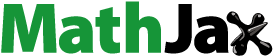
Abstract
TiO2 NPs and ITO NPs embedded systems are vital for surface modification in various applications. Enhancing the characterization of TiO2 NPs/ITO NPs nanoparticles to improve application efficiency is a key research direction. This study focuses on characterizing nanocomposites using TEM, SEM, XRD, UV-Vis spectroscopy, EDS, and PL spectroscopy. TiO2 NPs and ITO NPs were separately prepared using the sol-gel method, and their hybridization was achieved through an appropriate method. TEM images reveal the nano size of TiO2 NPs and TiO2 NPs/ITO NPs, with mean diameters of 12.25 ± 13 nm and 11.43 ± 11 nm, respectively. Hexagonal structures like nanoparticles are observed in the TEM analysis. SEM images demonstrate the uniform dispersion and smooth surface morphology of TiO2 NPs/ITO NPs. XRD analysis confirms the crystalline nature of TiO2 NPs, corresponding to specific crystallographic planes in the hexagonal structure. The addition of ITO NPs induces a redshift in the UV-Vis spectra, suggesting an influence on the bandgap of TiO2 NPs and potential applications in photocatalytic degradation and solar energy utilization. Photoluminescence spectroscopy reveals optical characteristics of the TiO2 NPs/ITO NPs hybrid nanostructures, with a visible emission peak redshift nm at room temperatures ranging from 400 °C to 600 °C. These findings contribute to understanding the optical behavior and interaction between ITO NPs and TiO2 NPs in the hybrid nanostructures. Overall, these characterization techniques provide valuable information on size, shape, crystallinity, optical properties, and interactions within the nanocomposites, benefiting further research and potential applications.
1. Introduction
A basic awareness of the physical characteristics and behaviors of nano-materials and nanostructures, as well as their design, manufacture, and use, can be used to define nanotechnology [Citation1,Citation2]. Contrary to bulk materials, nanomaterials, which range in size from 1 to 100 nm, have extraordinary surface areas and distinctive features in the disciplines of magnetism, electricity, optics, mechanics, solar cells [Citation3], sensor and catalysis [Citation4]. The world is currently being inspired by metal oxide nanoparticles (NPs) [Citation5–8] because of the prospective uses for them. Since they are well-known n-type transition-metal semiconductors, for example, TiO2 NPs have been chosen as alternative electrodes on solar cells for photocatalysis, sensing, and photoluminescence due to their easy and consistent manufacturing and distinctive electrical and optical properties [Citation5,Citation9]. For its remarkable physicochemical stability, photochemical activity, and high dielectric constant, titanium dioxide, often known as titania (TiO2), is one of among the most promising materials for numerous applications. It has been predicted that TiO2 NPs will be important in a variety of industries [Citation10,Citation11]. The use of TiO2 NPs as pigments [Citation12], catalysts [Citation13], support ceramics [Citation14], gas sensors, inorganic membranes [Citation15], waste water purification [Citation16], and solar energy conversion has attracted increasing interest in recent years [Citation17,Citation18]. On the other hand, TiO2 NPs have garnered considerable interest due to their exceptional photocatalytic activity, which finds applications in environmental remediation, water splitting, and solar energy conversion [Citation5,Citation16,Citation19,Citation20]. In recent years, the development and utilization of nanomaterials have gained significant attention in various fields due to their unique properties and potential applications [Citation1,Citation21,Citation22]. Among these nanomaterials, indium tin oxide nanoparticles (ITO NPs) and titanium dioxide nanoparticles (TiO2 NPs) have emerged as promising candidates owing to their excellent electrical [Citation23], optical, and catalytic properties. Individually, ITO NPs [Citation24] have been extensively studied for their high electrical conductivity and transparency [Citation25] in the visible region, making them suitable for applications in optoelectronic devices, such as transparent conductive coatings for solar cells [Citation26,Citation27], touchscreens, and displays [Citation28].
However, to further enhance the properties and functionalities of these nanoparticles regarding their optical properties, researchers did not explored the incorporation of ITO NPs into TiO2 NPs through a doping process yet. The incorporation of ITO NPs into TiO2 NPs has the potential to synergistically combine the beneficial properties of both materials, leading to enhanced performance and novel applications. In addition to hybridizing, post-synthesis thermal treatment, such as annealing, is a commonly employed technique to manipulate the structural and optical properties of nanomaterials [Citation29–31]. This process allows for the rearrangement of atoms, crystallization, and elimination of defects, ultimately influencing the material’s structure, morphology, and optical behavior [Citation32].
In this study, we aim to investigate the effect of annealing temperature on the structural and optical properties of ITO NPs/TiO2 NPs. We will systematically vary the annealing temperature over a range of values and characterize the resulting materials using various analytical techniques. Through this comprehensive analysis, we seek to gain insights into the structural changes, phase transformations, and optical behavior of the doped TiO2 NPs with ITO NPs, providing a foundation for their potential applications in optoelectronics, photocatalysis, and related fields. Overall, this research aims to contribute to the fundamental understanding of nanomaterials and provide valuable insights into the design and optimization of advanced functional materials by exploring the synergistic effects of doping ITO NPs into TiO2 NPs and the impact of annealing temperature on their structural and optical properties. It can be achieved through a sol-gel synthesis methods. This paves the way for the development of advanced functional materials with improved performance and expanded applications in various fields.
