Abstract
Microelectromechanical systems (MEMS) are devices that represent the integration of mechanical and electrical components in the micrometer regime. Self-assembled monolayers (SAMs) can be used to functionalise the surface of MEMS resonators in order to fabricate chemically specific mass sensing devices. The work carried out in this article uses atomic force microscopy (AFM) and X-ray photoemission spectroscopy (XPS) data to investigate the pH-dependent adsorption of citrate-passivated Au nanoparticles to amino-terminated Si3N4 surfaces. AFM, XPS and mass adsorption experiments, using ‘flap’ type resonators, show that the maximum adsorption of nanoparticles takes place at pH = 5. The mass adsorption data, obtained using amino functionalised ‘flap’ type MEMS resonators, shows maximum adsorption of the Au nanoparticles at pH = 5 which is in agreement with the AFM and XPS data, which demonstrates the potential of such a device as a pH responsive nanoparticle detector.
1. Introduction
Microelectromechanical systems (MEMS) devices are defined by the integration of electrical and mechanical components on the micron length scale Citation1. Examples of the use of MEMS devices include actuators Citation2,Citation3 and sensors Citation4–14. MEMS devices can be used as chemical sensors via mass detection Citation7,Citation14, whereby the resonant frequency of a MEMS resonator changes upon the adsorption of chemical species; the resultant change of resonant frequency is proportional to the mass adsorbed on the surface Citation4,Citation7,Citation15. Hence, the mass of the adsorbed species can be calculated using knowledge of the mass sensitivity of the device. Such devices operate on a similar principle to both quartz crystal microbalances (QCM) Citation16,Citation17 and surface acoustic wave (SAW) devices Citation18,Citation19. The species adsorbed on the surface of MEMS mass detection devices is dictated by the surface chemistry of the resonator with self-assembled monolayers (SAMs) having been employed to immobilise specific species to the MEMS sensors Citation5,Citation7–9,Citation12.
The aim of the research described in this article is to demonstrate the pH-dependent immobilisation of citrate-passivated Au nanoparticles to amino-functionalised Si3N4 surfaces with a view to transferring such surface chemistry to a MEMS resonator. Previous work has shown pH-dependent adsorption of citrate-passivated Au nanoparticles to aromatic amino-functionalised Au Citation20 and aliphatic amino-functionalised SiO2 Citation21 substrates. The investigation of the pH-dependent adsorption of citrate-passivated Au nanoparticles to aliphatic amino-functionalised Si3N4 substrates will provide a model system with which the pH-dependent adsorption of nanoparticles to MEMS devices can be tested. Atomic force microscopy (AFM) and X-ray photoemission spectroscopy (XPS) data will be used to determine the maximum pH at which citrate-passivated Au nanoparticles attach to −NH2-functionalised Si3N4 substrates. Thus, providing both (i) a reference of the pH-dependent adsorption of citrate-passivated Au nanoparticles to Si3N4 resonators modified with 3-aminopropyltrimethoxysilane (APTMS) SAMs, and (ii) a model system to show that such chemically modified microresonators can be used as mass sensors for nanoparticles ().
2. Results and discussion
AFM and XPS analysis have been used to monitor the pH-dependent adsorption of citrate-passivated nanoparticles on Si3N4 substrates modified by exposure to APTMS vapour. We have described the formation of thin films of APTMS by a vapour method onto Si3N4, previously Citation22. Here, we report the pH-dependent adsorption of citrate-passivated Au nanoparticles on these films by monitoring with AFM (surface coverage), XPS (elemental composition) and MEMS resonators (mass adsorption, see ). All three techniques reveal a peak adsorption at pH = 5.
2.1. Au nanoparticle adsorption studies
AFM images were obtained from Si3N4 substrates, modified with APTMS SAMs, after immersion for 2 h in aqueous solutions of citrate-passivated Au nanoparticles at five different values of pH (pH = 3, 4, 5, 6 and 7) (). A graph of nanoparticle surface coverage versus the pH is shown in (block circles).
Figure 2. AFM images and XPS Au 4f spectra of adsorption of citrate-passivated Au nanoparticles on APTMS-modified Si3N4 substrates at five different values of pH. The binding energies of both the Au 4f5/2 and Au 4f7/2 XPS peaks and peak fitting of the XPS spectra are also shown.
