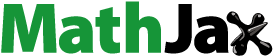
Abstract
Silver nanoparticles (AgNPs) are used in biomedicine applications. Other drugs combined with the AgNPs can improve efficacy in the treatment of diseases, and most such studies have focused on antibiotics. We determined the synergistic effects of Phyllanthus emblica-derived AgNPs in combination with Vatica diospyroides cotyledon extracts (VCE) against bacteria using agar well diffusion, broth microdilution, and minimum inhibitory concentration (MIC). Synergy of AgNPs and VCE was confirmed with the fractional inhibitory concentration index (FICI). To evaluate patterns of bacterial death, flow cytometry and electron microscopy were used. We found that the effective incubation time of AgNPs against bacteria was highly variable. Increasing AgNPs in the combination influenced antibacterial activity against Staphylococcus aureus and Bacillus subtilis. The MIC values interpreted through FICI showed synergy against S. aureus and indifference against B. subtilis. Flow cytometric profiles confirmed that the fraction of S. aureus that respond to a combination of VCE with AgNPs increased in dose-dependent manner. The response patterns of bacteria proceeded simultaneously as the cells lost intracellular components and suffered membrane damage. Synergy of AgNPs with a plant extract has become a promising approach, as green AgNPs and plant extracts are biocompatible and cost-effective resources that can utilized for the treatment of bacterial infectious diseases.
1. Introduction
The development of safe and effective bactericides is pursued in an effort to improve infectious disease treatments. Several studies have reported that some medicinal plant extracts can inhibit the growth and kill Gram-positive and Gram-negative bacteria by affecting the cell membranes of a wide range of pathogenic bacteria, such as food pathogens and poultry gastrointestinal tract pathogens [Citation1,Citation2]. In many reports, a few plant species have been tested for various antimicrobial properties specified by bacterial species, and typically only high concentrations of plant extracts can kill bacteria [Citation3,Citation4]. Interestingly, it is difficult for the bacteria to develop a resistance to the plant extracts because many involved targets are hit by several active compounds [Citation5].
Nanoparticles (NPs) with smallest dimension in the range 1–100 nm have general antibacterial properties against a wide range of bacteria, via mechanisms such as oxidative stress induction, metal ion release, and non-oxidative mechanisms [Citation6]. Unfortunately, some potential risks in the use of NPs have been monitored and reported. Asare et al. [Citation7] reported that normal cell functions were inhibited and DNA damage in human cells increased with prolonged exposure to a high concentration of NPs. Moreover, it is possible that bacteria may develop resistance to any nanoparticle upon continuous exposure to the NPs alone [Citation8]. Thus, efficacy at a low concentration and short time of exposure should be proven for candidate NPs.
Interestingly, biosynthesized NPs have been confirmed as biologically safer and more environment-friendly than NPs from chemical synthesis [Citation9]. Among the NPs synthesized, AgNPs are considered promising antibacterial agents with emphasis on bactericides [Citation10]. Recently, AgNPs have been synthesized using various plant species, which has become a promising alternative to the chemical method [Citation11,Citation12]. Phyllanthus emblica is commonly known as an important herbal drug widely used in Thai traditiobal medicine. AgNPs can be formed by adding silver nitrate to extracts of P. emblica fruit without using reducing agents or stabilizers [Citation13]. Although there have been reports of antibacterial combinations of NPs and antibiotics [Citation14–18], the efficacy of NPs in combination with a plant extract has never been described.
In our previous study, antibacterial activities of antibiotics increased when combined with fruit extract of Vatica diospyroides, an endemic woody species in Dipterocarpaceae, which is known for potent medicinal properties [Citation19]. We thus recommended the use of V. diospyroides fruit extract to inhibit bacterial species by combining it with biosynthesized AgNPs. To enable an alternative approach in controlling infectious diseases, this study suggests the synergistic use of AgNPs with V. diospyroides fruit extract, to inhibit the growth of pathogenic bacteria.
2. Materials and methods
2.1. Plant materials, chemicals and bacterial strains
Vatica diospyroides Symington fruit were collected from Khian Sa district in Surat Thani province, whereas the Phyllanthus emblica L. fruit were collected from Prince of Songkla University, Surat Thani campus, in Thailand. Silver nitrate (AgNO3-, +99.9% POCH CAS no. 7761-88-8, POLAND) was used as precursor in the preparation of silver nanoparticles (AgNPs). Four strains of bacteria, namely Staphylococcus aureus ATCC 25923, Bacillus subtilis ATCC 6633, Escherichia coli ATCC 25922 and Psuedomonas aeruginosa ATCC 27853, were prepared and maintained at the Scientific Laboratory and Equipment Center (SLEC), Prince of Songkla University, Surat Thani campus, Thailand.
