Abstract
To establish a gold/silica hybrid, nanomaterials (Au/SiO2) were incorporated into a gelatin methacrylate/chitosan matrix. By using FESEM, compressive strength testing, and conductivity/resistance measurements on gelatin (G)/chitosan (C), G/C-Au@SiO2 hydrogels developed. Biocompatibility investigations on osteoblasts MG-63 cells were carried out to determine whether the cell was compatible with the conductive hydrogel as it had been created. The results indicated that HNPs had improved compressive strength and conductivity without losing the favourable features such as biodegradable nature and porous shape of G/C hydrogel. The mechanical properties and Elastic modulus of composites hydrogels were enhanced twofold when hybrid nanomaterials were added to the mixture. The cyclic compressive analysis shows that pure G/C hydrogels lost their mechanical stability within the first few cycles, but G/C-Au@SiO2 hydrogels lasted for up to fifty cycles. It was demonstrated that osteoblast proliferation and adhesion were increased on the hydrogel in the CCK-8 experiment. Further, the cell survival of the hydrogels with G/C-Au@SiO2 conductivity was enhanced by 15% compared to that of pure G/C hydrogels. The morphological features of the MG-63 cells experiments were performed by using a Fluorescein diacetate hydrolysis (FDA) staining assay. This work offers a unique method for enhancing mechanical integrity and electrical properties in gelatin-based G/C hydrogels by adding bifunctional hybrid nanomaterials (HNPs) for bone fracture tissue engineering applications.
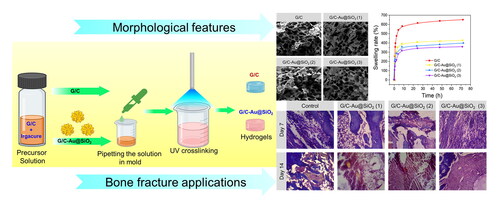
1. Introduction
Various diseases, such as trauma, arthritis, and sports-related injuries, can lead to cartilage and subchondral bone loss. 60% of individuals with knee arthroscopy have cartilage loss, with 15% or so showing clinical symptoms [Citation1–3]. Because injured cartilage lacks vascularization, innervation, lymphatic networks, and stem cells, its ability to self-heal is severely restricted. Because of donor-site morbidity and infection concerns and a high nonunion rate with host tissues, frequently utilized methods for bone tissue repair, such as autografting and allografting, are limited [Citation4–6]. Bone abnormalities are among the most common causes of morbidity and disability in older people. Healing of injured cartilage and bone tissue is still a challenge [Citation7–9]. Therefore, discovering a way to repair injured cartilage permanently and bone tissue is of great therapeutic relevance to patients with cartilage lesions and bone abnormalities [Citation10–12].
Hydrogels imitate the extracellular matrix (ECM) to offer mechanical support for cells in created tissues [Citation13–16]. Due to their remarkable characteristics, such as tunable physicochemical characteristics, biocompatibility, and relatively simple microfabrication, can also be used for other biological applications, such as gene or drug carriers, bioadhesives, and injectable biomaterials in cell therapy [Citation17–20]. It is a hydrophilic polymeric substance with a high-water content used to construct hydrogels. Hydrogels can be classed as natural, or synthetic based on their origin. They can influence cell-signalling pathways because they are biodegradable (e.g. gelatin and collagen) and include chitosan, elastin, hyaluronic acid [HA], and alginate [Citation21]. But they have limited mechanical strength, can trigger an immunological response, and have uncontrollable structure and breakdown dynamics [Citation22,Citation23]. As an alternative, synthetic hydrogels' structure and degradation kinetics (e.g. polyethene glycol [PEG] and polyvinyl alcohol) can be regulated [Citation24]. Because they lack physiologically active groups, manufactured hydrogels have a disadvantage compared to natural ones. In biomedical applications, mixing natural and synthetic hydrogels makes sense because of the distinct characteristics of each hydrogel type [Citation25–27].
The incorporation of metal-based gold nanostructures, on the other hand, is sufficient to increase the hydrogel's conductivity [Citation28]. Moreover, if the hydrogel's mechanical strength is improved, it may be used as a scaffold for biomedical applications and tissue engineering [Citation29–31]. In hydrogel systems, mechanical characteristics depend on the precursors' concentration and molecular weight and the crosslinks' density chemistry. While increasing crosslinking time makes the hydrogel stiffer, it limits the pharmacological functions of the matrix, for example, restricted migration and cell proliferation, or needs an expensive or lengthy pre-culture phase to get the scaffolds ready for use [Citation32]. To create bioactive hydrogels, it is essential to find ways to increase the mechanical characteristics of the gel without compromising its positive features. Bioactive silica nanostructures in this context might be a viable method to use. Hydrogel composites containing silica nanostructures can strongly bond with polymer matrix, strengthening the mechanical properties of composite hydrogels [Citation33]. For these reasons, silica nanostructures have been extensively studied for various biological purposes. According to a study by Yang et al., silica nanomaterials were employed as fillers to increase the viscoelastic and elastic modulus attributes of nanocomposite poly(acrylic acid) hydrogel matrix [Citation34]. For tissue engineering applications, the researchers also enhanced the compressive strength of composite hydrogels by forming a penetrating structure among the chitosan and poly(acrylic acid) using silica nanomaterials as a filler [Citation35]. For example, Zhu et al. fabricated mesoporous silica nanomaterials embedded in the chitosan hydrogels matrix and showed that the silica mesoporous nanomaterials improved the elastic modulus of the hydrogels matrix [Citation36].