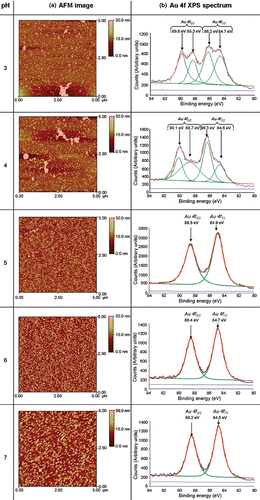
At pH values of 3 and 4 it can be seen that the occurrence of Au nanoparticle aggregates adsorbed on the surface is more pronounced than at pH = 5, 6 or 7 (). Presumably, the occurrence of a higher degree of nanoparticulate aggregates at pH values of 3 and 4, compared to pH values 5, 6 or 7, is due to a higher degree of protonation of the passivating citrate anions in solution, corresponding to a lower degree of electrostatic repulsion between the particles, leading to gold core fusion. The reduction of the number of repulsive negative charges on citrate-passivated Au nanoparticles at acidic pHs has previously been demonstrated by zeta potentiometry Citation21. The maximum adsorption of citrate-passivated Au nanoparticles occurs at pH=5 ( and ), which is consistent with previous work which shows that optimal adsorption of citrate-passivated Au nanoparticles on −NH2 terminated SAMs on SiO2 substrates occurs at pH ∼ 5 Citation21.
Figure 3. Graph showing both particle density (measured by AFM) and Au/Si ratio (measured by XPS) vs. pH of citrate-passivated Au nanoparticles adsorbed onto APTMS-modified Si3N4 substrates.
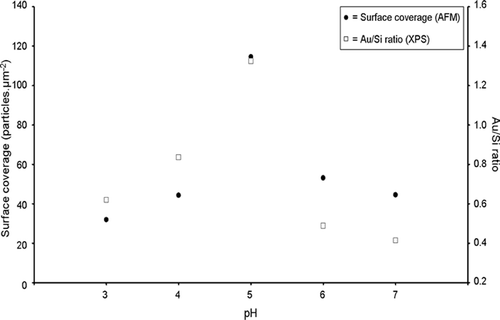
XPS spectra of APTMS-modified Si3N4 substrates were recorded after immersion in solutions of citrate-passivated Au nanoparticles over the pH range 3–7. The Au 4f spectra () and Si 2p spectra, shown in (see Appendix), were used to calculate the Au/Si ratio in order to determine the relative change of nanoparticle adsorption. The Au/Si ratios were used to study the adsorption of Au nanoparticles using the Si substrate as an internal standard, assuming that the amount of Si present is constant. (open squares) shows that the maximum Au/Si ratio occurs at pH ∼ 5 which shows that the maximum nanoparticle adsorption occurs at pH ∼ 5. This result concurs with the AFM data ( (closed circles)).
When nanoparticle adsorption is carried out at pH = 5, 6 and 7 the Au 4f spectra appear as two distinct peaks (the Au 4f5/2 and Au 4f7/2 peaks). However, when nanoparticle adsorption is carried out at pH = 3 and 4 the Au 4f peaks appear to split () and this coincides with the aggregation of Au nanoparticles (). The binding energy of the Au 4f7/2 for Au3+ occurs at 86.7 eV Citation23 and one of the split Au 4f7/2 peaks shifts towards this value. Therefore, this indicates that at pH = 3 and 4 the citrate-passivated Au nanoparticles are partially oxidised. Praharaj et al. Citation24 have observed that the addition of HCl to Au nanoparticles can lead to the oxidation of Au0 to Au3+ and HCl is used for the reduction of pH of the Au nanoparticle solution in this study. Therefore, the splitting of the Au 4f peaks by XPS is presumably due to the oxidation of the Au.
