding cancer, efforts have been made to use innovative nanomaterials (nanoparticles, nanostructures), which have a more remarkable ability to target cancer cells, to treat such patients. They kill malignant cells by irradiating them, providing a microscopic therapeutic effect within electrons [Citation8–12]. Nanoparticles are programmed to achieve optimal therapeutic efficacy, delivering therapeutic loads to target cells. Studies have also been performed on several nanocarriers based on lipids, polymers, and peptides for delivery to the respiratory system [Citation13–16]. Properties of nanoparticles for targeted delivery of nanoparticles to tumors motivate for targeted drug delivery in cancer treatment to kill cancer cells. In a way, that has the most minor damage to healthy cells [Citation16,Citation17]. One of the nanotechnology goals is to mount drugs on carriers, send them and release them into the target cell, which is called targeted drug delivery. Using nanoparticles, the drug can be intelligently delivered to the desired tissue, and improve the tissue without damaging other tissues [Citation15–17]. The scavenging capacity of SnNPs@C.officinalis and BHT at different concentrations expressed as percentage inhibition has been indicated in and . The nanoparticles showed a higher radical scavenging activity than the selected positive control. SnNPs@C.officinalis scavenged DPPH with the IC50 of 157 ± 0 µg/ml which is less than that of BHT.
Table 1. The antioxidant activities of butylated hydroxyl toluene, C. officinalis, SnCl2, and tin nanoparticles against DPPH.
Table 2. The IC50 of butylated hydroxyl toluene, C. officinalis, SnCl2, and tin nanoparticles in the antioxidant test.
In this study, the treated cells with different concentrations of the present SnNPs@ C. officinalis were assessed by MTT assay for 48 h about the cytotoxicity properties on normal (HUVEC) and breast malignancy cell lines, that is, MCF7, Hs 319.T, and MCF10. The absorbance rate was evaluated at 570 nm, which representing viability on the normal cell line (HUVEC) even up to 1000 μg/mL for SnNPs@C. officinalis ( and ). The viability of malignant breast cell line reduced dose-dependently in the presence of SnNPs@C. officinalis. The IC50 of SnNPs@C. officinalis were 132, 126, and 119 µg/mL against MCF7, Hs 319.T, and MCF10 cell lines, respectively ( and ).
Table 3. The anti-breast cancer properties of C. officinalis, SnCl2, and tin nanoparticles against human breast cancer cell lines.
Table 4. The IC50 of C. officinalis, SnCl2, and tin nanoparticles in cytotoxicity and anti-breast cancer tests.
4. Conclusion
In Conclusion, the tin nanoparticles were synthesized using the aqueous extract of a medicinal plant, namely C. officinalis. The nanoparticles were characterized using different techniques, including FT-IR, XRD, FE-SEM, and EDS analysis. SnNPs@ C. officinalis were green synthesized in a spherical morphology with a range size of 21.63 to 64.07 nm. The viability of malignant cancer cell lines was reduced dose-dependently in the presence of SnNPs@C. officinalis. The nanoparticles showed the best antioxidant activities against DPPH. So, the findings of the recent research show that biologically synthesized SnNPs@C. officinalis might be used to cure breast cancer. In addition, the current study offers that SnNPs@C. officinalis could be a new potential adjuvant chemopreventive and chemotherapeutic agent against cytotoxic cells.
Data availability statement
Data available on request from the authors.
References
- AshwlayanVD KA, Verma M. Therapeutic potential of Calendula officinalis. Pharm Pharmacol Int J. 2018;6:149–155.
- Cruceriu D, Balacescu O, Rakosy E. Calendula officinalis: potential roles in cancer treatment and palliative care. Integr Cancer Ther. 2018;17(4):1068–1078.
- Shahen MZ, Mahmud S, Rony M, et al. Effect of antibiotic susceptibility and inhibitory activity for the control of growth and survival of microorganisms of extracts of Calendula officinalis. EJMHS. 2019;1(3):1–9.
- Verma PK, Raina R, Agarwal S, et al. Phytochemical ingredients and pharmacological potential of Calendula officinalis linn. PBR. 2018;4:1–17.
- de Oliveira Carvalho H, Góes LDM, Cunha NMB, et al. Development and standardization of capsules and tablets containing Calendula officinalis L. hydroethanolic extract. Revista Latinoamericana de Química. 2018;46:16–27.
- Długosz ] M, Markowski M, Pączkowski C. Source of nitrogen as a factor limiting saponin production by hairy root and suspension cultures of Calendula officinalis L. Acta Physiol Plant. 2018;40(2):35.