Due to our hypotheses, we developed new nanomaterials that would simultaneously increase hydrogel's electrical and mechanical characteristics without sacrificing their intrinsic specialities for tissue engineering applications [Citation37–42]. By coating SiO2 NMs with Au nanomaterials, we have combined the advantageous features of both components. The next step included dispersing the Au@SiO2 nanomaterials, which had been produced uniformly into the G/C matrix before obtaining UV crosslinked composite hydrogels on the shape, physical and chemical properties, and biological characteristics of the hydrogels [Citation43–46]. According to work, G/C hydrogel composite containing hybrid nanomaterials had increased biocompatibility, electrical conductivity, and compressive strength without losing the favourable features of G/C hydrogel such as porous morphology and biocompatibility. The first time we've observed dual-functional nanostructures in hydrogels to increase their physical and chemical characteristics. A viable method for creating new composite scaffolds for biomedical applications would be to reinforce hydrogels with HNPs with dual functions.
2. Experimental section
2.1. Fabrication of gelatin methacrylate/chitosan (G/C)
As described in earlier studies, a G/C macromer was constructed according to a previous report [Citation35]. In brief, a 10 wt% gelatin solution was established in phosphate-buffered saline at 50 °C with continuous stirring. We added dropwise methacrylic anhydride and chitosan (0.8 mL/g of gelatin/chitosan) to stirred for 4 h the gelatin solution in the dark condition after wholly dissolved in the solution. To terminate the substitution process, a dialysis membrane was used to eradicate other byproducts and unreacted salts, including unreacted methacrylic acid, chitosan and methacrylic anhydride. During the dialysis, water was changed thrice and then air-dried and kept at −20 °C for later usage.
2.2. Fabrication of HNPs
Using an accessible electrostatic attraction between negatively charged SiO2 NMs and positively charged AuNMs, the hybrid (Au/SiO2) HNPs were fabricated in the previous work [Citation47]. To fabricate the SiO2 NMs, the cysteamine-modified method was used to prepare the Au nanomaterials. The accompanying information describes a precise technique for producing SiO2 NMs and AuNMs. With continuous stirring, 10 mL of AuNMs solution was added dropwise to a 20 mL SiO2 NMs solution synthesized, and the reaction mixture was agitated for 3 h before being analyzed. Before obtaining Au@SiO2 HNPs, the finishing reaction homogenate was stirred at 3000 rpm for 30 min and then re-dispersed using DD water.
2.3. Fabrication of hydrogels
shows a schematic of the preparation process for several hydrogel systems. A decisive concentration of 0.1% (w/v) of freeze-dried G/C (5 wt%) was added to DPBS with a photoinitiator. To generate a solid crosslinked G/C hydrogel, the G/C solution precursor was dissolved into a mould and subjected to UV radiation (360–480 nm; 6.9 mW/cm2) for 1 min. Composite hydrogels were prepared by mixing Au@SiO2 HNPs at 0.025, 0.050, and 0.1 mg/mL with G/C precursor solution and sonicating for 15 min to ensure homogenous dispersion of HNPs in the G/C composite. The mixed solutions were put into a mould and subjected to UV light. To remove any uncross-linked monomers and an unreacted photoinitiator, all fabricated hydrogels were submerged in DI water to wash them away. Hydrogels with Au@SiO2 HNP concentrations of 0.25, 0.50, and 0.1 mg/mL were labelled as G/C-Au@SiO2 (1), G/C-Au@SiO2 (2), and G/C-Au@SiO2 (3), respectively.
2.4. Characterization of hydrogels
Field-emission scanning electron microscopy (SEM, Bruker Gemini 500) was applied to characterize the as-prepared products' freeze-dried hydrogels' surface morphology and structure. To achieve this result, freeze-dried hydrogels (already known weight) were submerged at 37 °C in PBS (pH 7.4) to achieve this result. When the hydrogels were wet, they were removed from the PBS and reweighed at preset time intervals (20, 40, 60 min, 2, 4, 8, 24, 48, and 72 h) to measure their swelling weight. To determine the swelling ratio, we have followed the previously reported [Citation48–50].
The degradation profile of hydrogels was also examined by the previously reported [Citation51]. They were then weighed and wholly soaked in PBS (pH 7.4). All the samples were incubated at 37 °C for 24 h, and they were vigorously agitated at 100 rpm/min on a horizontal shaker. The PBS was updated every week. To ensure that the samples were completely dried, they were taken out at different time duration (3, 7, 14, 21, and 28 days), and dried samples were then weighed.
2.5. Examination of mechanical features
As-fabricated hydrogels were subjected to compressive testing to determine their mechanical characteristics [Citation52]. A sample was crushed until broken in the first mode; a sample was cyclically compressed in the second mode. The stress-strain curve had to be plotted to determine the compressive strength and Young's modulus from the first test. It was also shown that the hydrogel's durability could be resolved in a second test. Hydrogel discs with a diameter of 8 mm and a thickness of 5 mm (n = 3 from each group) were fabricated from the handmade mould for both experiments. The hydrogel was flowed into a handmade mould with circular pits of 8 mm diameter and immediately exposed to UV light for photocrosslinking. The photo crosslinked hydrogel was removed from the mould and submerged in PBS for 12 h. The Young's modulus and compressive strength of swollen equilibrium hydrogels were determined using a universal testing machine (UTM, MTDI INC. Korea) with a 100 N KAF-TC load sensor and a strain rate of 5.0 mm/s under ambient conditions. The cyclic compression test was used for the durability research. A cycle compression test was performed with a 60% load and 50 cycles. When the hydrogels were squeezed in the cyclic compressive test, the water inside the hydrogel flew out and was reabsorbed when the stress was removed.
