Abstract
Gel-derived TiO2–SiO2 and N–TiO2–SiO2 mixed oxides with the TiO2 : SiO2 weight ratio of 95 : 5 are synthesised and employed for phenol removal under UVA light and natural sunlight in this study. Both SiO2 and N are interestingly found to improve the specific surface area of resulting catalysts up to 169 m2 g−1. Meanwhile, only N is observed to shift significantly the light absorption of derived catalyst to visible range. Optimisation between specific surface area and crystallinity in TiO2–SiO2 mixed oxide is found to give rise to the superior photoactivity of TiO2–SiO2 catalyst in comparison with TiO2 counterpart. Under natural sunlight in Hochiminh City on October 2007, N–TiO2–SiO2 presents the outstanding photoactivity towards decomposition of 10 ppm phenol aqueous solution with the efficiency up to 100% as compared to those of 65 and 48% for bare TiO2–SiO2 and bare TiO2, respectively.
1. Introduction
Since the first exploration of Fujishima and Honda on TiO2 for photoelectrolysis of water to H2 Citation1, TiO2 material has been intensively and extensively studied in various kinds of fields owing to its miracle properties Citation2. In order to enhance the activity of TiO2 catalyst, much attention has been paid by many research groups to the increase in its specific surface area by atomic mixing of SiO2 with TiO2 Citation3–7. Nevertheless, as SiO2 is inserted in TiO2 lattice, the improvement of specific surface area of the resulting catalyst is also accompanied by the broadening of its bandgap energy Citation4. As a consequence, TiO2–SiO2 mixed oxide inherently absorbs much more UV light than visible counterpart compared to bare TiO2.
Although nitrogen-doped TiO2 (N–TiO2) catalyst has been intensively studied for the absorption of visible light Citation8–12, nitrogen-doped TiO2–SiO2 (N–TiO2–SiO2) catalyst has not been much explored. In this present research, the N–TiO2–SiO2 mixed oxide is synthesised using the sol–gel method. For simultaneous improvement of the specific surface area and crystallinity of N–TiO2–SiO2 catalyst, hydrothermal treatment is also employed right after the sol–gel process. The derived catalysts are characterised by using nitrogen adsorption for BET specific surface area, X-ray diffraction for crystallinity, UV-vis spectroscopy for light absorption and field-emission scanning electron microscopy (FE-SEM) for morphology. The photoactivities of catalysts are tested in the decomposition reaction of phenol in aqueous solution under UVA light and natural sunlight irradiation.
2. Experimental
2.1. Catalyst preparation
shows the schematic diagram of the preparation of TiO2–SiO2 mixed oxide. Three solutions were first prepared : the first solution denoted as S1 was prepared by mixing solvents (a mixture of ethanol and iso-propanol with the volume ratio = 1 : 1) with water and HCl; the second one denoted as S2 included the solvents, water and tetraethyl orthosilicate (TEOS, Merck), which was strongly mixed in a beaker; the third solution denoted as S3 was a mixture of the solvents and titanium(IV) isopropoxide (TTIP, Merck). The volume of the solvents in S1, S2 and S3 were 120, 60 and 60 mL, respectively. The corresponding amount of TEOS and TTIP were employed to obtain the final weight ratio of TiO2 : SiO2 of 95 : 5. The molar ratios of H2O : TEOS in S2 and H2O : (TEOS + TTIP) in this experiment were 2 : 1 and 4 : 1, respectively. The corresponding amount of 35% HCl solution was used in S1 to control the pH of this solution at 1. The above three solutions were then mixed and refluxed at 80°C for 1 h under vigorous stirring (ca 1500 rpm). The obtained sol–gel solution was thereafter hydrothermally treated in a lab-made autoclave at 150°C for 10 h. After that, the solvents were removed at 50°C using a rotary vacuum system. The aerogel was later dried in an oven at 105°C for 2 h and then calcined at different temperatures.
For the synthesis of N–TiO2–SiO2 catalyst, corresponding amount of urea was dissolved in the S1 solution to get the final urea : (TiO2 + SiO2) molar ratio of 1 : 1. Hydrochloride acid in the S1 solution was replaced by nitric acid.
In both TiO2–SiO2 and N–TiO2–SiO2 mixed oxides, the TiO2 : SiO2 weight ratio was controlled at 95 : 5. Calcination of catalyst was carried out at temperatures of 350°C, 400°C, 450°C, 500°C, 550°C, 600°C, 700°C and 900°C.
