Abstract
The demand for analysis of nanosized particles and assemblies of biologic and inorganic origin has increased in the recent decade together with the growing development of biotechnology and nanotechnology. Recent developments of electrostatic differential mobility analysis (DMA) provide an excellent characterization tool in the nanometer size range. With an increasing number of available nano-DMA (nDMA) systems, the question of data comparability and implementation of possible calibration procedures arise. Here we present analysis of proteins in a range between 3 nm (5.7 kDa) and 15 nm (660 kDa) with five different nDMA systems. Results show differences in the obtained sizes up to 15% between different nDMA systems, which consequently leads to the conclusion that a calibration procedure for each nDMA is necessary when applying such systems for the analysis of nanoparticles with respect to size and molecular mass.
1. Introduction
Differential mobility analysis is a long established method in aerosol physics Citation1 designed to characterise ions and charged particles according to their electrostatic mobility, to analyse ambient aerosols and aerosol products of combustion processes and to measure the formation and alteration of atmospheric aerosol particles Citation1 Citation2. The working range of DMA for these purposes is typically in the upper sub-µm to µm range. In the recent decade, mobility analysers were optimized and further developed to expand their working range into the single digit nanometer range, or in terms of molecular mass, into the low kDa range Citation3–5. This expansion of the working range enabled this technique to be applied in the broad field of chemistry, biochemistry and nanosciences for the analysis of polymers, inorganic nano-sized particles, biopolymers and larger assemblies, such as functional protein complexes, virus-like particles or intact viruses.
The particular advantage of this method compared to mass spectrometry is its high dynamic range in terms of molecular mass (kiloDa to GigaDa) Citation4 and its operation at atmospheric pressure. Combined with charged particle or molecular ion generation from liquid samples with nano-electrospray sources Citation6 Citation7 (n-ES) and subsequent neutralization of the highly charged species Citation8–10, this method has already been successfully applied in analysing important biochemical analytes, such as viruses and virus fragments Citation4 Citation11, bacteriophages, DNA Citation12, proteins and protein complexes Citation4 Citation13, as well as synthetic materials, such as polyethylene glycol polymers Citation14, polystyrene Citation15, PAMAM dendrimers Citation16 and nanometer sized silica particles Citation17.
With increasing distribution and importance of this method, the need for determination of important analytical parameters, such as accuracy and precision, arises. Until now, nano-DMAs (nDMA) were mainly characterized in a sequential setup Citation18 Citation19 Citation20 Citation21 Citation22, in which a first mobility analyser produced monodisperse aerosol particles from a polydisperse aerosol source, and a second mobility analyser scanned the size distribution produced by the first one. This setup enabled the comparison of the quality of the mobility analysis in terms of resolution and particle diffusion, but opens the field for interpretation of the collected results, because both DMAs have influence on the characteristics of the resulting size spectrum.
In investigations reported here we produced a monodisperse test aerosol by using various well-defined globular proteins in the size range between 3 and 16 nm. The monodispersivity of the so generated test aerosol is ensured by the inherent uniformity of the protein particles, simplifying the experimental set-up dramatically and allowing an independent characterisation of the sizing performance of various nDMAs. Size spectra obtained with two Vienna-type nDMAs Citation18 Citation23, two type-identical commercially available nDMAs (GEMMA, TSI Inc.) and a GEMMA prototype containing a nDMA Citation4 were analysed according to repeatability, comparability and resolution of the size spectra. Implications for the routine application of nDMAs in the analysis of nanoparticles of biological, inorganic or polymeric nature are discussed.