2.6. Measurement of conductivity/resistivity
Structural materials with conductive characteristics may have a vital role in tissue electroactive remodelling [Citation53–55]. This was done by using a 3-electrode setup with an electrochemical workstation at ambient conditions. The conductivity of the composite hydrogels was evaluated using a three-electrode method. Lastly, Hg/Hg2Cl2 (calomel electrode) and Pt wire (counter electrode and reference electrode). However, a glassy-carbon electrode covered with Au@SiO2 HNPs has been employed to test Au@SiO2 HNPs conductivities. The hydrogel-coated Ti (working electrode) test the conductivity of the composite hydrogels. As well as measuring the charge transfer resistance, ZIVE ZMANTM 2.3 was used to perform impedance spectroscopy analysis and presentation.
2.7. Hydrogel's biocompatibility assay
Cells were then sterilized in 75% ethanol, rinsed with PBS, and shaped with discs 8 mm in diameter (thickness = 1.5 mm) before being placed into 94-well plates. We seeded MG-63 cells (1 × 103 cells/well) to the matrix and incubated them with 5% CO2 at 37 °C. The medium was replaced every two days during incubation. Cell viability was assessed using the manufacturer's procedure for the Cell-Counting Kit-8 (CCK-8) [Citation56–60]. Each cell planted at the well plate was incubated for 2 h at 37 °C with CCK-8 solutions (10 v/v %) applied to each well. Absorbance was determined at 450 nm (Tecan, Austria). An optical density standard calibration curve calculated the acquired optical density's relative cell viability (%). All the experiments were repeated three times.
2.8. Fluorescein diacetate hydrolysis (FDA) staining
To determine the cell viability of MG-63 in leach liquid, FDA staining was performed. At a cell density of 1 × 104 cells/well, the cell suspension was seeded in the leaching liquid of G/C-Au@SiO2. Three, five, and seven days later, the samples with cells were incubated at 37 °C for 24 h. The medium was withdrawn at a predetermined period, and the plates were washed twice with PBS. FDA containing 100 mL of FDA (100 µg/mL) was used to stain scaffolds and scaffolds/MPs for 10 min. During the whole method, no light was used. Once again, PBS was used to rinse the plates three times. Finally, the images were taken using an inverted microscope [Citation61–63].
2.9. In vivo fracture model
This experiment employed Kunming mice (males) weighing six weeks and 100 g. Each mouse was injected with ketamine hydrochloride. A complete fracture (2.5 cm2) was anaesthetized on the day of the injury. The top section of the fur of the mice was discarded, and the skin was cleaned hygienically. In these experiments, a complete excision fracture model was developed to determine the fracture effects of G/C-Au@SiO2 (1–3). On the 14th day after the fracture, the animals were sacrificed using anaesthesia, and the fracture was examined. The whole fracture tissue and neighbouring muscle were collected on days 7 and 14, sliced, soaked in 4% paraformaldehyde, embedded in paraffin, and stained with hematoxylin and eosin. To prevent any external fracture injuries, all the mice were housed separately.
2.10. Statistical analysis
The data presented here were mean ± standard deviation (SD). The significance of the difference was tested by Student’s t test, which was significant when p < 0.05 and very significant when p < 0.01.
3. Results and discussion
3.1. Fabrication and characterization of HNPs
We fabricated AuNMs and SiO2 NMs in two different batches to treat the bone fracture. Effortless electrostatic interactions among positive surface charges on AuNMs and negative surface charges on SiO2 NMs were used to produce the Au@SiO2 HNPs. The TEM imaging, the surface morphology of HNPs was examined. As seen in , the surface of the SiO2 nanomaterials was elegantly adorned with AuNMs. UV–Vis–NIR spectroscopy () of AuNMs and SiO2 NMs verified the composite Au@SiO2 production. AuNMs displayed a significant absorption peak at max −525 nm in the UV–VIS–NIR spectrum, but SiO2 NMs did not show any considerable absorption peaks in the visible region. The Au@SiO2 NHPs displayed an absorption peak around a similar area (550–700 nm) with a broad type, indicating the effective connection of AuNMs on the shell of the SiO2 NMs.
3.2. Physico-chemical characterization of Au@SiO2 HNPs
In this study, Au@SiO2 HNPs will be incorporated into a G/C hydrogel to increase the physicochemical characteristics for biological applications. Hydrogels of two distinct kinds have been produced. As a result, two different formulations were created: one with pure gold and copper and another with gold and copper with varying amounts of HNPs. It was shown in that the hydrogels had been digitally and FESEM imaged. Pure G/C hydrogel was photographed () in a clear hue, whereas G/C-Au@SiO2 HNPs were photographed in a pinkish tint. As shown in , the pinkish hue of composite hydrogels was comparable to the HNPs. FESEM images of hydrogels are shown in . Every single hydrogel was microporous, with thin walls and empty structures, as shown in FESEM pictures. Composite hydrogels have a smaller pore size than pure G/C hydrogels. In composite hydrogels, the smaller pores are due to the strong connection between the hydrogel matrix and HNPs. As a result of these findings, the Au@SiO2 HNPs were uniformly distributed in the G/C matrix and exhibited similar porosity morphology to pure G/C.
Figure 3. FESEM images of pure G/C and G/C-Au@SiO2 HNPs containing various ratios (scale bar 20 µm).