2. Experimental part
2.1. Reagents
Titanium (IV) chloride (TiCl4 99%, France), indium (III) chloride (InCl3. 4H2O, 99.9%) brought form Millipore sigma, deionized water, tin (IV) chloride (SnCl4. 5H2O, 98%, Germany), ammonium hydroxide (NH3) solution (25%), ethyl alcohol (99.5%), ethylenediamine (EDA) (99.5%) and acetonitrile (99.5%) all are bought from Sigma-Alderich.
2.2. (1) Synthesis of TiO2 nanoparticle, Indium tin Oxide nanoparticles (ITO NPs) and characterization
TiO2 powder was made using titanium (IV) chloride, TiCl4 (99%) as a starting material. During the method, hydrous titanium oxides were precipitated after 3 ml of titanium (IV) chloride was gently introduced dropwise into 25 ml of deionized water. For 40 min, the solution was continuously stirred at 60 °C to ensure thorough mixing. After completing this procedure, a white precipitate solution was appeared at the bottom of the beaker. These were centrifuged out, washed numerous times in deionized water and methanol, and then dried for 12 h at 80 °C. Then treated at different temperature (400 °C −600 °C) to get pure TiO2 powder.
2.3. (2) Preparation of ITO nanoparticles by co-precipitation method
Indium (III) chloride (InCl3.4H2O) weighing 2.6 g and Tin (IV) chloride (SnCl4.5H2O) weighing 0.26 g were dissolved in 10 ml of distilled water at a temperature range of 40 °C to 50 °C. The resulting solution was stirred for 30 min. To control the dispersant, 1.4 ml of 5% ammonia solution was added to the mixture and stirred for 10 min. The pH level was monitored to ensure controlled acidity. As a precipitant, 0.76 ml of ethylene diamine solution was added. The obtained precipitant was stirred for a specific duration, then washed with deionized water and ethyl alcohol. After washing, the precipitant was dried at 120 °C for 1 h. Subsequently, it was calcined at a temperature range of 450 °C to 600 °C for 1 h to obtain ITO nano-powder.
3. Results and discussions
3.1. Characterizations of composites
In this case, the nanocomposites consist of TiO2 NPs and ITO NPs TEM images () were used to determine the size and shape of the nanocomposites. The mean diameter of the nanograins in the TiO2 NPs and TiO2 NPs@ITO NPs (where ‘@’ indicates the presence of ITO NPs) was found to be 12.25 ± 13 nm and 11.43 ± 11 nm, respectively. These values were comparable to previously published data [Citation33], indicating that the nanocomposites were similar in size to those reported in earlier studies. Furthermore, when examining the TEM images of TiO2 NPs (at a ITO NPS concentration of 10 wt %), smaller nanoparticles were observed. This suggests that the combination of TiO2 NPs and ITO NPs of various sizes interacted favorably, leading to the formation of smaller nanograins. The hexagonal structures seen in TEM indicate that the TiO2 NPs possessed beneficial properties, as they developed into this specific shape. Additionally, within the hexagonal structure, some locations showed the presence of sphere-like nanoparticles. The particle sizes obtained from the TEM analysis, 12.25 ± 13 nm and 11.43 ± 11 nm, suggest the possibility of a heterojunction formation between the TiO2 NPs and ITO NPs, respectively. This indicates that the different nanoparticles may have formed interfaces or junctions, potentially leading to unique properties or enhanced performance in the nanocomposites. Furthermore, demonstrates that the incorporation of ITO NPs on top of TiO2 NPs increased the surface roughness. This suggests that the presence of ITO NPs altered the surface characteristics of the nanocomposites, potentially influencing their optical or electronic properties. Thus, the result analysis provided valuable insights into the size, shape, and interactions between the TiO2 NPs and ITO NPs in the nanocomposites for their potential applications.
Figure 1. TEM image of (A) TiO2 NPs, (B) TiO2 NPs@ITO NPs, (C) SEAD pattern of TiO2 NPs, (D) SEAD pattern of TiO2 NPs@ITO NPs, (E) SEM image of TiO2 NPs, (F) SEM image of TiO2 NPs@ITO NPs, (G) Size distribution of TiO2 and (H) size distribution of TiO2 NPs@ITO NPs, (I) EDX image of TiO2 NPS and (J) EDX image of TiO2 NPs@ITO NPs.