Both AFM and XPS measurements demonstrate that maximum Au nanoparticle adsorption to an APTMS thin film occurs at pH = 5 (). To rationalise this observation we need to consider the pK a of the surface-active components. The pK a of aliphatic amine groups is 8–11 in free solution Citation25; however, when attached to a surface the pK a decreases to 6–8 Citation26. The pK a of COOH groups in free solution is ∼4.8 Citation27 and we might expect a shift to lower pK a on the surface of the Au nanoparticles. Thus, at pH values of 3 and 4 few COOH groups will be deprotonated, hence, there will be little or no repulsive electrostatic forces existing between the particles, leading to aggregation of the nanoparticles (). Furthermore, the neutral Au nanoparticles do not lend themselves to electrostatic attachment to the positively charged APTMS SAMs (although hydrogen bonding will occur). The maximum adsorption of Au nanoparticles occurs at pH = 5, which is due to the best balance between the citrate-passivated nanoparticles being negatively charged and the amino surface being positively charged (). However, at pH = 6 and 7 nanoparticle adsorption reduces as the APTMS thin film becomes increasingly deprotonated at a rate greater than that at which the Au nanoparticles become negatively charged (). Therefore, the electrostatic attraction between the negatively charged Au nanoparticles and the APTMS surface decreases due to a less positively charged surface as the pH increases.
2.2. Mass adsorption measurements
Initial mass adsorption measurements were made by measuring the frequency shift of simple ‘flap’ type resonators ( and ) at three different pH values. These MEMS devices were fabricated using focused ion beam (FIB) microfabrication to cut a flap with three free sides from a membrane of silicon nitride, 500 nm thick. Each device measures approximately 200 × 150 µm2 and is designed to oscillate in ‘flap mode’ about an axis of rotation through the fixed side. The devices were excited by mounting them on a piezoelectric chip in an SEM, which was connected to a vibrometer. Careful tuning of the drive frequency of the piezoelectric crystal, coupled with real time observation under the SEM, allowed the resonant frequency to be found once the edge of the flap became blurred.
Figure 5. Graph showing the mass of citrate-passivated Au nanoparticles adsorbed on APTMS-modified Si3N4 ‘flap’ resonators vs. pH.
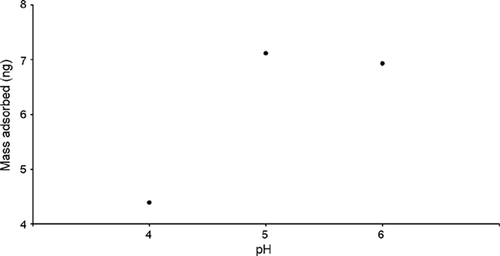
Table A1. The resonant frequencies of the ‘flap’ resonator before any modification (FR 1), after exposure of the resonator to APTMS vapour (FR 2) and then after subsequent immersion in citrate-passivated Au nanoparticles (FR 3). (The adsorbed mass, calculated from the frequency changes, is also shown).
The devices were calculated to have a frequency shift of 590 Hz/10−8 g using MathCAD software. Therefore, this value was used to calculate the masses adsorbed on the resonators once the frequency shift was determined. The results shown in indicate maximum nanoparticle adsorption at pH = 5, in agreement with the AFM and XPS data () though the difference in absorbance between pH = 5 and 6 is not as pronounced as that in .
3. Conclusions
The aim of the work presented in this article is to show that the pH-dependent adsorption of citrate-passivated Au nanoparticles on amino-functionalised Si3N4 resonators can be used as a model system for the development of MEMS nanoparticulate sensors (). AFM (), XPS () and mass detection, using chemically modified resonators (Figures and ), was used to monitor the pH-dependent adsorption of citrate-passivated Au nanoparticles on amino-functionalised Si3N4 surfaces. AFM images () reveal that the Au nanoparticles form aggregates on the APTMS surface at pH = 3 and 4. This observation is due to the loss of electrostatic repulsion between the particles as a result of decreased deprotonation of the COOH moieties on the nanoparticles at pH = 3 and 4 (). Maximum surface coverage of nanoparticles was observed at pH = 5 ( and (block circles)) when the balance between electrostatic repulsion between the Au nanoparticles and the degree of protonation of the APTMS surface is optimal (). At pH = 6 and 7 the degree of surface coverage ( and (block circles)) is less than at pH = 5, which is due to a decrease in surface protonation at higher values of pH compared to that at pH = 5 (). XPS analysis () was used to calculate the Au/Si ratio which, in agreement with the surface coverage calculated by AFM, shows maximum Au coverage at pH = 5 ( (open squares)). XPS also reveals splitting of both the Au 4f5/2 and Au 4f7/2 peaks, which is representative of oxidation of the Au nanoparticles. Such peak splitting was not observed when particle absorption was carried out at pH = 5, 6 or 7, which is consistent with the presence of discrete Au nanoparticles and little or no oxidation of the Au nanoparticles. The mass adsorption experiments, carried out using amino functionalised Si3N4 ‘flap’ resonators (), revealed maximum Au nanoparticle adsorption at pH = 5 (), which is in agreement with both the AFM and XPS data. However, the difference between nanoparticle adsorption at pH = 5 and 6 is not as pronounced for the mass adsorption experiments () as it is for the surface coverage obtained by AFM and the Au/Si ratio obtained by XPS ().