2.2. Catalyst characterisation and photocatalytic decomposition of phenol
The crystallinity of catalyst was measured by using X-ray diffraction (Rikagu, Cu-Kα). The BET specific surface area of catalyst was determined by nitrogen adsorption at 77 K (Chembet 3000). The bandgap energy of catalyst was estimated by using UV-vis diffuse reflectance spectroscopy (Jasco 500). The catalyst particle size and morphology were measured by using FE-SEM (Hitachi S-4800).
Photocatalytic decomposition of phenol was carried out in a 1 L reactor containing 300 mL of 10 ppm phenol aqueous solution and 0.15 g of catalyst. The reaction solution was well mixed by magnetic bar at c. 200 rpm. The outer light source for the reaction consisted of two UVA lamps with the total capacity of 30 W and the peak of light spectrum at 365 nm. Before turning on the lamps, the reaction solution was well mixed for 30 min in dark to ensure the equilibrium processes of adsorption and desorption of phenol on the surface of catalyst. For the analysis of phenol concentration after each period of 20 min, 5 mL of solution was sampled, centrifuged (c. 6000 rpm in 10 min) and filtered (using a 0.45 µm filter, Merck). The phenol concentration was determined by using UV-vis spectroscopy (Cary Varian 50). The reactor temperature was maintained at ∼30°C using a water cooling system. For the experiment under natural sunlight, the reactor was left outdoor in a sunny day from 11:00 am to 13:00 pm in Hochiminh city. The sunlight intensity during the experiment time was measured by using a lumen metre (Lux 5924, Hana).
3. Results and discussion
presents the specific surface areas of different catalysts. It is found that lab-made TiO2 prepared with sol–gel method and hydrothermal treatment shows very high BET specific surface area as compared with commercial TiO2 (P25). As SiO2 is atomically mixed with TiO2, the specific surface area of resulting TiO2–SiO2 mixed oxide is significantly increased up to 143.5 m2 g−1. This value is around three times higher than that of bare TiO2 catalyst. When nitrogen is doped in the TiO2–SiO2 material, the resulting N–TiO2–SiO2 is then observed to significantly increase up to 229.8 m2 g−1 on N–TiO2–SiO2-400 catalyst. Calcination temperature is found to strongly influence the specific surface area of N–TiO2–SiO2 catalyst as shown in . At low-calcination temperatures, from 350°C to 450°C, the specific surface area of N–TiO2–SiO2 is observed to increase and then decrease with the maximum value of 229.8 m2 g−1 at 400°C. This result may be ascribed to the contribution of remaining organic and inorganic compounds at low-calcination temperatures to the S BET of the resulting catalysts. Calcination at 400°C may maximise the S BET of both remaining organic and inorganic compounds in the N–TiO2–SiO2 catalyst. As calcination temperature increases from 450°C to 550°C, the S BET of N–TiO2–SiO2 is observed to again increase and then reach the highest value of 165.7 m2 g−1 at 550°C. At these calcination temperatures, the remaining organic compounds in the catalyst could be completely removed, which in turn significantly produces a large number of micro-pores in the catalyst. As a consequence, the S BET of catalyst calcined at 450–550°C is observed to increase. As calcination temperature is increased from 550°C to 900°C, the S BET of N–TiO2–SiO2 is substantially decreased. It is well known that low-calcination temperature is followed by low crystallinity of the derived catalyst Citation13, which could be consistently observed in . The lower the crystallinity, the higher is the BET specific surface area of catalyst Citation13. When calcination temperature is increased to over 450°C for complete removal of organic compounds, the S BET of catalyst is certainly improved. However, as the calcination temperature is too high, sintering effect is the main factor to increase the catalyst crystallinity, and therefore decrease its specific surface area Citation13.
presents the FE-SEM morphology of different catalysts. Bare TiO2 catalyst depicts the largest particle size of around 30 nm. Meanwhile, when TiO2 is doped with SiO2 and nitrogen, the particle sizes of resulting catalysts are observed to significantly decrease as shown in . The particle sizes of TiO2–SiO2 and N–TiO2–SiO2 catalysts are estimated around 13 and 8 nm, respectively.
X-ray diffraction patterns of nitrogen-doped TiO2–SiO2 mixed oxides calcined at different temperatures are shown in . When calcination temperature is increased from 350°C to 900°C, the crystallinity of the catalyst is significantly improved. shows the X-ray diffraction patterns of TiO2–SiO2 and N–TiO2–SiO2 mixed oxides in comparison with those of bare lab-made TiO2 and TiO2-P25. Lab-made catalysts present only anatase phase, whereas both anatase and rutile phase are observed on TiO2-P25. Crystal phase transformation is not observed on either bare TiO2–SiO2 or N–TiO2–SiO2. Meanwhile, on increasing calcination temperature to 900°C, phase transformation from anatase to rutile is found on TiO2–P25 Citation13. Thermal stability of TiO2–SiO2 and N–TiO2–SiO2 could result from the hydrothermal treatment process of sol–gel solution.