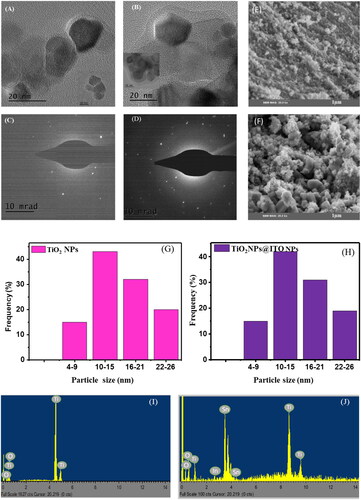
SAED (Selected Area Electron Diffraction) characterization which corresponds with TEM were used to confirm the crystalline structure of TiO2 nano-crystallites. , which are characteristic samples from , display a typical result. Several Debye-Sherrer rings, including those with numbers (101), (004), (200), (211), (204), (220) and (215), were indicated following JCPDS-ICDD card no. 21-1272 [Citation34] of the anatase phase, which corresponded with the XRD result. The polycrystalline grain formation-related diffused continuous ring system was visible in the SAED pattern.
SEM images of TiO2 nanoparticles (NPs) in display the agglomeration of particles with varying shapes, indicating a non-uniform dispersion. The SEM analysis confirms the homogeneous and smooth surface morphology of the TiO2 nanoparticles. The crystalline structure and form of the TiO2 nanoparticles remain intact in the nanocomposites. However, the morphology of TiO2 NPs undergoes a noticeable change when treated with ITO NPs, as evident in . This alteration can be attributed to surface interactions between the two types of nanoparticles. Upon treatment, ITO NPs can adsorb or deposit onto the surface of the TiO2 NPs, leading to modifications in their surface properties and morphology. The interaction between TiO2 NPs and ITO NPs may involve chemical reactions at the nanoparticle surfaces, resulting in the formation of new compounds or surface modifications that influence the morphology of the TiO2 NPs. It should be emphasized that the specific reasons behind the observed changes in morphology depend on various factors such as experimental conditions, nanoparticle characteristics, and interaction mechanisms between TiO2 and ITO NPs in the particular study mentioned.
From the size distribution histogram of TiO2 NPs and TiO2 NPs@ITO NPs materials, numerous dimension sizes can be seen with all of the categories that the particles are aggregated into, with the average size being roughly 10–15 nm for both TiO2 NPs and ITO NPs materials, it can be confirm the majority of the particle size can be found in the mentioned range above calculated particle size from the TEM.
The EDS (Energy Dispersive X-ray Spectroscopy) pattern, depicted in , provided evidence that the analyzed sample contained only two elements: titanium (Ti) and oxygen (O). This suggests that other trace elements were not detected in the synthesized nanopowder. However, when considering the atomic percentage and weight percentage, the composition of the TiO2 nanoparticles (NPs) indicated that it consisted entirely of Ti and O. This finding implies that the quantities of the elements involved in the reaction or compound formation of TiO2 align with the atomic ratio observed in , confirming a nearly equal ratio of Ti to O. Furthermore, displays the EDS pattern analysis, which reveals the presence of oxygen (O), titanium (Ti), tin (Sn), and indium (In) in the composite part. This indicates the coexistence of TiO2 and indium tin oxide (ITO). The corresponding weight percentage (wt %) and atomic percentage (At %) values for each element, as shown in , provide supporting evidence for these findings.
Table 1. EDS (elemental composition Wt % and at %) and band gap of TiO2 NPs, TiO2 NPs@ITO NPs.
In and , the provided details illustrate how the peak intensities change with temperature, visually representing the alterations in crystallinity and the decreasing intensities of the peaks.
Figure 2. XRD of (A) TiO2 NPs at different temperature and (B) TiO2 NPs@ITO NPs at different deposited mass of ITO NPs.
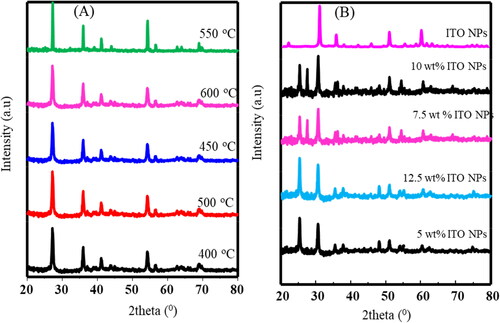
Table 2. XRD diffraction peak and d-spacing and values for TiO2 NPs produced by sol-gel synthesis at various annealing temperatures, correlating to their respective indices.
The ITO NPs XRD peaks diffraction at 2θ = 21.77 °C, 30.74 °C, 35.72 °C, 37.90 °C, 42.21 °C, 46.04 °C, 51.35 °C, 56.27 °C, 59.55 °C, 60.91 °C have been observed for the planes (211), (222), (400), (411), (332), (431), (440), (433), (611), and (622), respectively, show the Cubic like structure (JCPDS 06–0416)29 [Citation35]. Additionally, it is observed that as the temperature rose to 550 °C, the intensity of the TiO2 NPs increased. Here, the TiO2NPs’ atoms were able to reorganize themselves into a more ordered lattice as the temperature rose, which enhanced the crystallinity of the material. All of the diffraction peaks’ intensities, however, diminished as the temperature rose to 500 °C. A decline in peak intensity points to a possible reduction in X-ray scattering, which may be caused by a decline in the TiO2 nanoparticles’ crystalline quality. Grain development, lattice flaws, and even phase transitions may cause a drop in crystalline quality and, as a result, a fall in peak intensity at greater temperatures.