2.2. Preparation of V. diospyroides and P. emblica fruit extracts
V. diospyroides cotyledon extraction (VCE) followed the method described previously [Citation20]. Briefly, the cotyledon was separated from entire fruit and sliced into small pieces prior to drying. After the drying, acetone ((CH3)2CO) was used as the solvent in maceration. The pieces of dried cotyledon (270 g) were macerated for 5 days at room temperature. After that, the crude extract was prepared by evaporating the solvent used. Dried residue was obtained and stored in refrigulator (4 °C). The method used to prepare P. emblica fruit extract followed Ramesh et al. [Citation21]. The dried fruit materials (25 g) were extracted with boiling water (100 mL) for 10 min, and then were filtered twice using Whatman filter paper No.1 resulting in a 250 mg/mL final concentration. The aqueous extract was stored in dark at 4 °C prior to use as stabilizer and reducer in AgNPs synthesis.
2.3. Synthesis of AgNPs using P. emblica fruit extracts
A 10 mL sample of the aqueous fruit extract of P. emblica was added to 100 mL AgNO3- (169 µg/mL). The mixture was incubated for 0.5–24 h at room temperature, and thereafter AgNPs were evaluated using UV-visible spectroscopy. The confirmation of synthesis was performed on GENESYS 10S UV-Vis Spectrophotometer (Thermo Scientific, USA) over wavelengths from 300 to 700 nm at a resolution of 1 nm. The synthesized AgNPs were in the absorbance range of 425–436 nm [Citation21].
2.4. Antibacterial effect dependence on incubation time and concentration of synthesized AgNPs, P. emblica fruit extract, AgNO3– or a combination of VCE and AgNPs
The AgNPs synthesized with various times of incubation and the optimum AgNPs concentration against four strains bacteria S. aureus, B. subtilis, E. coli and P. aeruginosa were investigated using an agar well diffusion assay [Citation22]. P. emblica fruit extract, AgNO3- and synthesized AgNPs were each evaluated in terms of antibacterial function. The potential antibacterial properties were assessed from zones of inhibition. The optimal time of AgNPs incubation was chosen to achieve sufficient AgNPs concentration against all tested bacteria. Briefly, 108 cfu/mL of each bacterial culture was spread on nutrient agar plates with a sterile swab. A 6 mm diameter cork borer was punched into the agar medium and the hole was filled with 100 μL of pure but diluted AgNPs. The potential antibacterial properties of AgNPs were evaluated after incubation. Effects of combining VCE and AgNPs were also tested as described above.
2.5. Determination of minimal inhibitory concentration (MIC)
Minimum Inhibitory Concentrations (MICs) of tested substances, namely AgNPs and VCE, and synergistic effects of both substances against the bacteria were tested by broth microdilution and by Resazurin-based 96-well microdilution [Citation23]. The first well had 280 µL of mixed solution with Mueller Hinton Broth (MHB) and twofold amount of the tested substances. Wells 2–12 had 140 µL MHB. To make twofold serial microdilution, 140 µL aliquot from the first well was pipetted and added into the next well and mixed, repeating up to well 10. Wells 11 and 12 had negative (10% Dimethyl sulfoxide) and positive (30 µg/mL Ciprofloxacin) controls, respectively. 10 µL of 6.75 mg/mL Resazurin was added into each well prior to adding 50 µL of bacterial suspension. The inoculated plate was incubated at 37 °C for 24 h. After incubation, on evaluation of color, MIC was defined as the lowest concentration such that the resazurin color did not change from blue to pink.
2.6. Fractional inhibitory concentration index (FICI)
The synergy between VCE and AgNPs was assessed from the fractional inhibitory concentration index (FICI). The FIC was calculated as the MIC of VCE and AgNPs in combination, divided by the MIC of VCE or of AgNPs singly. FIC index indicates the interactions as follows: FICI < 0.5 indicates synergy, 0.5 <FICI <1 indicates partial synergy, FICI = 1 indicates additivity, 1 < FICI < 4 indicates indifferent, and FICI > 4 indicates antagonistic [Citation24].
FICI = FIC of VCE (1) + FIC of AgNPs (2)(1)
(1)
(2)
(2)
2.7. Flow cytometric (FCM) analysis and electron microscopy (EM)
The final concentrations that bacteria respond to, namely 0.5 × MIC (1.48 µg/mL of AgNPs: 250 µg/mL of VCE), MIC (2.96 µg/mL of AgNPs: 500 µg/mL of VCE), and 2 × MIC (5.92 µg/mL of AgNPs: 1,000 µg/mL of VCE) for S. aureus and 0.5 × MIC (0.37 µg/mL of AgNPs: 62.5 µg/mL of VCE), MIC (0.74 µg/mL of AgNPs: 125 µg/mL of VCE), and 2 × MIC (1.48 µg/mL of AgNPs: 250 µg/mL of VCE) for B. subtilis were added to wells containing 5 × 106 CFU/mL and incubated at 37 °C for 0–24 h. The membrane integrity and the complete granularity of bacteria were measured by using flow cytometry and electron microscopy. Briefly, after incubation with the synergistic combination, the cells were washed and re-suspended in 950 µL of phosphate-buffered saline (PBS). For FCM analysis, propidium iodide (PI) staining was performed prior to analyzing bacterial response profiles (fluorescence intensity; FL2 area and side scatter; SSC) on a BD FACSCalibur flow cytometer (Becton Dickinson Biosciences, San Jose, CA, USA). The populations of viable cells, membrane-damaged cells, injured cells and dead cells in each sample were analyzed using WinMDI version 2.9 software (Scripps Institute, La Jolla, CA) [Citation20]. For scanning and transmission EM, after incubation with the same combination as in the FCM test, 2.5% glutaraldehyde was added and mixed and then incubated at 4 °C for 1 h. The mixtures were centrifuged at 8000 rpm for 10 min, and then washed twice with PBS followed by resuspension in 950 µL of PBS. Characteristics of membrane integrity and complete granularity of bacteria were observed by Transmission EM (TEM) and Scanning EM (SEM), respectively. The TEM and SEM were JEOL JEM-2010 (JAPAN) and FEI Quanta 400 (The Czech Republic), respectively.