2. Materials and methods
2.1. Proteins
Protein stock solutions of about 1 mg/mL were prepared from commercial protein powders (Sigma-Aldrich) with a 20 mM ammonium acetate buffer (pH 6.0–6.7). Because of the negligible salt concentration of the protein samples, it was possible to analyse in buffer diluted solutions without further purification. However, in cases where involatile salt residues are observed and thus could influence the measured size of the protein particles, protein solutions were purified with Microspin (GE Healthcare) or with Microcon Centrifugal Devices (Millipore) until salt concentrations below 1 µg/mL were reached. The concentration of the different proteins was adjusted to the level that mainly monomer (M) and no significant dimeric (2M) signals were detected. The typical concentration was 20 µg/mL for proteins with a molecular mass >20 kDa and 5 µg/mL for proteins with a molecular mass <20 kDa. Insulin (5.7 kDa), ubiquitin (8.6 kDa), cytochrome C (12.3 kDa), myoglobin (17.6 kDa), carbonic anhydrase (29.0 kDa), ovalbumin (44.6 kDa), avidin (64.0 kDa), bovine serum albumin (66.4 kDa), native enolase dimer (93.4 kDa), fibrinogen (339 kDa), ferritin (483 kDa) and thyroglobulin (660 kDa) solutions were electrosprayed to produce monodisperse aerosol used in this study.
2.2. Nano-electrospray (N-ES instrument)
The N-ES source (Mod. 3480, TSI Inc) was used for the aerosolization of the protein samples. For this instrument and above named solvent system, we found that a stable cone-jet mode operation was obtained at 2 kV and 0.1-0.3 L/min CO2 (99.995%, Air Liquide N) and 1-1.5 L/min compressed air (99.999% synthetic air, Air Liquide) Citation17. A pressure difference of 4 psi between sample and spray chamber was applied, which resulted in a sample solution flow of 67 nL/min through the fused silica capillary (25 µm inner diameter and 160 µm outer diameter, uncoated; supplied by TSI Inc). The primary generated droplet size, which is determined by the conductivity of the solvent system and the sample flow through the capillary as well as the inner diameter of the capillary was 150 nm, corresponding to a flow of 2 × 1011 droplets per minute or about 1.7 × 108 droplets per/cm3. The N-ES source generates highly positively charged droplets, which are then subsequently neutralised in bipolar ion environment obtained with a Po-210 α-radiation source (TSI Inc). This process results in mainly singly negatively and positively charged airborne molecules and nanoparticles Citation8 Citation9. N-ES settings, buffer concentrations, and solution conductivity were identical for all instruments used in this study.
2.3. Nano differential mobility analysers
2.3.1. Gas phase electrophoretic macromolecule mobility analyser (GEMMA and GEMMA Prototype)
The commercial GEMMA system, used at the Vienna University of Technology (GEMMA TU Vienna) and University of California Los Angeles (GEMMA UCLA) consists of a nano-electrospray (nES) unit, a nDMA and an ultrafine condensation particle counter (CPC) as detector (all devices from TSI Inc). The operating particle size range of this instrument combination is between 3 nm (limited by the particle detection device, CPC) and 65 nm (the upper scan limit of the nDMA when using maximum sheath flow for maximum nDMA resolution). Both GEMMA systems were operated with negative high voltage at the central nDMA electrode, thus separating the positively charged fraction of the generated singly charged ions/particles. The detection limit of the system (nDMA coupled to the CPC) in terms of particle concentration is in the order of one singly charged particle/cm3. However, due to the limited charging efficiency in the N-ES source, concentrations of at least 104 protein particles/cm3 are necessary to obtain appropriate particle count statistics across the whole selected sizing range within a reasonable time (120 s/scan). Ten scans were averaged for each GEMMA spectrum presented in this paper. Both GEMMA systems (TU Vienna and UCLA) were operated under similar settings concerning sheath gas flow, scan time and size range.
Details (operation conditions and construction details) of the GEMMA prototype at the University of Vienna (GEMMA Protoype) are given in and in detail in a previous published paper Citation4.
Table 1. Properties and operating parameters of the evaluated nDMAs.
2.3.2. Parallel nano-differential mobility analysing system (PDMA)
The PDMA system is an advanced in-house development (Faculty of Physics, University of Vienna) combined with the above mentioned N-ES source and with an electrical ion/particle detection device working on the Faraday cup (FC) principle. Parallel to the scanning nDMA an identical separation nDMA is simultaneously operated for sampling of the selected nanoparticles, hence the acronym PDMA Citation24. Recent development extended the lower sizing limit of the PDMA system down to 0.7 nm. The applied detection method needs a certain minimum number of charged species (concentration) in order to achieve measurable electrical signal. The detection limit of this nDMA/FC system is currently in the order of 103 charged particles/cm3 to ensure good counting statistics.