and demonstrate that the XRD peak positions and intensities of TiO2 NPs change when ITO NPs are added. This indicates that the presence of ITO NPs affects the structure of TiO2 NPs. The composite of TiO2 NPs/ITO NPs clearly exhibits all the known XRD peaks of ITO NPs. However, the peak positions and d-spacing of TiO2 NPs shift after the addition of ITO NPs. These shifts are evident in , which shows the changes at different annealing temperatures and different percentages of deposited ITO NPs. This suggests that ITO NPs have an impact on the crystallography of TiO2 NPs, which may have potential applications in other fields. The lattice parameters for TiO2 NPs and TiO2 NPs@ITO NPs, calculated from XRD peaks = 3.124 Å and
= 4.642 Å and
=3.214 Å and
= 4.725 Å. are respectively.
Utilizing the powder XRD method, the produced TiO2 film was examined to determine its crystal structure; the outcome is shown in . The production of crystalline TiO2 is indicated by the strong and intense diffraction peaks. The anatase TiO2 (JCPDS No. 21-1272) crystal planes [Citation36] can be indexed to the (101), (004), (200), (105), (211), (204), (105), (220) and (215) diffraction peaks at 2θ = 25.41°, 37.83°, 48.45°, 54.35°, 55.90°,62.8°, 68.97°, 70.30° and 75.11 °C respectively at 550 °C annealing temperature.
3.2. Optical properties
Regardless of the temperatures used during the annealing process, the highest peaks in the measurements of TiO2 nanoparticles are consistently observed at a wavelength of 340 nm, as shown in of the UV-Vis spectrum. However, a shift towards longer wavelengths (redshift) in the TiO2 nanoparticle spectra is only noticeable at specific temperatures, specifically at 550 °C. As the TiO2 nanoparticles are subjected to different temperatures, their actual energy gap changes accordingly (seen in ). However, when 10% by weight of ITO (Indium Tin Oxide) nanoparticles are added to TiO2 nanoparticles, a new redshifted wavelength with a modified band spectrum of TiO2 nanoparticles appears at 430 nm, as depicted in . This shift can be attributed to the movement of electrons from the valence band (VB) to the conduction band (CB) energy levels.
Figure 3. UV-Vis of (A) TiO2 NPs at different temperature, (B) TiO2 NPs (550 °C) at different deposited mass of ITO NPs, (C) Band gap of TiO2 NPs at different temperature, (D) Correlation of band gap with ITO NPs mass weight, (E) Band gap of TiO2 NPs at different temperature, (F) Correlation of band gap and ITO NPs mass deposited.
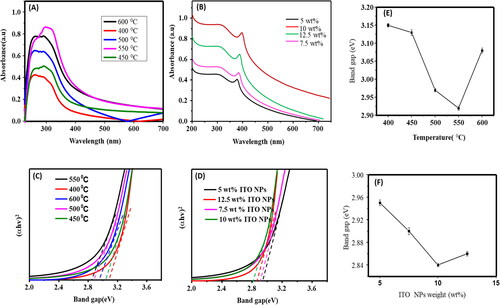
The change of the band gap of TiO2 NPs at different annealing temperatures and the band gap of TiO2 NPs at different weight percentages of ITO NPs is demonstrated by the tauc plots of TiO2 NPs and TiO2 NPs/ITO NPs shown in , and . As a result, the band gap of shows a decreasing trend from 400 °C to 600 °C, but an increase beyond 550 °C demonstrating how the annealing temperature can affect the materials’ band gap, which relates to their conductivity. The tauc plots seen in and of TiO2 NPs and TiO2 NPs/ITO NPs demonstrate, the variation of the band gap of TiO2 NPs at different annealing temperature and the band gap of TiO2 NPs at different wt % of ITO NPs. Consequently, as temperature raised from 400 °C −600 °C the band gap of the shows decreasing, but after the 550 °C to increase. Indicating the annealing temperature can influence the band gap of the materials which corresponded to the conductivity of the materials. As seen and , the small band gap seen at 550 °C.
Furthermore, the band gap change of TiO2 NPs at 10 wt% of ITO NPs decreased to 2.84 eV from 2.92 eV when treated by varied wt% of ITO NPs (see and ). It can be assigned that the chemical reactions or alloying at the interface may result from the interaction of TiO2 and ITO NPs. This may alter the TiO2 NPs’ electrical structure or add new energy levels, which would impact the band gap. The total band gap of the nanocomposite may change if ITO NPs are present, since they usually have a lower band gap than TiO2 O. Furthermore, dopants can alter the band gap’s breadth by generating energy levels within it. The annealing temperature or other factors can affect the type and amount of faults or dopants.