3. Results
3.1. Synthesis of silver nanoparticles (AgNPs) from P. emblica fruit extracts
shows the AgNO3- solution after adding the fruit extract of P. emblica, during 0.5–24 h of incubation. The color and turbidity of sample containing AgNO3 changed increasingly from light brownish to brown with high turbidity. The sample had an observable initial turbidity after 6 h of incubation. The UV–visible absorption spectra of the AgNPs at different incubation times were recorded. This showed the successful synthesis of AgNPs by P. emblica fruit extract, and the synthesis was confirmed by UV-visible spectrophotometer. Formation of AgNPs was initiated at 0.5 h of incubation, which was also the beginning of observing a brown color. The maximum absorption peak was located at 430–434 nm which is in the proper theoretical range of wavelengths (200–800 nm) for AgNPs synthesis [Citation25].
3.2. Antibacterial effect dependence on incubation time and concentration of synthesized AgNPs or combination of V. diospyroides cotyledon extract (VCE) and AgNPs
To examine the antibacterial activity of the synthesized AgNPs, alone and in combination with VCE, antibacterial susceptibility tests were performed against gram positive and gram negative bacteria. As shown in and , AgNPs alone and proportions among P. emblica fruit extract, AgNO3- and AgNPs with DMSO showed highest antibacterial activity against the tested bacteria with clear zone sizes of 13.0, 10.0, 12.0 and 12.56 mm, respectively (). All strains of bacteria responded to AgNPs in dose-dependent manner (). When the incubation time with AgNPs increased, the treatment had decreasing antibacterial activity against all tested strains. For S. aureus and P. aeruginosa, antibacterial activity significantly decreased (p = 0.05). As the concentration of AgNPs was diluted, the size of inhibition zone decreased with all bacterial strains. As shown in , VCE plus AgNPs showed remarkable antibacterial activity against S. aureus and B. subtilis with larger clear zone sizes than that for AgNPs singly (). Effects of incubation time and concentration of AgNPs in the combination resembled the results obtained for AgNPs alone, but with larger inhibition zones (reaching 18 mm). On increasing AgNPs and decreasing VCE concentration, the combination gave reduced size inhibition zones for both S. aureus and B. subtilis. No activity was observed against the growth of E. coli and P. aeruginosa (data not shown). Therefore, VCE and AgNPs were more effective against gram-positive bacteria than against gram-negative bacteria.
Figure 2. Antibacterial activity of combination of VCE and AgNPs against (a) S. aureus, (b) B. subtilis.
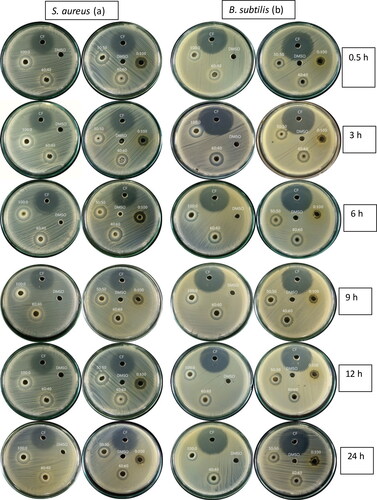
Table 1. Effect of synthesis incubation time and concentration of synthesized AgNPs on antibacterial activity.
Table 2. Effects of proportions of P. emblica fruit extract and DMSO, AgNO3- and DMSO, and AgNPs and DMSO, on inhibition of four bacteria.
Table 3. Effects of proportions of V. diospyroides cotyledon extract (VCE) and AgNPs incubated for 0.5–24 h on antibacterial activity against S. aureus and B. subtilis.
3.3. Minimum inhibitory concentrations (MICs) and fractional inhibitory concentration index (FICI)
AgNPs alone showed antibacterial ability against S. aureus and B. subtilis with MIC values of 11.83 and 0.74 µg/mL, respectively ( and ). The MIC (2000 and 500 µg/mL) of VCE indicated growth inhibition of S. aureus and B. subtilis, respectively. Interestingly, in combination the AgNPs and VCE had MIC for S. aureus and B. subtilis that revealed a significant decrease (fourfold) (from 2,000 to 500, 500 to 125, and 11.83 to 2.96 µg/mL) except for the MIC of AgNPs against B. subtilis being similar when used alone (MIC = 0.74 µg/mL) ( and ). The synergistic effects of AgNPs and VCE were assessed from the FICI shown in . Synergy existed for the AgNPs and VCE combinations against S. aureus, with FICI = 0.5, but not for B. subtilis (FICI =1.25 indicates indifference).