High voltage of positive polarity was applied to the central electrode, thus analyzing the negatively charged particle fraction. The PDMA was operated under scanning conditions to yield a particle size spectrum from 1 to 20 nm within 120 s. Ten spectra were averaged for the presented data of each protein sample.
2.3.3. Long nano-differential mobility analyser (L-nDMA)
The L-nDMA is also an in-house (Faculty of Physics, University of Vienna) constructed nDMA coupled with the above described N-ES source. The difference to the PDMA is a ten-fold increased separation length, namely 150 mm (see ), which results in a different sizing range, starting from 2 nm up to 200 nm. The detection system of the L-nDMA is also based on the FC principle. This system was used with identical scanning rate (2 s/channel) as the PDMA and all GEMMA systems. Ten spectra of each protein were averaged for the final data analysis.
Figure 1. Operating scheme of a nDMA. HV–High Voltage–scanned from 0 to 10 kv during mobility analysis, R1–Inner Radius of the central electrode, R2–Outer Radius of the central electrode.
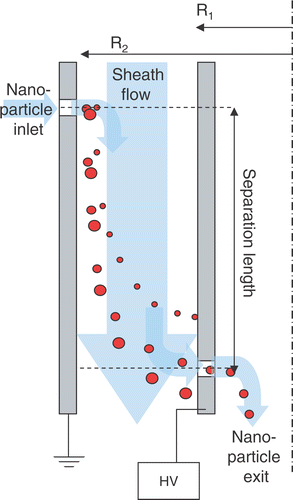
Properties and operation parameters which are important for the subsequent comparison and discussion of the results, are summarized in for all here described nDMAs.
2.4. Performance and resolution of n-DMAs
The principle and features of a DMA has been described extensively in literature Citation1 Citation3 Citation5 Citation23, so only key aspects of this technique are briefly summarized here, which are relevant for this investigation. Electrical ion mobility is defined by equation (Equation1) and is the ratio between electrostatic forces and friction that leads to constant particle movement when facing a certain electrical field. The electrical mobility can also be expressed in terms of design and operation parameters of the DMA () and leads as a consequence to only one particular size fraction of ions/particles that is analysed by the DMA at certain sheath gas and voltage settings (equation Equation2). Because the number of charges of the analysed ions/particles is determined by the neutralization step via the Po-210 source Citation8 Citation9, a size spectrum can be obtained by scanning through all size fractions by varying the voltage of the central electrode and by incorporating the charging probability of the analysed size fractions.
i | = |
Number of charges |
Z | = |
Electrical mobility diameter |
R 1, R 2 | = |
Inner and outer radius of the DMA electrode |
DP | = |
Particle/ion diameter, exiting the DMA for a given voltage |
Qsh | = |
Sheath gas flow |
L | = |
Separation length of the DMA |
C(DP ) | = |
Cunningham slip correction factor (see equation (Equation3)) |
λ | = |
Mean free path of the surrounding gas molecules: 66 nm at 20°C and 101 kPa |
The resolution of a DMA is influenced by the DMA geometry, particle/ion diffusion and physical properties of the sheath gas. (Equations Equation4 to 6) describe the relationship between the resolution of a DMA and conditions in the separation path and particle/ion properties. A detailed discussion about the DMA transfer function can be found in literature Citation5 Citation23 Citation25 Citation26.