In , the deconvoluted line spectra of the peaks are also displayed for TiO2 NPs (O 1s, 2P3/2), TiO2 NPs@ITO NP (O 1s, 2P3/2). As revealed from the XPS fine spectra in , the difference of binding energies and area intensity of numerical values changed. The two deconvoluted banding energy of O 1s of TiO2 was reduced to 529.7 and 533.1 eV from 531.5 and 534.1 eV after the addition of ITO NPs. This implies that TiO2 NPs and ITO NPs have been interacted. TiO2 is proven to exist by the appearance of the characteristic Ti 2p (Ti 2p3/2 and Ti 2p1/2) peaks at strong double peaks, which are associated with Ti − O and Ti − OH bonds, respectively. The 529.7 and 533.1 eV peaks of TiO2@ITO NPs at 531.5 and 534.1 eV had the largest area intensities of O 1s binding energies of TiO2 NPs, suggesting that the O 1s quantity in the ITO NPs had replaced the intensity of O 1s. Nevertheless, because ITO NPs contributed to change binding-energy peaks, the relative area intensities of O 1s and 2P3/2 peaks of TiO2 NPs @ITO NPs differed from those of TiO2 NPs.
In this work, we employed a unique synthesis method for TiO2 NPs by incorporating a precursor of TiCl4 in DI water. This approach sets it apart from previously reported methods that utilized different precursors. By comparing the outcomes of their synthesis approach with similar published papers, the authors can effectively demonstrate the distinctiveness of their method and its significant impact on the structural and optical properties of the TiO2 NPs. Existing methods for doping/depositing materials often face limitations, including a lack of control over structural properties, non-uniform coating thickness or composition, and the potential introduction of defects or impurities during the deposition process. In contrast, the authors have developed a novel doping methodology that overcomes these limitations and enables precise control over the weight mass of deposited materials. By separately preparing and optimizing both the TiO2 NPs and the dopant materials, we successfully achieve impurity-free, pure crystalline structures with enhanced performance. The deposition of ITO NPs onto TiO2 NPs and investigated their structural and optical properties as a function of annealing temperature. While the details of the comparison are provided in , it can be inferred that the synthesis and deposition approaches utilized in this work yield superior results compared to previously published papers. These superior results are evidenced by better control over structural properties, improved uniformity in coating thickness and composition, and enhanced optical characteristics. Overall, our synthesis method using TiCl4 in DI water, their novel doping approach for precise control, and the comparison of their results with similar published papers highlight the advancements made beyond the state-of-the-art in terms of structural control, uniformity, and optical properties.
Table 3. TiO2 performance in different aspects.
3.3. Photoluminescence properties
Understanding how charges recombine in a semiconductor is crucial because it strongly influences the properties and efficiency of dye-sensitized solar cells (DSSCs). Photoluminescence (PL) is a useful tool for studying how effectively charge carriers are trapped, migrate, and transfer, as well as what happens to electron-hole pairs in semiconductor particles. When titanium dioxide (TiO2) absorbs photons with higher energy levels than its band-gap energy, it generates photoinduced charge carriers (positive holes and negative electrons). In , various annealing temperatures reveal distinct photoluminescence (PL) spectra for TiO2 nanoparticles (NPs), with varying PL intensity. The PL intensity of TiO2 exhibited an ascending trend within the temperature range of 400 °C to 600 °C, specifically as follows: 400 °C < 450 °C < 600 °C < 500 °C < 550 °C. This suggests that TiO2 NPs effectively exhibit their PL characteristics at an annealing temperature of 550 °C, which could potentially be suitable for alternative applications.
Figure 5. PL spectra at room temperature of (A) TiO2 NPs at different annealing temperature (B) TiO2 NPs@ITO NPs at different wt% of ITO NPs (C) CIE of TiO2 NPs at different Temperature (D) CIE of TiO2 NPs@ITO NPs at different wt% of ITO NPs.
Furthermore, energy is released in the form of photo-induced electron and hole-recombination, which results in PL emission spectra. Therefore, less charge recombination is indicated by a lower PL intensity. displays the observed PL spectra of TiO2 NPs and TiO2 NPs/ITO NPs. TiO2 NPs and TiO2 NPs/ITO NPs exhibit five peaks with emission at around 395, 497, 550, 624 and 750 nm. Because of the photo-induced charge carriers’ quick recombination, the TiO2 exhibited a greater photoluminescence intensity. When ITO NPs were added to TiO2, the PL intensity dropped. The primary cause of this is the development of a Schottky barrier [Citation42] at the interface between ITO NPs and TiO2 NPs, which may function as an electron sink to effectively stop the electron-hole recombination process.
In addition, It is evident that the PL intensity (seen in is distributed as follows: 5 wt % of ITO NPs (TiO2 > 7.5 wt % of ITO NPs (TiO2 > 12.5 wt % of ITO NPs (TiO2 > 10 wt % of ITO NPs (TiO2>). Long-lived carriers and effective electron-hole separation are shown by the decline in PL intensity. According to this work, a p-n junction that helps with charge separation can be created by depositing ITO NPs on TiO2 surface. Furthermore, rare earth ions might infiltrate the ITO NPs’ lattice and create a shallow trapper, which would extend the carrier’s lifespan and successfully lower the rate at which electrons and holes recombine. Consequently, it can be said that the existence of ITO NPs may be useful to enhance the Photoluminescence performance of TiO2.