Figure 3. (A) XRD patterns of N–TiO2–SiO2 calcined at different temperatures: (a) 350°C, (b) 400°C, (c) 450°C, (d) 500°C, (e) 550°C, (f) 600°C, (g) 700°C, (h) 900°C and (j) TiO2 (P25-Degussa); (B) XRD patterns of different catalyst: (a) N–TiO2–SiO2–550, (b) TiO2–SiO2–550, (c) TiO2–550 and (d) TiO2 (P25-Degussa).
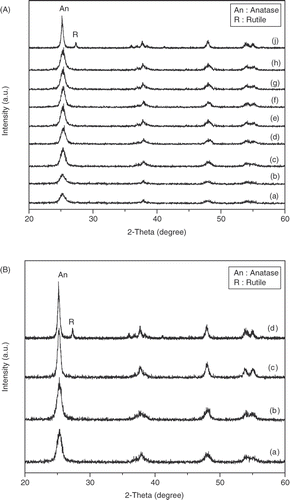
Table 1. BET specific surface areas of different catalysts.
UV-vis DRS of N–TiO2–SiO2 mixed oxide calcined at different temperatures is shown in . At low-calcination temperature, N–TiO2–SiO2 presents strong absorption of visible light. This result could be explained by the trace residue of organic compounds after calcination of catalyst at low temperature. The colours of catalysts calcined at 350°C, 400°C and 450°C are observed by unaided eyes as brown, pale yellow and white, respectively. As increasing calcination temperature up to 500°C is well agreed to completely remove organic compounds, N–TiO2–SiO2 is still observed to have strong absorption of visible light as compared to bare TiO2–SiO2. This phenomenon is ascribed to the substitution of the oxygen atom in TiO2–SiO2 by its nitrogen counterpart Citation8,Citation9, which results in the decrease in bandgap energy of the derived N–TiO2–SiO2 catalyst. As a consequence, the UV-vis spectrum of N–TiO2–SiO2 shifts to the visible range as shown in .
Photocatalytic activities of different catalysts towards phenol removal under UVA light are shown in . As N–TiO2–SiO2 catalyst is calcinced at temperatures lower than 450°C, its photoactivity efficiency is too low and comparable at ∼20%. On increasing calcination temperature up to 450°C, phenol removal efficiency of catalyst is significantly improved from c. 20% to over 40% as presented in . The photoactivity of catalyst is then observed to increase to the maximum value at calcination temperature of 600°C. However, as the catalyst is calcined at temperature more than 600°C, its phenol removal efficiency is substantially reduced.
Table 2. Phenol removal efficiency of different catalysts under UVA irradiation.
Although N–TiO2–SiO2-350 and N–TiO2–SiO2-400 catalysts present superior specific surface area and visible light absorption as shown in and , their crystallinity is too low because of a lot of organic traces in the catalyst. As a consequence, N–TiO2–SiO2-350 and N–TiO2–SiO2-400 catalysts display too low photoactivity towards phenol removal, which is ascribed to the large presence of recombination centres of excited electrons and holes in their lattices Citation14. As calcination temperature is increased, the regularity of catalyst lattice is gradually improved as presented in . Nevertheless, the BET specific surface area and visible light absorption of the catalyst are largely weighed off ( and ). Accordingly, the optimum value of calcination temperature of catalyst inherently exists to obtain the best catalyst, that is, N–TiO2–SiO2-600 with the maximum phenol removal efficiency of 56.03%. It is also found in this study that the N–TiO2–SiO2 catalyst shows superior photoactivity towards phenol removal as compared to the bare TiO2–SiO2 counterpart under the same preparation condition.
depicts the phenol removal efficiency of different catalysts according to time under natural sunlight. It is interestingly found that N–TiO2–SiO2 presents superior photoactivity towards phenol removal as compared to bare TiO2 and TiO2–SiO2 catalysts. Although phenol removal efficiency of the N–TiO2–SiO2 catalyst is around 10% higher than that of the bare TiO2–SiO2 counterpart under UVA light irradiation, the photoactivity of the former towards phenol removal is up to 40% higher than that of the latter under natural sunlight irradiation. These results could be explained by the strongly visible absorption of N–TiO2–SiO2 in comparison with bare TiO2 and TiO2–SiO2 as shown in as well as the superior specific surface area of the N–TiO2–SiO2.