Figure 3. MIC determination of AgNPs (A and B), V. diospyroides cotyledon extract (C and D) and synergistic blend of both substances (E and F) against S. aureus (a) and B. subtilis (b) tested by broth microdilution and resazurin assay, with 2 replicate wells.
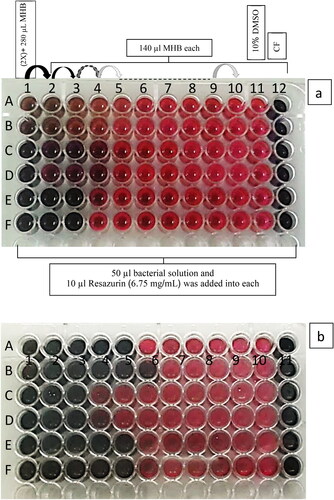
Table 4. Minimum inhibitory concentrations (MIC) and minimum bactericidal concentrations (MBC) of AgNPs and V. diospyroides cotyledon extract (VCE) when used singly or in combination against S. aureus and B. subtilis.
Table 5. FIC values of AgNPs and VCE, and FICI for the two-substance combinations.
3.4. AgNPs combined with VCE affected bacterial membrane and caused intracellular disruptions
Representative results of flow cytometric analysis for the synergistic antibacterial effects of AgNPs and VCE on S. aureus and B. subtilis strains are shown in . The responses of bacterial membrane and intracellular status to the synergy are shown separately for treated S. aureus and B. subtilis cells, exposed to various concentrations (0.5 × MIC, MIC and 2 × MIC). After 3–24 h treatments, the response patterns of tested bacteria were different. S. aureus incubated with 0.5 × MIC, MIC and 2 × MIC for 0, 3 and 12 h displayed continuously increased counts in dose-dependent manner relative to unincubated samples, increasing from 5.2% at 0 h in the 0.5 × MIC to 24.0 and 27.9% in the treated samples of MIC and 2 × MIC, respectively, and increasing by from 24.0 to 41.2 to 42.0% at 3 h; and from 30.37 to 54.9 to 59.8% at 12 h, respectively. Incubation for 6 and 24 h had positive and negative effects on cell responses, increasing from 17.3 to 28.5%, and decreasing from 64.4 to 62.4%. respectively.
Figure 4. Flow cytometry dot plots for S. aureus and B. subtilis treated with 0.5xMIC, MIC and 2xMIC or combination of AgNPs and VCE incubated for 0–24 h. *The regions divided by the lines were interpreted as: lower left for viable cells (FL2-negative and SSC-negative), lower right for membrane-damaged cells (FL2-positive and SSC-negative), upper left for injured cells (FL2-negative and SSC-positive), and upper right for dead cells (FL2-positive and SSC-positive). PI was used to evaluate bacterial membrane permeability. If loss or damage of membrane occurred, PI could penetrate to the cell and bind with nucleic acids (FL2-positive). In the injured cell with breakdown of intracellular components, loss of granularity of the cell or high complexity could increase the reflection of light (SSC-positive). Thus, the signals in the density plot are interpreted by each quarter.
When B. subtilis was incubated for 3, 6 and 24 h, the highest fraction of responding cells was achieved by treating with the MIC concentration (20.6, 30.6, and 31.7%, respectively). Dose-dependent response (17.9, 31.1 and 33.5%, respectively) was observed when B. subtilis was treated and incubated for 12 h. Time-dependent response was achieved when treating B. subtilis with the MIC concentration (15.3, 20.6, 30.6, 31.1 and 31.7). This increase was not higher than those obtained when treating S. aureus with the same concentration. Synergistic efficacy appeared to be a consequence of S. aureus and B. subtilis membrane damage (high FL2) and intracellular disruption (high SSC), which was associated with both AgNPs and VCE. These results were confirmed by SEM and TEM images. The SEM and TEM images of S. aureus and B. subtilis treated synergistically with AgNPs and VCE are shown in , and Citation5(b,d,f,h), respectively, with clear evidence of pores formed in the outer membrane, showing rather wrinkled shapes and connected to 60 nm size of AgNPs (), and thus confirming membrane damage and intracellular disruption with leakage, leading to cell death.
Figure 5. SEM and TEM images of S. aureus and B. subtilis treated with synergistic MIC of AgNPs and VCE: (a and c) SEM and TEM images of untreated S. aureus; (b and d) SEM and TEM images of treated S. aureus; (e and g) SEM and TEM images of untreated B. subtilis; and (f and h) SEM and TEM images of treated B. subtilis (scale bar is 1 µm).