ΔV | = |
Half width of the in voltage space normalized peak of a monodisperse particle/ion |
Vp | = |
Voltage setting of the peak center of a monodisperse particle/ion |
G | = |
Constant factor from theory Citation5 which depends on geometry, usually very close to unity |
Pe | = |
Peclet number (5) |
b | = |
Device parameter (6) |
D | = |
Diffusion coefficient of the particle/ion in question |
As can be seen from (equations Equation4 to 6), several parameters contribute to a DMA performance. Due to particle/ion diffusion, which decreases the resolution and leads to particle losses, transit time through the DMA has to be minimized, which is especially important for particles or ions below a size of 30 nm. This leads to the conclusion that a DMA should be operated with highest possible sheath gas flow (see equation Equation5). To decrease the separation path, the separation length L of the DMA (inlet into the DMA and exit point in the central electrode) has to be minimized. This leads as a consequence to a narrower annular width (R 2 minus R 1) within the DMA minimizing the term b + b −1 in equation (Equation5), resulting in higher voltages which have to be applied to a DMA (). Chen and Pai Citation27 has found from simulations that the geometry of particle/ion inlet and outlet slits has also a substantial influence on establishing a homogenous electrical field and a turbulence free zone between the inlet and outlet slits. A comparative study of the of the broadening of the transfer function of three practically identically constructed DMAs Citation28 showed, that even minor differences in the inlet section for sheath gas flow lead to a different performance of the DMAs concerning resolution. The effectiveness of electrophoretic mobility analysis of nanoparticles/ions paved the way to further DMA prototypes in which attempts are made to optimize the transfer function of the DMA in producing turbulence free flows even with extremely high sheath flow rates Citation29, or as alternative for example, to scan the sheath flow instead of the voltage of the central electrode or to apply a DMA with variable separation length Citation30 Citation31.
2.5 Sizing accuracy of nDMAs
The obtained raw size data were first normalized to the maximum height of the analyte peak and then the full width at half maximum (FWHM) was determined (see ). The count mean diameter (CMD) of the monomer peak was calculated for all size spectra according to equation (Equation7). Subsequently all CMDs corresponding to one protein sample analysed with one nDMA instrument were averaged to give one data point (used in ). The standard deviation of each CMD was calculated to obtain a value that reflects the repeatability of the nDMA analysis on each instrument.
C | = |
Count mean diameter of the monomer spectrum
|
b, e | = |
Integration borders of the Peak |
Ii | = |
Signal intensity at the size channel i |
Di | = |
Mobility diameter of the size channel i |
3. Results and discussion
The size spectra of the protein ovalbumin (same sample solution) obtained with two different nDMAs are shown in . A shift of about 1 nm between the spectra can be observed, although all parameters necessary to calculate a size spectrum from the raw data, such as gas properties, charging probability of the particles, geometrical parameters, sheath flow rate, are precisely known and implemented in the analysers software, Citation3 Citation18 Citation23. The observed differences in the size for identical protein particles has been found for al five nDMA systems (see ). As can be seen, the obtained CMDs are within a certain size interval, with a spread of up to 15% related to average particle size obtained by all instruments for all measured proteins. This difference between the investigated nDMAs becomes even more significant, as the repeatability of the position of the CMD for each protein particle/ion was just of the order of ±0.1% within a series of ten subsequent gathered size spectra obtained with one randomly chosen nDMA. This is about 100 times smaller than the spread of measured CMD values.
It is noticeable that the relationship between molecular mass and the CMD of the analysed globular proteins exhibits an excellent correlation coefficient (R 2 ≥ 0.98) for each instrument.
The relative (normalised to the CMD value) FWHM of measured peaks are similar for all investigated instruments and typically below 6% for proteins with a molecular mass >30 kDa except for the L-nDMA showing the relative FWHM in this molecular mass range of about 8%. This is a result of the longer separation distance connected with increased peak broadening by diffusion Citation23. This effect becomes dominating for measurements of species with a molecular mass <30 kDa, which corresponds to 5 nm diameter. For such small particles, the diffusion-driven broadening leads to relative FWHM of the order of 10% for smallest investigated proteins for all DMAs but the L-nDMA, where values of about 15% were found.
The observed size differences between the investigated instruments may result from various sources such as differences in particle/ion detection method, as well as the voltage scanning algorithm which recovers the actual size distribution from raw data Citation18. The L-nDMA and PDMA systems use for particle/ion detection a FC device, thus they measure directly and for practical reasons instantaneously the presence of a charged nanoparticle or ion. The three GEMMA systems use a CPC for the charged particle/ion detection. This method is known to deliver somewhat varying peak shape depending on the nDMA scan procedure and the CPC detection event Citation32. Whatever the reasons for the differences in the determined size values found in this study are, it is obvious that the use of calibrants of known size, molecular mass and high structural homogeneity (monodisperse) are absolutely necessary for each individual instrument even in case of identically constructed devices. The use of calibration compounds such as proteins, polymers or inorganic particles, are indispensable for the precise functioning of any nDMA system, especially in case of the analytical task of molecular mass determination (the nDMA system is used as ‘mass spectrometer’ at ambient pressure Citation4 Citation15) or in which conclusions about size, changes of size caused by agglomeration, denaturation, degradation or the formation of complexes of certain analytes have to be drawn.