Utilizing color calculation software, exhibits the CIE Chromaticity diagram color coordinates, which are obtained from the color-corresponding PL spectra showcased in , correspondingly. The Blue, green/yellow, and red emission under an excitation wavelength of 220 nm are symbolized by the CIE color coordinates of the phosphor, which are (0.4, 0.33), (0.5, 0.5), and (0.7, 0.5) for the 400 °C conditions, (0.4, 0.34), (0.5, 0.52), and (0.7, 0.52) for the 450 °C conditions, (0.35, 0.3), (0.5, 0.8), and (0.7, 0.45) for the 500 °C conditions, (0.35, 0.3), (0.5, 0.8), and (0.7, 0.4) for the 550 °C conditions, and (0.3, 0.2), (0.56, 0.8), and (0.7, 0.4) for the 600 °C conditions, respectively. These color coordinates provide a quantitative representation of the phosphor’s color emissions at different temperatures, allowing for a better understanding of its behavior and characteristics. also demonstrated a significant fluctuation in the color coordinates corresponding to the alteration in the mass of ITO NPs (Indium Tin Oxide Nanoparticles) deposited on TiO2 NPs (Titanium Dioxide Nanoparticles). Specifically, for different weight percentages (wt%) of ITO NPs, the corresponding points on the graph are as follows: For 5 wt%, 7.5 wt%, 10 wt% and 12.5 wt% of ITO NPs, Color coordinates of (0.30, 0.30), (0.5, 0.8), (0.7, 0.4), and (0.35, 0.30), (0.5, 0.8), (0.7, 0.3), and (0.30, 0.20), (0.55, 0.8), (0.7, 0.35) and (0.28, 0.2), (0.55, 0.8), (0.7, 0.4) respectively. These color coordinates represent the correlated color deposition of ITO NPs mass on TiO2 NPs, respectively. The observed variations in the CIE color coordinates, in conjunction with changes in temperature, provide substantial evidence that TiO2 NPs@ITO NPs core/shell nanoparticles hold promising potential for applications in security writing demonstrations.
4. Conclusions
Nanocomposites of TiO2 NPs and ITO NPs were characterized using different techniques. When TiO2 NPs were treated with ITO NPs, TEM and SEM images showed a change in morphology, suggesting potential surface contacts and chemical reactions between the nanoparticles. ITO NPs deposit on the surface of TiO2 NPs as a result of these interactions and reactions, changing the morphology and surface characteristics of the latter. The maximum levels of purity and crystallinity were achieved at an annealing temperature of 550 °C. While the following drop at higher temperatures may be due to variables such as particle development, lattice flaws, or phase transformations impacting the crystalline quality, a rise in XRD intensity of TiO2 NPs with temperature implies enhanced lattice order. It is clear that the presence of ITO NPs has altered the structural characteristics of TiO2 NPs. The TiO2 NPs/ITO NPs composite shows all of the anticipated XRD peaks that correspond to ITO NPs, demonstrating their successful integration. Nevertheless, with the addition of ITO NPs, the peak locations of TiO2 NPs change. This implies that ITO NPs may have an impact on TiO2 NPs’ crystallography, thereby opening up new uses. The band gap of TiO2 NPs changes when temperature changes, however when 10 wt% of ITO NPs are added to TiO2 NP, the band gap of TiO2 NPs decreases significantly, from 2.92 eV to 2.84 eV. The addition of ITO NPs to TiO2 reduces PL intensity due to the development of a Schottky barrier at the interface, preventing electron-hole recombination. Higher 10 wt% of ITO NPs lead to decreased PL intensity, indicating effective electron-hole separation. The presence of ITO NPs can enhance the photoluminescence performance of TiO2 by creating a p-n junction and extending carrier lifespan.
Author’s contributions
HFE and FBD have equally contributed to the grand success of this article. Both ZN Urgessa and JR Botha contributed technically. HFE and ZNU: conceptualization and methodology, formal analysis, investigation; FBD and JRB: validation, resources, writing review and editing; HFE: writing original draft preparation; JRB: visualization; FBD: supervision, project administration and funding acquisition.
Acknowledgements
The authors are grateful to Walter Sisulu University (WSU) for the support
Disclosure statement
No potential conflict of interest was reported by the author(s).
Data availability statement
All data generated or analysed during this research are included in this published article.
Additional information
Funding
References
- Baig N, Kammakakam I, Falath W. Nanomaterials: a review of synthesis methods, properties, recent progress, and challenges. Mater Adv. 2021;2(6):1821–1871. doi: 10.1039/D0MA00807A.
- Osman DAM, Mustafa MA. Synthesis and characterization of zinc oxide nanoparticles using zinc acetate dihydrate and sodium hydroxide. J Nanosci Nanoeng. 2015;1(4):248–251.