- Sui Y, Xie L, Meng D, et al. Cardiovascular protective properties of green synthesised iron nanoparticles from Calendula officinalis leaf aqueous extract on mitoxantrone-induced DNA fragmentation and apoptosis in HDMVECn, HUVEC, HAEC, HCAEC, HCASMC and HPAEC cells. J Exp Nanosci. 2022;17(1):126–137.
- Stewart B, C.P W. World Cancer Report 2014. International Agency for Research on Cancer World Health Organization; Lyon, France: 2014 [cited 24 Mar 2015]. Available from http://www.iarc.fr/en/publications/books/wcr/wcr-order.php.
- Rasmussen JW, Martinez E, Louka P, et al. Zinc oxide nanoparticles for selective destruction of tumor cells and potential for drug delivery applications. Exp Opin Drug Deliv. 2010;7(9):1063–1077.
- Felice B, Prabhakaran MP, Rodríguez AP, et al. Drug delivery vehicles on a nano-engineering perspective. Mater Sci Eng C. 2014;41:178–195.
- Fernandes E, Ferreira JA, Peixoto A, et al. New trends in guided nanotherapies for digestive cancers: a systematic review. J Control Release. 2015;209:288–307.
- Caputo F, de Nicola M, Ghibelli L. Pharmacological potential of bioactive engineered nanomaterials. Biochem Pharmacol. 2014;92(1):112–130.
- Danhier F, Feron O, Preat V. To exploit the tumor microenvironment: passive and active tumor targeting of nanocarriers for anti-cancer drug delivery. J Control Release. 2010;148(2):135–146.
- Laurent S, Dutz S, Häfeli UO, et al. Magnetic fluid hyperthermia: focus on superparamagnetic iron oxide nanoparticles. Adv Colloid Interface Sci. 2011;166(1-2):8–23.
- Maier-Hauff K, Ulrich F, Nestler D, et al. Efficacy and safety of intratumoral thermotherapy using magnetic iron-oxide nanoparticles combined with external beam radiotherapy on patients with recurrent glioblastoma multiforme. J Neurooncol. 2011;103(2):317–324.
- Kolosnjaj-Tabi J, di Corato R, Lartigue L, et al. Heat-generating iron oxide nanocubes: Subtle “destructurators” of the tumoral microenvironment. ACS Nano. 2014;8(5):4268–4283.
- Bhattacharyya S, Kudgus RA, Bhattacharya R, et al. Inorganic nanoparticles in cancer therapy. Pharm Res. 2011;28(2):237–259.
- Johannsen M, Thiesen B, Wust P, et al. Magnetic nanoparticle hyperthermia for prostate cancer. Int J Hyperthermia. 2010;26(8):790–795.
- Bañobre-López M, Teijeiro A, Rivas J. Magnetic nanoparticle-based hyper-thermia for cancer treatment. Rep Pract Oncol Radiother. 2013;18(6):397–400.
- Huang Y, Zhu C, Xie R, et al. Green synthesis of nickel nanoparticles using fumaria officinalis as a novel chemotherapeutic drug for the treatment of ovarian cancer. J Exp Nanosci. 2021;16(1):368–381.
- Klein S, Sommer A, Distel LV, et al. Superparamagnetic iron oxide nanoparticles as novel X-ray enhancer for low-dose radiation therapy. J Phys Chem B. 2014;118(23):6159–6166.
- Chatterjee DK, Fong LS, Zhang Y. Nanoparticles in photodynamic therapy: an emerging paradigm. Adv Drug Deliv Rev. 2008;60(15):1627–1637.
- Zhang AP, Sun YP. Photocatalytic killing effect of TiO2 nanoparticles on Ls- 174-t human Colon carcinoma cells. World J Gastroenterol. 2004;10(21):3191–3193.
- Ali D, Alarifi S, Alkahtani S, et al. Cerium oxide nanoparticles induce oxidative stress and genotoxicity in human skin melanoma cells. Cell Biochem Biophys. 2015;71(3):1643–1651.
- Neri D, Supuran CT. Interfering with pH regulation in tumors as a therapeutic strategy. Nat Rev Drug Discov. 2011;10(10):767–777.
- Seo JW, Chung H, Kim MY, et al. Development of water-soluble single-crystalline TiO2 nanoparticles for photocatalytic cancer-cell treatment . Small. 2007;3(5):850–853.
- Hou Z, Zhang Y, Deng K, et al. UV-emitting upconversion-based TiO2 photosensitizing nanoplatform: near-infrared light mediated in vivo photodynamic therapy via mitochondria-involved apoptosis pathway. ACS Nano. 2015;9(3):2584–2599.