4. Conclusions
The repeatability (±0.1%) of the measured size values in one series of ten subsequent collected size spectra with each nDMA shows evidently the ability of these instruments for the characterisation and analysis of nano-sized biological, inorganic and polymeric particles. But, the fact that all nDMA systems even though operated under optimal and nearly identical working conditions consistently show a sizing disagreement indicates that not all parameters which influence the size analysis can be controlled to a level at which sizing would agree to 5% or better. This leads to the final conclusion that for analysis of nanoparticles, macromolecules, proteins and polymers, a calibration of each individual nDMA system with well-defined size or molecular mass standards is inevitable.
Acknowledgements
WWS thanks the Austrian Science Foundation (FWF) for partial financial support of this research (grant P16185-N02). JAL acknowledges support from the W. M. Keck Foundation, UCLA Jonsson Comprehensive Cancer Center, the U. S. Department of Energy for funding of the UCLA-DOE Institute for Genomics and Proteomics, and the U. S. National Institutes of Health (RR 20004).
References
- Flagan , RC . 1998 . History of electrical aerosol measurements . Aerosol Sci. Technol. , 28 : 301
- Kittelson , DB . 1998 . Engines and nanoparticles: A review . J. Aerosol Sci. , 29 : 575
- Chen , D-R , Pui , DYH , Hummes , D , Fissan , H , Quant , FR and Sem , GJ . 1998 . Design and evaluation of a nanometer aerosol differential mobility analyser . J. Aerosol Sci. , 29 : 497
- Bacher , G , Szymanski , WW , Kaufman , SL , Zöllner , P , Blaas , D and Allmaier , G . 2001 . Charge-reduced nano electrospray ionization combined with differential mobility analysis of peptides, proteins, glycoproteins, noncovalent protein complexes and viruses . J. Mass Spectrom. , 36 : 1038
- Mora , JFde la . 1998 . Differential mobility analysis of molecular ions and nanometer particles . TrAC , 17 : 328
- Wilm , M and Mann , M . 1996 . Analytical properties of the nanoelectrospray ion source . Anal. Chem , 68 : 1
- Juraschek , R , Dülcks , T and Karas , M . 1999 . Nanoelectrospray-More than just a minimized-flow electrospray ion source . J. Am. Soc. Mass Spectrom. , 10 : 300
- Reischl , GP , Makela , JM , Karch , R and Necid , J . 1996 . Bipolar charging of ultrafine particles in the size range below 10 nm . J. Aerosol Sci. , 27 : 931
- Fuchs , NA . 1963 . On the stationary charge distribution on aerosol particles in bipolar ionic atmosphere . Geofis. Pura Appl , 56 : 185
- Hussin , A , Scheibel , HG , Backer , KH and Porstendorfer , J . 1983 . Bipolar diffusion charging of aerosol particles—I.Experimental results within the diameter range 4–30 nm . J. Aerosol Sci. , 14 : 671
- Hogan , CJ , Kettleson , EM , Ramaswami , B , Chen , D-R and Biswas , P . 2006 . Charge reduced electrospray size spectrometry of mega- and gigadalton complexes: Whole viruses and virus fragments . Anal. Chem. , 28 : 844
- Mouradian , S , Skogen , JW , Dorman , FD , Zarrin , F , Kaufman , SL and Smith , LM . 1997 . DNA Analysis using an electrospray scanning mobility particle sizer . Anal. Chem. , 69 : 919
- Loo , JA , Berhane , B , Kaddis , CS , Wooding , KM , Xie , Y , Kaufman , SL and Chernushevich , IV . 2005 . Electrospray ionization mass spectrometry and ion mobility analysis of the 20S Proteasome complex . J. Am. Soc. Mass Spectrom. , 16 : 998