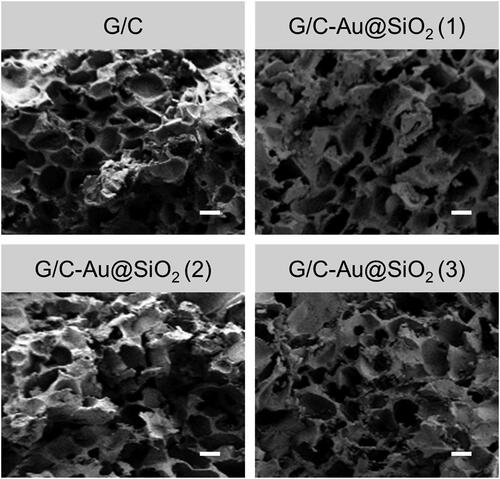
Hydrogels' capacity to swell directly impacts gas exchanges, bodily fluid absorption, nutrition transfer, cell encapsulation, and cell ingrowth inside the constructions. As seen in , various hydrogels have distinct swelling properties. In contrast, G/C-Au@SiO2 composite hydrogels demonstrated a substantially lower swelling ratio (300–400%) than G/C alone. It took 8 h for pure G/C to reach its equilibrium swelling point, whereas G/C-Au@SiO2 hydrogels took 4 h to get their equilibrium swelling. Incorporating HNPs into G/C hydrogels resulted in a reduction in swelling. The low swelling in the hydrogel’s matrix may be ascribed to the smaller porous size in hydrogels due to the strong bonding among the G/C and HNPs and the decreased bonding positions accessible for water molecules. As Navaei and colleagues observed, the hydrogel system's reduced swelling rate and narrower pore size were connected.
Figure 4. A) Swelling ratio of pure G/C and G/C-Au@SiO2 HNPs containing various ratios incubated in PBS buffer for different time intervals. B) Degradation of pure G/C and G/C-Au@SiO2 HNPs containing various ratios.
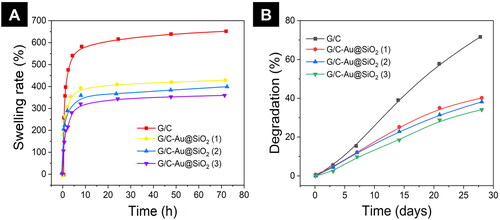
shows the results of a four-week deterioration test in PBS at 37 °C. Compared to pure G/C, composite hydrogels deteriorated at a similar pace in the first week. Increasing the incubation time led to G/C-Au@SiO2 (1–3) degradation. A substantial change in degradations was noticed from the 2nd week onwards, but it was more evident in the 4th week. This may be recognized as potential interactions between HNPs and G/C composites. Using HNPs, the degradation of G/C hydrogel was regulated. According to the experiments, hydrogels' swelling and degrading characteristics were interdependent. Therefore, the matrix with a high swelling ratio will interact with more water molecules, leading to rapid deterioration.
In contrast, the matrix with a low swelling ratio would exhibit a slower degradation. Consequently, both results suggested that Au@SiO2 HNPs regulated the strong swelling propensity. The quick breakdown ratio of G/C hydrogels with controlled degradation was seen as essential for improving the development of an ECM matrix, proliferation, and cell spreading.
3.3. G/C-Au@SiO2 hydrogels compressive strength
Biomedical applications advantage from the scaffolds' high load-bearing capability. Therefore, the compressive strength of pure G/C hydrogels, as well as G/C-Au@SiO2 hydrogels, was determined (). After 65–75% of strain, all the hydrogels ruptured (). It is worth noting that compressive strength or stress-bearing ability has been enhanced in the following order: (1) > (2) > (3) > G/C-Au@SiO2 (3). G/C-Au@SiO2 composite hydrogels had considerably higher compressive strength and Young's modulus values than pure G/C, as shown in . It was also found that hydrogels made of pure G/C lost their mechanical stability within the first few cycles, but those made of G/C-Au@SiO2 HNPs lasted up to fifty cycles (). Many experiments have shown that composite hydrogels with HNPs have a higher mechanical strength. This suggests a synergetic impact of SiO2 NMs and Au HNPs on HNPs mechanical characteristics.
3.4. G/C-Au@SiO2 hydrogels conductivity/resistivity
Electrical impedance spectroscopy was used to assess the conductivity of Au@SiO2 HNPs and G/C-Au@SiO2 HNPs containing various amounts of HNPs (). An inverse relationship exists between charge transfer resistance (Rct) and conductivity in the impedance spectrum obtained from Nyquist plots () in electrochemical impedance analysis (EIA). Au@SiO2 HNP EIS spectra () revealed a tiny semicircle in the high-frequency area, indicating a reduced Rct value, whereas the G/C-Au@SiO2 HNPs by EIS spectrum (). This value was 1.2k for Au@SiO2 HNPs, while the values for G/C-Au@SiO2 (1), (2), and (3) were 4.8, 4.7, and 4.6 K, respectively (). A lower Rct value for Au@SiO2 HNPs, on the other hand, implies a higher conductivity than composite hydrogels. According to several research investigations, an excellent conductive platform given by conductive hydrogels improves cell-to-cell and cell-to-hydrogel interactions. They also noted an increase in bone cell adhesion, proliferation, and differentiation when Au HNPs were incorporated into a decellularized matrix and an increase in connexin 43 electrical connection proteins and elongated, aligned shape.