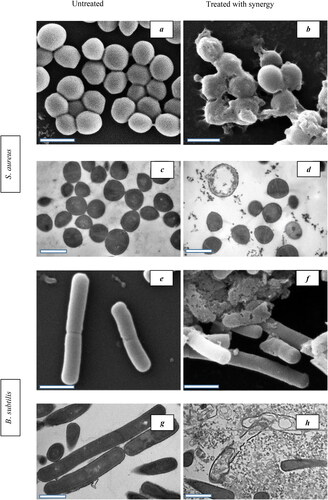
Figure 6. SEM images show the AgNPs effects after treating (a) B. subtilis, and (b) S. aureus cells and the red arrows point to locations of AgNPs adhesion with bacterial cells, whereas free AgNPs are indicated by yellow arrow. The calculated size of AgNPs was approximately 60 nm with spherical shape (scale bar is 1 µm).
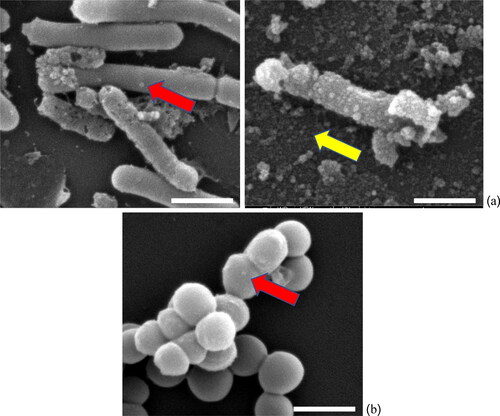
4. Discussion
In this study, we used P. emblica fruit extracts for synthesizing AgNPs with a general method, to avoid the risks of conventional synthesis using chemicals. We followed the Ramesh et al. [Citation21] method using AgNO3- to induce AgNP formation in P. emblica fruit extracts with incubation for 0.5–24 h. It is interesting to note that after 0.5 h of incubation, color of the solution appeared to be light brown and it continuously increased to dense brown with absorption at 432–434 nm wavelength. The color change might be due to phytochemicals in the plant [Citation26] whereas the wavelength indicates the complete formation of maximum yield of AgNPs in the size of 60 nm diameter [Citation25].
AgNPs can be synthesized by two types of methods, namely top down or bottom up. Antibacterial effects of AgNPs depend on various common factors such as the composition, size, shape, synthesis method, and material sources (plants or bacteria) [Citation17,Citation27–29]. Using plant extract or culture supernatant of bacteria to synthesize AgNPs are examples of the bottom-up approach [Citation30] completed after 48–96 h of incubation [Citation28,Citation29]. Thus, we confirmed and reasoned that when the antibacterial activity was investigated, the widest clear zone of inhibition found in the treatment with 0.5 h incubation for all bacteria, indicated efficient AgNPs that might have been complete early on during the incubation. From our results, P. emblica fruit extract is a most suitable resource for green AgNPs synthesis because it is eco-friendly, has high efficacy, and gives a rapid green AgNPs synthesis [Citation21].
We found that combinations of VCE and AgNPs showed strong synergistic antibacterial activity against the tested bacteria, with synergy established by comparing to the activities of VCE or AgNPs used singly. Previous studies have reported that AgNPs are effective against both gram-positive and gram-negative bacteria [Citation31–33]. On the other hand, our results showed that the AgNPs were more active against Gram-positive than Gram-negative bacteria. It can be seen that the concentration of AgNPs singly or in a combination affected antibacterial activity. Gram positive bacteria exhibited larger clear zones than the gram negative bacteria. AgNPs when used by themselves did not have any antibacterial activity against the gram negative bacteria, which indicates that the AgNPs in this study are especially appropriate for only Gram-positive bacteria. However, most previous studies have reported that Gram-negative bacteria are more susceptible to AgNPs [Citation34]. Antibacterial activity of the AgNPs might be affected after attachment of AgNPs on the bacteria, and subsequently be affected by the type of bacterial wall which is thicker for Gram negative than for Gram-positive strains. As another explanation, the AgNPs can penetrate into the cells and induce reactive oxygen species (ROS) and free radical generation [Citation35,Citation36] leading to damage to granularity, and consequently inhibited cell growth [Citation37].
In many studies on antibacterial activity, the efficacy results are not satisfactory, but when using advanced methods, the results are improved. Based on the synergistic model, both bacteria were killed in a shorter time at low concentrations of both VCE and AgNPs. Details of the interpretation of the broth microdilution plus Resazurin assay were based on the FICI as indicator. The FICI results showed that achieved bactericidal effect was synergistic against S. aureus, while an indifferent combination effect was found against B. subtilis. This is the first report that confirmed synergistic effect of AgNPs with a plant extract against a strain of gram positive bacteria. Antibacterial effects of VCE against S. aureus could be enhanced by AgNPs. In contrast, the combination of AgNPs and VCE led to decreased antibacterial effect of VCE against B. subtilis. The antibacterial activity of combined AgNPs and VCE might be due to phytochemicals in VCE (see ). VCE has terpenoids and anthraquinones that possess antibacterial activity against only gram positive bacteria [Citation20]. Unfortunately, AgNPs could effectively inhibit the activity of VCE since the activity of VCE was decreased. This issue may be due to the cell wall structure of gram-positive bacteria [Citation19] and some possible mechanisms are outer membrane warping, free translocation on membrane, penetration to interior, and membrane embedment [Citation38].