- Saucy , DA , Ude , S , Lenggoro , IW and Mora , JFde la . 2004 . Mass analysis of water-soluble polymers by mobility measurement of charge-reduced ions generated by electrosprays . Analytical Chemistry , 76 : 1045
- Ku , BK and Mora , JFde la . 2004 . Mass distribution measurement of water-insoluble polymers by charge-reduced electrospray mobility analysis . Anal. Chem. , 76 : 814
- Müller , R , Laschober , C , Szymanski , WW and Allmaier , G . 2007 . Determination of molecular weight, particle size and density of high number generation PAMAM dendrimers using MALDI-TOF-MS and nES-GEMMA . Macromolecules , 40 : 5599
- Laschober , C , Kaufman , SL , Reischl , G , Allmaier , G and Szymanski , WW . 2006 . Comparison between an unipolar corona charger and a Polonium-based bipolar neutralizer for the analysis of nanosized particles and biopolymers . J. Nanosci. Nanotech. , 6 : 1474
- Reischl , GP , Makela , JM and Necid , J . 1997 . Performance of a Vienna-Type nano-DMA at 1.2-20 nm . Aerosol Sci. Technol. , 27 : 651
- Brimili , W , Stratmann , F , Wiedensohler , A , Covert , D , Russell , LM and Berg , O . 1998 . Determination of differential mobility analyser transfer function using identical instruments in series . Aerosol Sci. Technol. , 27 : 215
- Fissan , H , Hummes , D , Stratmann , F , Buscher , P , Neumann , S , Pui , DYH and Chen , D-R . 1996 . Experimental comparison of four differential mobility analysers for nanometer aerosol measurements . Aerosol Sci. Technol. , 24 : 1
- Fissan , H , Pöcher , A , Neumann , S , Boulaud , D and Pourpix , M . 1998 . Analytical and empirical transfer functions of a simplified spectromètre de mobilité . J. Aerosol Sci. , 29 : 289
- Hummes , D , Stratmann , F , Neumann , S and Fissan , H . 1996 . Experimetnal determination of the transfer function of a differential mobility analyser (DMA) in the nanometer size range . Part. and Part. Sys. Charact. , 13 : 327
- Reischl , GP . 1991 . Measurement of ambient aerosols by the differential mobility analyser method: Concepts and realization criteria for the size range between 2 and 500 nm . Aerosol Sci. Technol. , 14 : 5
- 2007 . Austrian Patent No. AT 502 207 A1 (W.W. Szymanski, G. Reischl, C. Laschober, and G. Allmaier)
- Stolzenburg , MR . 1988 . An Ultrafine Aerosol Size Distribution Measuring System , Minneapolis, MN : University of Minnesota .
- Reischl , GP . 1991 . The relationship of input and output aerosol characteristics for an ideal differential mobility analyser particle standard . J. Aerosol Sci. , 22 : 297
- Chen , D-R and Pui , DYH . 1997 . Numerical modeling of the performance of differential mobility analysers for nanometer aerosol measurements . J. Aerosol Sci. , 28 : 985
- Karlsson , MNA and Martinsson , BG . 2003 . Method to measure and predict the transfer function size dependence of individual DMAs . J. Aerosol Sci. , 34 : 603
- Rosser , S and Mora , JFde la . 2005 . Vienna-type DMA of high resolution and high flow rate . Aerosol Sci. Technol. , 39 : 1191
- Seol , KS , Yaburnoto , J and Takeuchi , K . 2002 . A differential mobility analyser with adjustable column length for wide particle size range measurements . Journal of Aerosol Science , 33 : 1481
- Collins , DR , Nenes , A , Flagan , RC and Seinfeld , JH . 2000 . The scanning flow DMA . J. Aerosol Sci. , 31 : 1129
- Russell , LM , Flagan , RC and Seinfeld , JH . 1995 . Asymetric instrument response resulting from mixing effects in accelerated DMA-CPC measurements . Aerosol Sci. Technol. , 23 : 491