4. Conclusions
The N–TiO2–SiO2 photocatalyst with high specific surface area and strong visible light absorption is synthesised and characterised for decomposition of phenol in aqueous solution. SiO2 is found to significantly increase the specific surface area of the resulting TiO2–SiO2 mixed oxide. Meanwhile, the insertion of nitrogen in the TiO2–SiO2 lattice is observed to substantially shift the UV-vis spectroscopy of the derived N–TiO2–SiO2 to visible range. The high specific surface area up to 169 m2 g−1 and good crystallinity of N–TiO2–SiO2 could also be ascribed to the hydrothermal treatment method that was employed right after the sol–gel process. Owing to strong absorption of visible light, high specific surface area and good crystallinity of the N–TiO2–SiO2 catalyst, its photoactivity towards phenol removal is superior compared to those of bare TiO2–SiO2 and the bare TiO2 counterparts. The phenol removal efficiencies of N–TiO2–SiO2, TiO2–SiO2 and TiO2 catalysts under natural sunlight in Hochiminh City in October 2007 are 100, 65 and 48%, respectively. The result presents strongly the potential application of N–TiO2–SiO2 in environmental treatment under real sunlight.
Acknowledgements
The authors gratefully acknowledge financial support from the Ministry of Science and Technology through the Technological Incubation programme. We also thank for their kind suggestions and help colleagues from the Faculty of Environment, the Faculty of Materials Technology and the Faculty of Chemical Technology, Hochiminh City University of Technology.
References
- Fujishima , A and Honda , K . 1972 . Electrochemical photolysis of water at a semiconductor electrode . Nature , 238 : 37 – 38 .
- Fujishima , A , Hashimoto , K and Watanabe , T . 1999 . TiO2 Photocatalysis Fundamentals and Applications , Tokyo : BKC .
- Dutoit , DCM , Gobel , U , Schneider , M and Baiker , A . 1996 . Titania-silica mixed oxides V. Effect of sol-gel and drying conditions on surface properties . J. Catal. , 164 : 433 – 439 .
- Gao , X and Wachs , IE . 1999 . Titania-silica as catalysts: Molecular structural characteristics and physico-chemical properties . Catal. Today , 51 : 233 – 254 .
- Nguyen , T-V and Yang , O-B . 2003 . Photoresponse and AC impedance characterization of TiO2-SiO2 mixed oxide for photocatalytic water decomposition . Catal. Today , 87 : 69 – 75 .
- Nguyen , T-V , Choi , D-J and Yang , O-B . 2005 . Effect of chelating agents on the properties of TiO2-SiO2 mixed oxide for photocatalytic water decomposition . Res. Chem. Intermed. , 31 : 483 – 491 .
- Nguyen , T-V , Kim , S and Yang , O-B . 2004 . Water decomposition on TiO2-SiO2 and RuS2/TiO2-SiO2 photocatalysts: The effect of electronic characteristics . Catal. Commun. , 5 : 59 – 62 .
- Asahi , R , Morikawa , T , Ohwaki , T , Aoki , K and Taga , Y . 2001 . Visible-light photocatalysis in nitrogen-doped titanium oxides . Science , 293 : 269 – 271 .
- Nosaka , Y , Matsushita , M , Nishino , J and Nosaka , AY . 2005 . Nitrogen-doped titanium dioxide photocatalysts for visible response prepared by using organic compounds . Sci. Technol. Adv. Mater. , 6 : 143 – 148 .
- Yuan , J , Chen , M , Shi , J and Shangguan , W . 2006 . Preparation and photocatalytic characterization of nitrogen doped TiO2 photocatalyst . Int. J. Hydrogen Energy , 31 : 1326 – 1331 .
- Wang , Z , Cai , W , Hong , X , Zhao , X , Xu , F and Cai , C . 2005 . Photocatalytic degradation of phenol in aqueous nitrogen-doped TiO2 suspensions with various light sources . Appl. Catal. B: Environ. , 57 : 223 – 231 .
- Joung , S-K , Amemiya , T , Murabayashi , M and Itoh , K . 2006 . Relation between photocatalytic activity and preparation conditions for nitrogen-doped visible light-driven TiO2 photocatalysts . Appl. Catal. A: Gen. , 312 : 20 – 26 .
- Nguyen , T-V , Lee , H-C and Yang , O-B . 2006 . The effect of pre-thermal treatment of TiO2 nanoparticles on the performance of dye-sensitized solar cells . Sol. Energy Mater. Sol. Cells. , 90 : 967 – 981 .
- Litter , MI and Navío , JA . 1996 . Photocatalytic properties of iron-doped titania semiconductors . J. Photochem. Photobiol. A: Chem. , 98 : 171 – 181 .