Cell envelope of gram positive bacteria includes peptidoglycan, which is a well-established target for antibiotics [Citation39]. B. subtilis is non-pathogenic in healthy individuals and the bacteria have even been reported as potential therapeutic agent, whereas the pathogen S. aureus is a known cause of human diseases [Citation40]. B. subtilis could inhibit the growth of S. aureus in natural competition. Therefore, our results could provide insights to deriving new bactericidal agents from medicinal plants that possesses safe and eco-friendly advantages with effects against only pathogenic gram positive S. aureus.
We have verified the synergy between AgNPs and VCE on the tested bacteria using flow cytometry and electron microscopy. We hypothesized that VCE could affect agent-induced membrane damage of bacteria. The bactericidal pathways of AgNPs and their challenges in the treatment of bacterial infections remain currently unclear [Citation41]. Katva et al. [Citation16] reported that the drug and AgNPs complexes release Ag+ at a higher rate. Therefore, the active compounds of VCE may result in conjugation of the compounds with Ag+. This will result in an effective concentration of the combination at a specific site and the loss of membrane integrity. As seen in Flow cytometric profiles and Electron microscopy images, the active compounds permeabilized bacterial membranes and might therefore provide the AgNPs access to internal target sites [Citation42]. Flow cytometric profiles and images from Electron microscopy showed that combination of VCE and AgNPs affected cell membrane integrity and intracellular components in both bacteria. While other studies have confirmed treatments of gram-positive bacteria with AgNPs, without involving cell wall disruption [Citation43], the actions of AgNPs plus plant extract have never before been explored. On the other hand, many recent studies have tried to explore the green synthesis of AgNPs using new effective plants or other organisms to reveal potential antimicrobial activity [Citation44–46]. Those AgNPs may have high potential for applications in the treatment of bacterial infections when combined with plant extracts.
In conclusion, AgNPs and VCE act as antibacterial agents when used singly or in combination. The bacterial response patterns had synergy characterized by flow cytometric analysis and Electron microscopy images. This study confirmed the effects of green-synthesized AgNPs on increasing antibacterial activity of VCE. The mechanisms of action of the VCE and AgNPs combination need more investigation. The enhancement of the antibacterial activity due to added AgNPs could promote the use of medicinal plant extracts that are efficient and safe, providing new possibilities in healthcare and veterinary treatments.
Declaration of interest statement
The authors have no conflicts of interest to declare.
Acknowledgements
We are grateful to Associate Professor Seppo J. Karrila for kindly editing this manuscript
Additional information
Funding
References
- Gonelimali FD, Lin J, Miao W, et al. Antimicrobial properties and mechanism of action of some plant extracts against food pathogens and spoilage microorganisms. Front Microbiol. 2018;9:1639. https://doi.org/10.3389/fmicb.2018.01639.
- McMurray RL, Ball M, Tunney MM, et al. Antibacterial activity of four plant extracts extracted from traditional Chinese medicinal plants against Listeria monocytogenes, Escherichia coli, and Salmonella enterica subsp. enterica serovar enteritidis. Microorganisms 2020;8(6):962. https://doi.org/10.3390/microorganisms8060962.
- Tura GT, Eshete WB, Tucho GT. Antibacterial efficacy of local plants and their contribution to public health in rural Ethiopia. Antimicrob Resist Infect Control 2017;6:76. https://doi.org/10.1186/s13756-017-0236-6.
- Hemeg HA, Moussa IM, Ibrahim S, et al. Antimicrobial effect of different herbal plant extracts against different microbial population. Saudi J Biol Sci. 2020;27(12):3221–3227. https://doi.org/10.1016/j.sjbs.2020.08.015
- Gupta PD, Birdi TJ. Development of botanicals to combat antibiotic resistance. J Ayurveda Integr Med. 2017;8(4):266–275. https://doi.org/10.1016/j.jaim.2017.05.004.
- Wang L, Hu C, Shao L. The antimicrobial activity of nanoparticles: present situation and prospects for the future. Int J Nanomed. 2017;12:1227–1249. https://doi.org/10.2147/IJN.S121956.
- Asare N, Instanes C, Sandberg WJ, et al. Cytotoxic and genotoxic effects of silver nanoparticles in testicular cells. Toxicology 2012;291(1-3):65–72. https://doi.org/10.1016/j.tox.2011.10.022.
- Amaro F, Morón Á, Díaz S, et al. Metallic nanoparticles—friends or foes in the battle against antibiotic-resistant bacteria? Microorganisms 2021;9(2):364. https://doi.org/10.3390/microorganisms9020364.
- Zhang D, Ma XL, Gu Y, et al. Green synthesis of metallic nanoparticles and their potential applications to treat cancer. Front Chem. 2020;8:799. https://doi.org/10.3389/fchem.2020.00799.