- Gemeda GF, Etefa HF, Hsieh C-C, et al. Preparation of ZnO/NiO-loaded flexible cellulose nanofiber film electrodes and their application to dye-sensitized solar cells. Carbohyd Polym Technol Appl. 2022;3:100213. doi: 10.1016/j.carpta.2022.100213.
- Belay T, Worku LA, Bachheti RK, et al. Nanomaterials: introduction, synthesis, characterization, and applications. In: Advances in smart nanomaterials and their applications. Amsterdam: Elsevier; 2023. p. 1–21.
- Etefa HF, Imae T, Yanagida M. Enhanced photosensitization by carbon dots co-adsorbing with dye on p-type semiconductor (nickel oxide) solar cells. ACS Appl Mater Interfaces. 2020;12(16):18596–18608. doi: 10.1021/acsami.0c02413.
- Etefa HF, Kumar V, Dejene FB, et al. Modification of flexible electrodes for P-type (nickel oxide) dye-sensitized solar cell performance based on the cellulose nanofiber film. ACS Omega. 2023;8(17):15249–15258. doi: 10.1021/acsomega.3c00383.
- Belachew K, Laxmikanth C, Fekede L, et al. Conversion of Mn2+ into Mn3+ in manganese ions doped KF-CaO-B2O3 glasses: electrical and spectroscopic properties. Physica B. 2022;645:414225. doi: 10.1016/j.physb.2022.414225.
- Etefa HF, Kumar V. Hybrid photocatalyst nanomaterials in solar cell applications. In: Multifunctional hybrid semiconductor photocatalyst nanomaterials: application on health, energy and environment. Cham: Springer; 2023. p. 221–238.
- Sargazi S, Simge E, Gelen SS, et al. Application of titanium dioxide nanoparticles in photothermal and photodynamic therapy of cancer: an updated and comprehensive review. J Drug Delivery Sci Technol. 2022;75:103605. doi: 10.1016/j.jddst.2022.103605.
- Armaković SJ, Savanović MM, Armaković S. Titanium dioxide as the most used photocatalyst for water purification: an overview. Catalysts. 2022;13(1):26. doi: 10.3390/catal13010026.
- Arun J, Nachiappan S, Rangarajan G, et al. Synthesis and application of titanium dioxide photocatalysis for energy, decontamination and viral disinfection: a review. Environ Chem Lett. 2023;21(1):339–362. doi: 10.1007/s10311-022-01503-z.
- Nasrollahzadeh M, Atarod M, Jaleh B, et al. In situ green synthesis of Ag nanoparticles on graphene oxide/TiO2 nanocomposite and their catalytic activity for the reduction of 4-nitrophenol, congo red and methylene blue. Ceram Int. 2016;42(7):8587–8596. doi: 10.1016/j.ceramint.2016.02.088.
- Parrino F, Palmisano L. Titanium dioxide (TiO2) and its applications. Amsterdam: Elsevier; 2020.
- Saqib NU, Adnan R, Shah I, et al. Activated carbon, zeolite, and ceramics immobilized TiO2 photocatalysts for the enhanced sequential uptake of dyes and Cd2+ ions. J Dispersion Sci Technol. 2023;44(12):2292–2302. doi: 10.1080/01932691.2022.2070497.
- Yu J, Wang AC, Zhang M, et al. Water treatment via non-membrane inorganic nanoparticles/cellulose composites. Mater Today. 2021;50:329–357. doi: 10.1016/j.mattod.2021.03.024.
- Zahra Z, Habib Z, Chung S, et al. Exposure route of TiO2 NPs from industrial applications to wastewater treatment and their impacts on the agro-environment. Nanomaterials. 2020;10(8):1469. doi: 10.3390/nano10081469.
- Roy J. The synthesis and applications of TiO2 nanoparticles derived from phytochemical sources. J Ind Eng Chem. 2022;106:1–19. doi: 10.1016/j.jiec.2021.10.024.
- Nemera DJ, Etefa HF, Kumar V, et al. Hybridization of nickel oxide nanoparticles with carbon dots and its application for antibacterial activities. Luminescence. 2022;37(6):965–970. doi: 10.1002/bio.4241.
- Verma V, Al-Dossari M, Singh J, et al. A review on green synthesis of TiO2 NPs: photocatalysis and antimicrobial applications. Polymers. 2022;14(7):1444. doi: 10.3390/polym14071444.
- Danish MSS, Estrella LL, Alemaida IMA, et al. Photocatalytic applications of metal oxides for sustainable environmental remediation. Metals. 2021;11(1):80. doi: 10.3390/met11010080.
- Ahmed SF, Mofijur M, Rafa N, et al. Green approaches in synthesising nanomaterials for environmental nanobioremediation: technological advancements, applications, benefits and challenges. Environ Res. 2022;204(Pt A):111967. doi: 10.1016/j.envres.2021.111967.
- Etefa HF, Nemera DJ, Dejene FB. Green synthesis of nickel oxide NPs incorporating carbon dots for antimicrobial activities. ACS Omega. 2023;8(41):38418–38425. doi: 10.1021/acsomega.3c05204.