3.5. Assessment of biocompatibility and proliferation
CCK-8 evaluation was used to determine the biocompatibility of pure G/C and G/C-Au/SiO2-conductive hydrogels [Citation64–66]. Early cell adhesion (3 and 6 h) was also an important marker for differentiation, leading to increased proliferation and cell-hydrogel interaction. CCK-8 results are displayed in . Because hybrid Au@SiO2 HNPs were used in G/C hydrogel, the CCK-8 assay showed no detrimental impacts on MG-63 osteoblasts' activities. Interestingly, after 3 and 6 h of seeding, the conductive hydrogels showed a substantial increase in cell density on the third day and day seven. It was also revealed that increasing the concentration of HNPs from 0.025% to 0.1% resulted in a higher cell density in MG-63 cells cultured on conductive hydrogels. This demonstrated that incorporating Au@SiO2 HNPs (0.1 mg/mL) into G/C hydrogels improved biocompatibility.
3.6. FDA staining analysis
MG 63 cells have grown for 3, 6 h, 3 days and 7 days; FDA staining was used to assess cell proliferation and activity in the leach liquor of G/C-Au@SiO2 (1–3). FDA staining can reveal MG 63 cellular morphology and influence the cell's structure (). FDA was the sole staining agent used on viable cells, which showed a brilliant green complete structure. When tested by the FDA, virtually all MG 63 cells were glowing green, and the cell nucleus was still intact. There were no adverse effects from the G/C-Au@SiO2 (1–3). There were also substantial differences between G/C-Au@SiO2 (1–3) compared to untreated cells, which indicated the matrix shows strong biocompatibility in the MG63 cells ().
Figure 8. G/C-Au@SiO2 HNPs performed fluorescein diacetate hydrolysis (FDA) staining with various compositions using MG 63 bone cells (scale bar 100 µm).
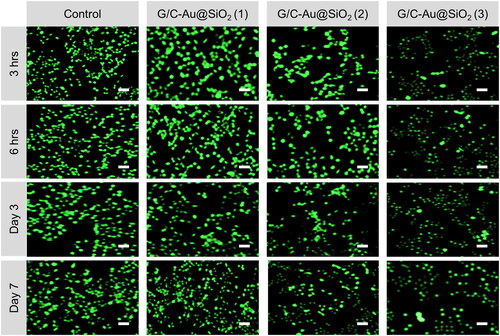
Figure 9. A) Fluorescein diacetate hydrolysis (FDA) staining by G/C-Au@SiO2 HNPs with various compositions using MG 63 bone cells. B) Histological assessment of the anti-inflammatory effects of G/C-Au@SiO2 HNPs with various composition inflammation on days 7 and 14 (scale bar 100 µm). C) The degree of inflammation score was assessed on a 0–5.
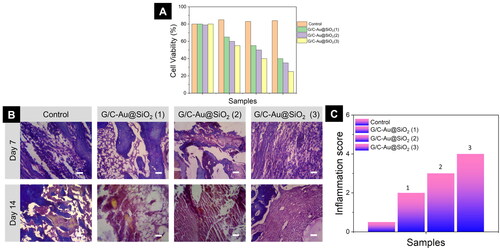
3.7. Assessment of animal fracture model
In an in vivo investigation employing Kunming mice, the G/C-Au@SiO2 (1–3) hydrogels were used at increasing doses and determined by histopathological analyses. The results demonstrated that both G/C-Au@SiO2 (1–3) hydrogels improved fracture healing ability. Injuries of the mice for healing were examined once every seven days. In the G/C-Au@SiO2 (1–3) bandage-treated group, a substantial reduction in the fracture region was noted, and on day 7, the fracture was complete, culminating in the recovery of the tissue. The fracture model treated with G/C-Au@SiO2 (1–3) bandages was better than those treated with as-prepared G/C-Au@SiO2 (1–3) bandages. These findings showed that a significant improvement in the fracture healing ability was observed using Au@SiO2. Fracture closure using the G/C-Au@SiO2 (1–3) dressing was higher than the Au@SiO2 (1–3) bandage. According to our findings, the nanocomposites exhibited more potential fracture healing effects than the control.
3.8. Histological evaluation
A histological study is a powerful tool for observing an improvement in fracture healing and tissue repair [Citation67]. Histological observations using hematoxylin and eosin (H&E) staining focused on the cure of the fracture mice owing to the effects of G/C-Au@SiO2 (1–3) (control) and G/C-Au@SiO2 (1–3) (). Compared to bare fracture, on days 11 and 14, an effective cure was demonstrated using G/C-Au@SiO2 (1–3) and G/C-Au@SiO2 (1–3). Compact keratinocytes in the epidermolytic epidermis in fracture treated with G/C-Au@SiO2 (1–3) were observed compared to those in a bare fracture in the histological sections shown (). The nanomaterials also increased the healing rate of the fracture treated with G/C-Au@SiO2 (1–3), which is apparent from the histological micrographs. Au@SiO2 nanomaterials, which possess biocompatibility and anticoagulant properties, are ideal for fracture cure, as seen in vivo studies. Light microscopy images of the sections showed complete quantitative regeneration of fresh subcutaneous tissues. In addition, the degree of fracture closure was assessed macroscopically. The findings () implied that G/C-Au@SiO2 (1–3) therapy facilitated substantial healing through fibroblast migration, adequate growth of epithelial cells, and blood flow restoration due to the addition of new blood vessels. We also developed a bandaging material focused on G/C-Au@SiO2 (1–3) hydrogels, which for the first time to the best of our knowledge, were tested to be used for fracture healing to improve fracture dressing techniques.