- Qing Y, Cheng L, Li R, et al. Potential antibacterial mechanism of silver nanoparticles and the optimization of orthopedic implants by advanced modification technologies. Int J Nanomed. 2018;13:3311–3327. https://doi.org/10.2147/IJN.S165125.
- Masum M, Siddiqa MM, Ali KA, et al. Biogenic synthesis of silver nanoparticles using Phyllanthus emblica fruit extract and its inhibitory action against the pathogen acidovorax oryzae strain rs-2 of rice bacterial brown stripe. Front Microbiol. 2019;10:820. https://doi.org/10.3389/fmicb.2019.00820.
- Nguyen DH, Lee JS, Park KD, et al. Green silver nanoparticles formed by Phyllanthus urinaria, Pouzolzia zeylanica, and Scoparia dulcis leaf extracts and the antifungal activity. Nanomaterials (Basel, Switzerland) 2020;10(3):542. https://doi.org/10.3390/nano10030542.
- Meena RK, Meena R, Arya DK, et al. Synthesis of silver nanoparticles by Phyllanthus emblica plant extract and their antibacterial activity. Mat Sci Res India 2020;17(2):136–145. http://dx.doi.org/10.13005/msri/170206.
- Aabed K, Mohammed AE. Synergistic and antagonistic effects of biogenic silver nanoparticles in combination with antibiotics against some pathogenic microbes. Front Bioeng Biotechnol. 2021;9:652362. https://doi.org/10.3389/fbioe.2021.652362.
- Otieno JN, Hosea KM, Lyaruu HV, et al. Multi-plant or single-plant extracts, which is the most effective for local healing in tanzania? Afr J Tradit Complement Altern Med. 2008;5:165–172. https://doi.org/10.4314/ajtcam.v5i2.31269.
- Katva S, Das S, Moti HS, et al. Antibacterial synergy of silver nanoparticles with gentamicin and chloramphenicol against Enterococcus faecalis. Phcog Mag. 2017;13:S828–S33. https://doi.org/10.4103/pm.pm_120_17.
- Chen YH, Wang WH, Lin SH, et al. Synergistic antibacterial effect of casein-AgNPs combined with tigecycline against Acinetobacter baumannii. Polymers 2021;13(9):1529. https://doi.org/10.3390/polym13091529.
- Essawy E, Abdelfattah MS, El-Matbouli M, et al. Synergistic effect of biosynthesized silver nanoparticles and natural phenolic compounds against drug-resistant fish pathogens and their cytotoxicity: an in vitro study. Mar Drugs 2021;19(1):22. https://doi.org/10.3390/md19010022.
- Keawchai K, Chumkaew P, Permpoonpattana P, et al. Synergistic effect of Hydnophytum formicarum tuber and vatica diospyroides symington cotyledon extracts with ampicillin on pathogenic bacteria. J Appl Biol Biotechnol. 2022;10:6–11. https://doi.org/10.7324/JABB.2022.100202.
- Musimun C, Chuysongmuang M, Permpoonpattana P, et al. FACS analysis of bacterial responses to extracts of vatica diospyroides fruit show dose and time dependent induction patterns. Walailak J Sci Technol. 2017;14:883–891. https://wjst.wu.ac.th/index.php/wjst/article/view/2376.
- Ramesh PS, Kokila T, Geetha D. Plant mediated green synthesis and antibacterial activity of silver nanoparticles using emblica officinalis fruit extract. Spectrochim Acta A Mol Biomol Spectrosc. 2015;142:339–343. https://doi.org/10.1016/j.saa.2015.01.062.
- Mohd Yusof H, Abdul Rahman N, Mohamad R, et al. Microbial mediated synthesis of silver nanoparticles by Lactobacillus plantarum TA4 and its antibacterial and antioxidant activity. Appl Sci. 2020;10(19):6973. https://doi.org/10.3390/app10196973
- Sarker SD, Nahar L, Kumarasamy Y. Microtitre plate-based antibacterial assay incorporating resazurin as an indicator of cell growth, and its application in the in vitro antibacterial screening of phytochemicals. Methods 2007;42(4):321–324. https://doi.org/10.1016/j.ymeth.2007.01.006.
- Mohammadi M, Khayat H, Sayehmiri K, et al. Synergistic effect of colistin and rifampin against multidrug resistant Acinetobacter baumannii: A systematic review and Meta-analysis. Open Microbiol J. 2017;11:63–71. https://doi.org/10.2174/1874285801711010063.
- Paramelle D, Sadovoy A, Gorelik S, et al. A rapid method to estimate the concentration of citrate capped silver nanoparticles from UV-visible light spectra. Analyst 2014;139(19):4855–4861. https://doi.org/10.1039/C4AN00978A
- Urnukhsaikhan E, Bold BE, Gunbileg A, et al. Antibacterial activity and characteristics of silver nanoparticles biosynthesized from carduus crispus. Sci Rep. 2021;11(1):21047. https://doi.org/10.1038/s41598-021-00520-2.
- Zhang XF, Liu ZG, Shen W, et al. Silver nanoparticles: synthesis, characterization, properties, applications, and therapeutic approaches. IJMS 2016;17(9):1534. https://doi.org/10.3390/ijms17091534.