The pH-dependent adsorption of citrate-passivated Au nanoparticles to chemically modified microresonators with a high Q-factor Citation4 will be investigated. This will allow the fabrication of highly sensitive mass-detecting MEMS devices.
Acknowledgements
This work was supported by the Engineering and Physical Sciences Research Council (EPSRC) (Basic technology) under Grant No. GR/571514/01 and the EU STREP Nano 3-D (NMP4-CT-2005-01406).
References
- Maboudian , R . 1998 . Surface processes in MEMS technology . Surf. Sci. Rep. , 30 : 207 – 269 .
- Liew , LA , Saravanan , RA , Bright , VM , Dunn , ML , Daily , JW and Raj , R . 2003 . Processing and characterization of silicon carbon-nitride ceramics: application of electrical properties towards MEMS thermal actuators . Sens. Actu. A , 103 : 171 – 181 .
- Wang , W , Tatic-Lucic , S , Brown , WL and Vinci , R . 2008 . Design of a bidirectional MEMS actuator with high displacement resolution, large driving force and power-free latching . Microelec. Eng. , 85 : 587 – 598 .
- Boonliang , B , Prewett , PD , Hedley , J , Preece , J and Hamlett , CA . 2008 . A focused-ion-beam micro-paddle resonator for mass detection . J. Micromech. Microeng. , 18 : 015021 (4 pp)
- Choi , NJ , Lee , YS , Kwak , JH , Park , JS , Park , KB , Shin , KS , Park , HD , Kim , JC , Huh , JS and Lee , DD . 2005 . Chemical warfare agent sensor using MEMS structure and thick film fabrication method . Sens. Actu. B , 108 : 177 – 183 .
- Chun , DW , Hwang , KS , Eom , K , Lee , JH , Cha , BH , Lee , WY , Yoon , DS and Kim , TS . 2007 . Detection of the Au thin-layer in the Hz per picogram regime based on the microcantilevers . Sens. Actu. A , 135 : 857 – 862 .
- Goeders , KM , Colton , JS and Bottomley , LA . 2008 . Microcantilevers: sensing chemical interactions via mechanical motion . Chem. Rev. , 108 : 522 – 542 .
- Gong , JW , Chen , QF , Fei , WF and Seal , S . 2004 . Micromachined nanocrystalline SnO2 chemical gas sensors for electronic nose . Sens. Actu. B , 102 : 117 – 125 .
- Hauptmann , PR . 2006 . Selected examples of intelligent (micro) sensor systems: state-of-the-art and tendencies . Meas. Sci. & Tech. , 17 : 459 – 466 .
- Lavrik , NV , Sepaniak , MJ and Datskos , PG . 2004 . Cantilever transducers as a platform for chemical and biological sensors . Rev. Sci. Instrum. , 75 : 2229 – 2253 .
- Li , P and Li , XX . 2006 . A single-sided micromachined piezoresistive SiO2 cantilever sensor for ultra-sensitive detection of gaseous chemicals . J. Micromech. Microeng. , 16 : 2539 – 2546 .
- Meier , DC , Evju , JK , Boger , Z , Raman , B , Benkstein , KD , Martinez , CJ , Montgomery , CB and Semancik , S . 2007 . The potential for and challenges of detecting chemical hazards with temperature-programmed microsensors . Sens. Actu. B , 121 : 282 – 294 .
- Mlsna , TE , Cemalovic , S , Warburton , M , Hobson , ST , Mlsna , DA and Patel , SV . 2006 . Chemicapacitive microsensors for chemical warfare agent and toxic industrial chemical detection . Sens. Actu. B , 116 : 192 – 201 .