4. Conclusions
In conclusion, this work has effectively fabricated Au@SiO2 HNPs incorporated with G/C conductive hydrogels. The porous G/C-Au@SiO2 conductive hydrogels have improved electrical and mechanical properties compared to pure G/C hydrogels. Degradation and swelling behaviour of the conductive hydrogel was also enhanced in this case. In addition, G/C-Au@SiO2 conductive hydrogels showed enhanced MG-63 cell adhesion and homogeneous cell growth. Therefore, it is possible to improve hydrogel scaffolding technology by adding dual-functional HNPs using the straightforward manufacturing approach. It was proposed in the study, as well, that bone tissue engineering might benefit from the use of G/C-Au@SiO2 conducting hydrogels. Further characterization will be performed to validate the potential of these kinds of hybrid hydrogels for other biomedical applications and mainly fracture tissue engineering research.
Disclosure statement
The authors declare no competing financial interest.
Data availability statement
All data generated or analysed during this study are included in this submitted article. The raw data shall be made available upon request to the corresponding author.
References
- Sun X, Yin H, Wang Y, et al. In situ articular cartilage regeneration through endogenous reparative cell homing using a functional bone marrow-specific scaffolding system. ACS Appl Mater Interfaces. 2018;10(45):38715–38728.
- Li C, Wang K, Li T, et al. Patient-specific scaffolds with a biomimetic gradient environment for articular cartilage–subchondral bone regeneration. ACS Appl Bio Mater. 2020;3(8):4820–4831.
- Radhakrishnan J, Subramanian A, Krishnan UM, et al. Injectable and 3D bioprinted polysaccharide hydrogels: from cartilage to osteochondral tissue engineering. Biomacromolecules. 2017;18(1):1–26.
- Chen G, Dong C, Yang L, et al. 3D scaffolds with different stiffness but the same microstructure for bone tissue engineering. ACS Appl Mater Interfaces. 2015;7(29):15790–15802.
- Zhang N, Lock J, Sallee A, et al. Magnetic nanocomposite hydrogel for potential cartilage tissue engineering: synthesis, characterization, and cytocompatibility with bone marrow derived mesenchymal stem cells. ACS Appl Mater Interfaces. 2015;7(37):20987–20998.
- Shen T, Dai Y, Li X, et al. Regeneration of the osteochondral defect by a wollastonite and macroporous fibrin biphasic scaffold. ACS Biomater Sci Eng. 2018;4(6):1942–1953.
- Peng D, Han B, Kong Y, et al. Facile synthesis and characterization of Au nanoparticles-loaded kaolin mediated by Thymbra spicata extract and its application on bone regeneration in a rat calvaria defect model and screening system. J Exp Nanosci. 2022;17(1):86–99.
- Runqin H, Fenglian N, Qiuxiang C. Mechanical properties of TiO2-filled CNT/PMMA composites. J Exp Nanosci. 2017;12(1):308–318.
- Shuai C, Nie Y, Gao C, et al. The microstructure evolution of nanohydroxapatite powder sintered for bone tissue engineering. J Exp Nanosci. 2013;8(5):762–773.
- Zhao Y, Ding X, Dong Y, et al. Role of the calcified cartilage layer of an integrated trilayered silk fibroin scaffold used to regenerate osteochondral defects in rabbit knees. ACS Biomater Sci Eng. 2020;6(2):1208–1216.
- Simson JA, Strehin IA, Lu Q, et al. An adhesive bone marrow scaffold and bone morphogenetic-2 protein carrier for cartilage tissue engineering. Biomacromolecules. 2013;14(3):637–643.
- Zhang B, Huang J, Narayan RJ. Gradient scaffolds for osteochondral tissue engineering and regeneration. J Mater Chem B. 2020;8(36):8149–8170.
- Tian L, Wei M, Ji L, et al. Fabrication and investigation of cardiac patch embedded with gold nanowires for improved myocardial infarction therapeutics. J Exp Nanosci. 2021;16(1):212–228.
- Park KM, Choi JH, Bae JW, et al. Nano-aggregates using thermosensitive chitosan copolymers as a nanocarrier for protein delivery. J Exp Nanosci. 2009;4(3):269–275.
- Negi LM, Chauhan M, Garg AK. Nano-appended transdermal gel of tenoxicam via ultradeformable drug carrier system. J Exp Nanosci. 2013;8(5):657–669.
- Jamshidi P, Ma P, Khosrowyar K, et al. Tailoring gel modulus using dispersed nanocrystalline hydroxyapatite. J. Exp. Nanosci. 2012;7(6):652–661.
- Feng X, Zhou T, Xu P, et al. Enhanced regeneration of osteochondral defects by using an aggrecanase-1 responsively degradable and N-cadherin mimetic peptide-conjugated hydrogel loaded with BMSCs. Biomater Sci. 2020;8(8):2212–2226.
- Zhang K, He S, Yan S, et al. Regeneration of hyaline-like cartilage and subchondral bone simultaneously by poly(l-glutamic acid) based osteochondral scaffolds with induced autologous adipose derived stem cells. J Mater Chem B. 2016;4(15):2628–2645.
- Wu X, Zhou M, Jiang F, et al. Marginal sealing around integral bilayer scaffolds for repairing osteochondral defects based on photocurable silk hydrogels. Bioact Mater. 2021;6(11):3976–3986.
- Dashnyam K, Lee J-H, Singh RK, et al. Optimally dosed nanoceria attenuates osteoarthritic degeneration of joint cartilage and subchondral bone. Chem Eng J. 2021;422:130066.
- Radhakrishnan J, Manigandan A, Chinnaswamy P, et al. Gradient nano-engineered in situ forming composite hydrogel for osteochondral regeneration. Biomaterials. 2018;162:82–98.