- Peiris MK, Gunasekara CP, Jayaweera PM, et al. Biosynthesized silver nanoparticles: are they effective antimicrobials? Mem Inst Oswaldo Cruz. 2017;112(8):537–543. https://doi.org/10.1590/0074-02760170023.
- Kumari SC, Padma PN. A facile, green approach for enhanced synthesis of silver nanoparticles by Weissella confusa produced dextran. Plant Arch. 2020;20:1598–1602.
- Deepak V, Umamaheshwaran PS, Guhan K, et al. Synthesis of gold and silver nanoparticles using purified URAK. Colloids Surf B Biointerfaces 2011;86(2):353–358. https://doi.org/10.1016/j.colsurfb.2011.04.019.
- Bruna T, Maldonado-Bravo F, Jara P, et al. Silver nanoparticles and their antibacterial applications. IJMS 2021;22(13):7202. https://doi.org/10.3390/ijms22137202.
- Fayaz M, Balaji K, Girilal M, et al. Biogenic synthesis of silver nanoparticles and their synergistic effect with antibiotics: a study against gram-positive and gram-negative bacteria. Nanomedicine 2010;6(1):103–109. https://doi.org/10.1016/j.nano.2009.04.006.
- Morones JR, Elechiguerra JL, Camacho A, et al. The bactericidal effect of silver nanoparticles. Nanotechnology 2005;16(10):2346–2353. https://doi.org/10.1088/0957-4484/16/10/059.
- Yin IX, Zhang J, Zhao IS, et al. The antibacterial mechanism of silver nanoparticles and its application in dentistry. Int J Nanomed. 2020;15:2555–2562. https://doi.org/10.2147/IJN.S246764.
- Dakal TC, Kumar A, Majumdar RS, et al. Mechanistic basis of antimicrobial actions of silver nanoparticles. Front Microbiol. 2016;7:1831. https://doi.org/10.3389/fmicb.2016.01831.
- Durán N, Durán M, de Jesus MB, et al. Silver nanoparticles: a new view on mechanistic aspects on antimicrobial activity. Nanomedicine 2016;12(3):789–799. https://doi.org/10.1016/j.nano.2015.11.016.
- Sondi I, Salopek-Sondi B. Silver nanoparticles as antimicrobial agent: a case study on E. coli as a model for gram-negative bacteria. J Colloid Interface Sci. 2004;275(1):177–182. https://doi.org/10.1016/j.jcis.2004.02.012.
- Lin L, Chi J, Yan Y, et al. Membrane-disruptive peptides/peptidomimetics-based therapeutics: Promising systems to combat bacteria and cancer in the drug-resistant era. Acta Pharm Sin B. 2021;11(9):2609–2644. https://doi.org/10.1016/j.apsb.2021.07.014.
- Rajagopal M, Walker S. Envelope structures of gram-positive bacteria. Curr Top Microbiol Immunol. 2017;404:1–44. https://doi.org/10.1007/82_2015_5021.
- Gonzalez DJ, Haste NM, Hollands A, et al. Microbial competition between Bacillus subtilis and Staphylococcus aureus monitored by imaging mass spectrometry. Microbiology (Reading) 2011;157(Pt 9):2485–2492. https://doi.org/10.1099/mic.0.048736-0.
- López-Heras M, Theodorou IG, Leo BF, et al. Towards understanding the antibacterial activity of Ag nanoparticles: electron microscopy in the analysis of the materials-biology interface in the lung. Environ Sci Nano. 2015;2(4):312–326. https://doi.org/10.1039/C5EN00051C.
- Ruden S, Hilpert K, Berditsch M, et al. Synergistic interaction between silver nanoparticles and membrane-permeabilizing antimicrobial peptides. Antimicrob Agents Chemother. 2009;53(8):3538–3540. https://doi.org/10.1128/AAC.01106-08.
- Vazquez-Muñoz R, Meza-Villezcas A, Fournier PGJ, et al. Enhancement of antibiotics antimicrobial activity due to the silver nanoparticles impact on the cell membrane. PLoS One 2019;14(11):e0224904. https://doi.org/10.1371/journal.pone.0224904.
- Skóra B, Krajewska U, Nowak A, et al. Noncytotoxic silver nanoparticles as a new antimicrobial strategy. Sci Rep. 2021;11(1):13451. https://doi.org/10.1038/s41598-021-92812-w.
- Liu X, Chen JL, Yang WY, et al. Biosynthesis of silver nanoparticles with antimicrobial and anticancer properties using two novel yeasts. Sci Rep. 2021;11(1):15795. https://doi.org/10.1038/s41598-021-95262-6.
- Hemlata, Meena PR, Singh AP, Tejavath KK. Biosynthesis of silver nanoparticles using Cucumis prophetarum aqueous leaf extract and their antibacterial and antiproliferative activity against cancer cell lines. ACS Omega 2020;5(10):5520–5528. https://doi.org/10.1021/acsomega.0c00155.