- Sosna-Głębska A, Szczecińska N, Znajdek K, et al. Review on metallic oxide nanoparticles and their application in optoelectronic devices. Acta Innov. 2019;30:5–15. doi: 10.32933/ActaInnovations.30.1.
- Bai J, Zhou B. Titanium dioxide nanomaterials for sensor applications. Chem Rev. 2014;114(19):10131–10176. doi: 10.1021/cr400625j.
- Azani MR, Hassanpour A, Torres T. Benefits, problems, and solutions of silver nanowire transparent conductive electrodes in indium tin oxide (ITO)‐free flexible solar cells. Adv Energy Mater. 2020;10(48):2002536. doi: 10.1002/aenm.202002536.
- Aydın EB, Sezgintürk MK. Indium tin oxide (ITO): a promising material in biosensing technology. TrAC, Trends Anal Chem. 2017;97:309–315. doi: 10.1016/j.trac.2017.09.021.
- Naghdi S, Rhee KY, Hui D, et al. A review of conductive metal nanomaterials as conductive, transparent, and flexible coatings, thin films, and conductive fillers: different deposition methods and applications. Coatings. 2018;8(8):278. doi: 10.3390/coatings8080278.
- Hofmann AI, Cloutet E, Hadziioannou G. Materials for transparent electrodes: from metal oxides to organic alternatives. Adv Elect Mater. 2018;4(10):1700412. doi: 10.1002/aelm.201700412.
- Shamsi J, Urban AS, Imran M, et al. Metal halide perovskite nanocrystals: synthesis, post-synthesis modifications, and their optical properties. Chem Rev. 2019;119(5):3296–3348. doi: 10.1021/acs.chemrev.8b00644.
- Baig N. Two-dimensional nanomaterials: a critical review of recent progress, properties, applications, and future directions. Compos A Appl Sci Manuf. 2023;165:107362. doi: 10.1016/j.compositesa.2022.107362.
- Rodrigues J, Medeiros S, Vilarinho PM, et al. Optical properties of hydrothermally synthesised and thermally annealed ZnO/ZnO2 composites. Phys Chem Chem Phys. 2020;22(16):8572–8584. doi: 10.1039/D0CP00091D.
- Miao J, Ercius P, Billinge SJ. Atomic electron tomography: 3D structures without crystals. Science. 2016;353(6306):aaf2157. doi: 10.1126/science.aaf2157.
- Rahman A, Harunsani MH, Tan AL, et al. Zinc oxide and zinc oxide-based nanostructures: biogenic and phytogenic synthesis, properties and applications. Bioprocess Biosyst Eng. 2021;44(7):1333–1372. doi: 10.1007/s00449-021-02530-w.
- Toro RG, Diab M, de Caro T, et al. Study of the effect of titanium dioxide hydrosol on the photocatalytic and mechanical properties of paper sheets. Materials. 2020;13(6):1326. doi: 10.3390/ma13061326.
- Nguyen AKQ, Huynh TT, Ho VTT. Preparation and characterization of indium doped tin oxide (ITO) via a non-aqueous sol-gel. Mol Cryst Liq Cryst. 2016;635(1):32–39. doi: 10.1080/15421406.2016.1200920.
- Rane SS, Kajale DA, Arbuj SS, et al. Hydrogen, ethanol and ammonia gas sensing properties of nano-structured titanium dioxide thick films. J Mater Sci Mater Electron. 2017;28(12):9011–9016. doi: 10.1007/s10854-017-6632-0.
- Lin XiuChun LX, Li JingYi LJ, Ma Si MS, et al. Toxicity of TiO2 nanoparticles to Escherichia coli: effects of particle size, crystal phase and water chemistry. PLoS ONE. 2014;9(10):e110247.
- Gao C, Li J, Shan Z, et al. Preparation and visible-light photocatalytic activity of In2S3/TiO2 composite. Mater Chem Phys. 2010;122(1):183–187. doi: 10.1016/j.matchemphys.2010.02.030.
- Ahamed M, Khan MM, Akhtar MJ, et al. Role of Zn doping in oxidative stress mediated cytotoxicity of TiO2 nanoparticles in human breast cancer MCF-7 cells. Sci Rep. 2016;6(1):30196. doi: 10.1038/srep30196.
- Yadeta TF, Imae T. Effect of carbon dot on photovoltaic performance of n-TiO2/p-NiO and n-TiO2/p-CuO heterojunctions in dye-sensitized solar cells. Appl Surf Sci. 2023;637:157880. doi: 10.1016/j.apsusc.2023.157880.
- Li S, Wang Y, Li T, et al. Skin bioinspired anti-ultraviolet melanin/TiO2 nanoparticles without penetration for efficient broad-spectrum sunscreen. Colloid Polym Sci. 2021;299(11):1797–1805. doi: 10.1007/s00396-021-04905-7.
- Kumari A, Ponnam A. Role of sweep direction and substrate work function on resistive switching of titanium-di-oxide [TiO2] nanoparticles. Curr Appl Phys. 2021;25:75–81. doi: 10.1016/j.cap.2021.03.002.