- Magli S, Rossi GB, Risi G, et al. Design and synthesis of chitosan–gelatin hybrid hydrogels for 3D printable in vitro models. Front Chem. 8 2020. https://www.frontiersin.org/article/103389/fchem.2020.00524.
- Baei P, Jalili-Firoozinezhad S, Rajabi-Zeleti S, et al. Electrically conductive gold nanoparticle-chitosan thermosensitive hydrogels for cardiac tissue engineering. Mater Sci Eng C. 2016;63:131–141.
- Qiao Z, Lian M, Han Y, et al. Bioinspired stratified electrowritten fiber-reinforced hydrogel constructs with layer-specific induction capacity for functional osteochondral regeneration. Biomaterials. 2021;266:120385.
- Xing J, Peng X, Li A, et al. Gellan gum/alginate-based Ca-enriched acellular bilayer hydrogel with robust interface bonding for effective osteochondral repair. Carbohydr Polym. 2021;270:118382.
- Choi S, Lee JS, Shin J, et al. Osteoconductive hybrid hyaluronic acid hydrogel patch for effective bone formation. J Control Release. 2020;327:571–583.
- Lan W, Xu M, Qin M, et al. Physicochemical properties and biocompatibility of the bi-layer polyvinyl alcohol-based hydrogel for osteochondral tissue engineering. Mater Des. 2021;204:109652.
- Maharjan B, Kumar D, Awasthi GP, et al. Synthesis and characterization of gold/silica hybrid nanoparticles incorporated gelatin methacrylate conductive hydrogels for H9C2 cardiac cell compatibility study. Compos Part B Eng. 2019;177:107415.
- Tsuzuki N, Oshita N, Seo J, et al. Effect of platelet-rich plasma-incorporated gelatin hydrogel microspheres and subchondral drilling on equine cartilage defects. J Equine Vet Sci. 2014;34(6):820–824.
- Riveiro A, Amorim S, Solanki A, et al. Hyaluronic acid hydrogels reinforced with laser spun bioactive glass micro- and nanofibres doped with lithium. Mater Sci Eng C Mater Biol Appl. 2021;126:112124.
- Zhang W, Zhang Y, Zhang A, et al. Enzymatically crosslinked silk-nanosilicate reinforced hydrogel with dual-lineage bioactivity for osteochondral tissue engineering. Mater Sci Eng C. 2021;127:112215.
- Hettinghouse A, Katyal P, Chen C, et al. Sustained delivery of pgrn-derivative atsttrin via e5c hydrogel protects cartilage and bone quality in a rabbit model of post-traumatic osteoarthritis. Osteoarthr Cartil. 2021;29:S193–S194.
- Liu P, Li M, Yu H, et al. Biphasic CK2.1-coated β-glycerophosphate chitosan/LL37-modified layered double hydroxide chitosan composite scaffolds enhance coordinated hyaline cartilage and subchondral bone regeneration. Chem Eng J. 2021;418:129531.
- Re’em T, Witte F, Willbold E, et al. Simultaneous regeneration of articular cartilage and subchondral bone induced by spatially presented TGF-beta and BMP-4 in a bilayer affinity binding system. Acta Biomater. 2012;8(9):3283–3293.
- Marionneaux A, Walters J, Guo H, et al. Tailoring the subchondral bone phase of a multi-layered osteochondral construct to support bone healing and a cartilage analog. Acta Biomater. 2018;78:351–364.
- Zhao Y, Gao C, Liu H, et al. Infliximab-based self-healing hydrogel composite scaffold enhances stem cell survival, engraftment, and function in rheumatoid arthritis treatment. Acta Biomater. 2021;121:653–664.
- Guo JL, Kim YS, Koons GL, et al. Bilayered, peptide-biofunctionalized hydrogels for in vivo osteochondral tissue repair. Acta Biomater. 2021;128:120–129.
- Liu J, Lu Y, Xing F, et al. Cell-free scaffolds functionalized with bionic cartilage acellular matrix microspheres to enhance the microfracture treatment of articular cartilage defects. J Mater Chem B. 2021;9(6):1686–1697.
- Yang W, Cao Y, Zhang Z, et al. Targeted delivery of FGF2 to subchondral bone enhanced the repair of articular cartilage defect. Acta Biomater. 2018;69:170–182.
- Li JJ, Kaplan DL, Zreiqat H. Scaffold-based regeneration of skeletal tissues to meet clinical challenges. J Mater Chem B. 2014;2(42):7272–7306.
- Singh YP, Moses JC, Bhardwaj N, et al. Injectable hydrogels: a new paradigm for osteochondral tissue engineering. J Mater Chem B. 2018;6(35):5499–5529.
- Camarero-Espinosa S, Rothen-Rutishauser B, Foster EJ, et al. Articular cartilage: from formation to tissue engineering. Biomater Sci. 2016;4(5):734–767.
- Chien DM, Dung DTM, Dam LD. Preparation of nitrogen co-doped SiO2/TiO2 thin films on ceramic with enhanced photocatalytic activity under visible-light irradiation. J Exp Nanosci. 2012;7(3):254–262.
- Lim H-S, Kim B, Suh K-D. Multiwalled carbon nanotube/SiO2 composite nanofibres prepared by electrospinning. J Exp Nanosci. 2010;5(4):329–336.
- Huo S-F, Zhang Z-C, Ma Q-L. Surface enhanced Raman scattering on SiO2/Ag nanoparticles aggregate and preparation of nitrogen-doped carbon dots by pyrolysis of Co(2,2′-bipyridine)2(dicyanamide)2. J Exp Nanosci. 2016;11(8):669–680.