- Cui S, Yin D, Chen Y, et al. In vivo targeted deep-tissue photodynamic therapy based on near-infrared light triggered upconversion nanoconstruct. ACS Nano. 2013;7(1):676–688.
- Lucky SS, Idris NM, Li Z, et al. Titania coated upconversion nanoparticles for near-infrared light triggered photodynamic therapy. ACS Nano. 2015;9(1):191–205.
- Idris NM, Lucky SS, Li Z, et al. Photoactivation of core-shell titania coated upconversion nanoparticles and their effect on cell death. J Mater Chem B. 2014;2(40):7017–7026.
- Sutradhar P, Saha M. Green synthesis of zinc oxide nanoparticles using tomato (Lycopersicon esculentum) extract and its photovoltaic application. J Exp Nanosci. 2016;11(5):314–327.
- Ahmeda A, Mahdavi B, Zaker F, et al. Chemical characterization and anti‐hemolytic anemia potentials of tin nanoparticles synthesized by a green approach for bioremediation applications. Appl Organometal Chem. 2020;34(3): e5433.
- Chen J, Patil S, Seal S, et al. Rare earth nanoparticles prevent retinal degeneration induced by intracellular peroxides. Nat Nanotechnol. 2006;1(2):142–150.
- Das M, Patil S, Bhargava N, et al. Auto-catalytic ceria nanoparticles offer neuroprotection to adult rat spinal cord neurons. Biomaterials. 2007;28(10):1918–1925.
- Korsvik C, Patil S, Seal S, et al. Superoxide dismutase mimetic properties exhibited by vacancy engineered ceria nanoparticles. Chem Commun. 2007;(10):1056–1058.
- Hong R, Han G, Fernandez JM, et al. Glutathione-mediated delivery and release using monolayer protected nanoparticle carriers. J Am Chem Soc. 2006;128(4):1078–1079.
- Zangeneh MM, Zangeneh A, Pirabbasi E, et al. Falcaria vulgaris leaf aqueous extract mediated synthesis of iron nanoparticles and their therapeutic potentials under in vitro and in vivo condition. Appl. Organometal. Chem. 2019;33:e5246.
- Jalalvand AR, Zhaleh M, Goorani S, et al. Chemical characterization and antioxidant, cytotoxic, antibacterial, and antifungal properties of ethanolic extract of allium saralicum RM fritsch leaves rich in linolenic acid, methyl ester. J Photochem Photobiol B. 2019;192:103–112.
- Li C, Zhang Y, Li M, et al. Fumaria officinalis-assisted synthesis of tin nanoparticles as an anti-human gastric cancer agent. Arabian J Chem. 2021;14(10):103309.
- Souri M, Hoseinpour V, Ghaemi N, et al. Procedure optimization for green synthesis of tin dioxide nanoparticles by yucca gloriosa leaf extract. Int Nano Lett. 2019;9(1):73–81.
- Mahdavi B, Paydarfard S, Zangeneh MM, et al. Assessment of antioxidant, cytotoxicity, antibacterial, antifungal, and cutaneous wound healing activities of green synthesized manganese nanoparticles using ziziphora clinopodioides lam leaves under in vitro and in vivo condition. Appl Organometal Chem. 2020;34(1):e5248.
- Mahdavi B, Saneei S, Qorbani M, et al. Ziziphora clinopodioides lam leaves aqueous extract mediated synthesis of zinc nanoparticles and their antibacterial, antifungal, cytotoxicity, antioxidant, and cutaneous wound healing properties under in vitro and in vivo conditions. Appl Organometal Chem. 2019;33(11):e5164.
- Mahdavi B, Paydarfard S, Rezaei‐Seresht E, et al. Green synthesis of NiONPs using trigonella subenervis extract and its applications as a highly efficient electrochemical sensor, catalyst, and antibacterial agent. Appl Organomet Chem. 2021;35(8):e6264.
- Yuan C, Jiang B, Xu X, et al. Anti-human ovarian cancer and cytotoxicity effects of nickel nanoparticles green-synthesized by alhagi maurorum leaf aqueous extract. J Exp Nanosci. 2022;17(1):113–125.
- Ogunyemi SO, Zhang F, Abdallah Y, et al. Biosynthesis and characterization of magnesium oxide and manganese dioxide nanoparticles using Matricaria chamomilla L. extract and its inhibitory effect on Acidovorax oryzae strain RS-2. Artif Cells Nanomed Biotechnol. 2019;47(1):2230–2239.
- Moon SA, Salunke BK, Saha P, et al. Comparison of dye degradation potential of biosynthesized copper oxide, tin dioxide, and silver nanoparticles using kalopanax pictus plant extract. Korean J Chem Eng. 2018;35(3):702–708.