- Diegoli S, Mendes PM, Baguley ER, et al. pH-dependent gold nanoparticle self-organization on functionalized Si/SiO2 surfaces. J Exp Nanosci. 2006;1(3):333–353.
- Jiang G, Li S, Yu K, et al. A 3D-printed PRP-GelMA hydrogel promotes osteochondral regeneration through M2 macrophage polarization in a rabbit model. Acta Biomater. 2021;128:150–162.
- Yuan Q, Shah J, Hein S, et al. Controlled and extended drug release behavior of chitosan-based nanoparticle carrier. Acta Biomater. 2010;6(3):1140–1148.
- Dharmalingam K, Anandalakshmi R. Functionalization of cellulose-based nanocomposite hydrogel films with zinc oxide complex and grapefruit seed extract for potential applications in treating chronic wounds. Polymer (Guildf). 2020;202:122620.
- Nataraj D, Aramwit P, Nagananda GS, et al. Development, characterization and evaluation of the biocompatibility of catechol crosslinked horsegram protein films. Eur Polym J. 2020;134:109800.
- Depan D, Venkata Surya PKC, Girase B, et al. Organic/inorganic hybrid network structure nanocomposite scaffolds based on grafted chitosan for tissue engineering. Acta Biomater. 2011;7(5):2163–2175.
- Masud RA, Islam MS, Haque P, et al. Preparation of novel chitosan/poly (ethylene glycol)/ZnO bionanocomposite for wound healing application: effect of gentamicin loading. Materialia. 2020;12:100785.
- Chen M, Tian J, Liu Y, et al. Dynamic covalent constructed self-healing hydrogel for sequential delivery of antibacterial agent and growth factor in wound healing. Chem Eng J. 2019;373:413–424.
- Synytsya A, Poučková P, Zadinová M, et al. Hydrogels based on low-methoxyl amidated citrus pectin and flaxseed gum formulated with tripeptide glycyl-l-histidyl-l-lysine improve the healing of experimental cutting wounds in rats. Int J Biol Macromol. 2020;165(Pt B):3156–3168.
- Zhang S, Ou Q, Xin P, et al. Polydopamine/puerarin nanoparticle-incorporated hybrid hydrogels for enhanced wound healing. Biomater Sci. 2019;7(10):4230–4236.
- Mohamed Subarkhan MK, Ramesh R, Liu Y. Synthesis and molecular structure of arene ruthenium(II) benzhydrazone complexes: impact of substitution at the chelating ligand and arene moiety on antiproliferative activity. New J Chem. 2016;40(11):9813–9823.
- Subarkhan MKM, Ramesh R. Ruthenium(II) arene complexes containing benzhydrazone ligands: Synthesis, structure and antiproliferative activity. Inorg Chem Front. 2016;3(10):1245–1255.
- Mohan N, Mohamed Subarkhan MK, Ramesh R. Synthesis, antiproliferative activity and apoptosis-promoting effects of arene ruthenium(II) complexes with N, O chelating ligands. J Organomet Chem. 2018;859:124–131.
- Sathiya Kamatchi T, Mohamed Subarkhan MK, Ramesh R, et al. Investigation into antiproliferative activity and apoptosis mechanism of new arene Ru(ii) carbazole-based hydrazone complexes. Dalton Trans. 2020;49(32):11385–11395.
- Balaji S, Mohamed Subarkhan MK, Ramesh R, et al. Synthesis and structure of arene Ru(II) N∧O-chelating complexes: in vitro cytotoxicity and cancer cell death mechanism. Organometallics. 2020;39(8):1366–1375.
- Chung CYS, Fung SK, Tong KC, et al. A multi-functional PEGylated gold(iii) compound: potent anti-cancer properties and self-assembly into nanostructures for drug co-delivery. Chem Sci. 2017;8(3):1942–1953.
- Deng B, Ma P, Xie Y. Reduction-sensitive polymeric nanocarriers in cancer therapy: a comprehensive review. Nanoscale. 2015;7(30):12773–12795.
- Margiotta N, Savino S, Denora N, et al. Encapsulation of lipophilic kiteplatin Pt(IV) prodrugs in PLGA-PEG micelles. Dalton Trans. 2016;45(33):13070–13081.
- Kalaiarasi G, Mohamed Subarkhan M, Fathima Safwana CK, et al. New organoruthenium(II) complexes containing N, X-donor (X = O, S) heterocyclic chelators: synthesis, spectral characterization, in vitro cytotoxicity and apoptosis investigation. Inorg Chim. Acta. 2022;535:120863.
- Swaminathan S, Haribabu J, Mohamed Subarkhan MK, et al. Impact of aliphatic acyl and aromatic thioamide substituents on the anticancer activity of Ru(ii)-p-cymene complexes with acylthiourea ligands-in vitro and in vivo studies. Dalton Trans. 2021;50(44):16311–16325.
- Giriraj K, Mohamed Kasim MS, Balasubramaniam K, et al. Various coordination modes of new coumarin Schiff bases toward cobalt (III) ion: Synthesis, spectral characterization, in vitro cytotoxic activity, and investigation of apoptosis. Appl Organomet Chem. 2022;36:e6536.
- Sonamuthu J, Cai Y, Liu H, et al. MMP-9 responsive dipeptide-tempted natural protein hydrogel-based wound dressings for accelerated healing action of infected diabetic wound. Int J Biol Macromol. 2020;153:1058–1069.