- Zhou , W , Khaliq , A , Tang , YJ , Ji , HF and Selmic , RR . 2005 . Simulation and design of piezoelectric microcantilever chemical sensors . Sens. Actu. A , 125 : 69 – 75 .
- Li , YC , Ho , MH , Hung , SJ , Chen , MH and Lu , MSC . 2006 . CMOS micromachined capacitive cantilevers for mass sensing . J. Micromech. Microeng. , 16 : 2659 – 2665 .
- Lazcka , O , Campo , FJD and Munoz , FX . 2007 . Pathogen detection: a perspective of traditional methods and biosensors . Biosens. Bioelec. , 22 : 1205 – 1217 .
- O'Sullivan , CK and Guilbault , GG . 1999 . Commercial quartz crystal microbalances - theory and applications . Biosens. Bioelec. , 14 : 663 – 670 .
- Dorozhkin , LM and Rozanov , IA . 2001 . Acoustic wave chemical sensors for gases . J. Anal. Chem. , 56 : 399 – 416 .
- Smith , JP and Hinson-Smith , V . 2006 . The new era of SAW devices . Anal. Chem. , 78 : 3505 – 3507 .
- Zhu , T , Fu , X , Mu , T , Wang , J and Liu , Z . 1999 . pH-Dependent adsorption of gold nanoparticles on p-aminothiophenol-modified gold substrates . Langmuir , 15 : 5197 – 5199 .
- Diegoli , S , Mendes , PM , Baguley , ER , Leigh , SJ , Iqbal , P , Garcia Diaz , YR , Begum , S , Critchley , K , Hammond , GD , Evans , SD , Attwood , D , Jones , IP and Preece , JA . 2006 . pH-Dependant gold nanoparticle self-organization on functionalised Si/SiO2 surfaces . J. Exp. Nanosci. , 1 : 333 – 353 .
- Hamlett , CAE , Critchley , K , Gorzny , M , Evans , SD , Prewett , PD and Preece , JA . 2008 . Vapour phase formation of amino functionalised Si3N4 surfaces . Surf. Sci. , 602 : 2724 – 2733 .
- Ertas , G , Demirok , UK and Suzer , S . 2005 . Enhanced peak separation in XPS with external biasing . Appl. Surf. Sci. , 249 : 12 – 15 .
- Praharaj , S , Panigrahi , S , Basu , S , Pande , S , Jana , S , Ghosh , SK and Pal , T . 2007 . Effect of bromide and chloride ions for the dissolution of colloidal gold . J. Photochem. Photobio. A , 187 : 196 – 201 .
- Patai , S . 1968 . The Chemistry of the Amino Group , London, , UK : Interscience Publishers .
- Wang , B , Oleschuk , RD and Horton , JH . 2005 . Chemical force titrations of amine- and sulfonic acid-modified poly(dimethylsiloxane) . Langmuir , 21 : 1290 – 1298 .
- Atkins , PW . 1998 . Physical Chemistry , 6th , Oxford, , UK : Oxford University Press .
- Frens , G . 1973 . Controlled nucleation for the regulation of the particle size in monodisperse gold suspensions . Nat. Phys. Sci. , 241 : 20 – 22 .
- Wei , ZQ , Wang , C and Bai , CL . 2000 . Surface imaging of fragile materials with hydrophilic atomic force microscope tips . Surf. Sci. , 467 : 185 – 190 .
4. Experimental
All chemicals were obtained from Aldrich unless stated.
4.1. Preparation of citrate-passivated Au nanoparticles
Aqueous solutions of citrate-passivated Au nanoparticles were prepared by the method described by Frens Citation28. A solution of chloroauric acid (0.01 g, 2.5 mmol in UHQ water) was heated under reflux for 2 h. Sodium citrate tribasic dihydrate (0.023 g, 0.15 mol) was added to the refluxing chloroauric acid solution and heating continued until there was no further colour change (colourless to red). The solution was allowed to cool to room temperature, centrifuged and the supernatant, containing the nanoparticles, was retained.
4.2. SAM formation
4.2.1. Preparation of Si3N4 substrates
Si/Si3N4 substrates (500 nm thick Si3N4, Silson Ltd, Northampton, UK) were cleaned and hydroxylated using a method described by Wei et al. Citation29. The substrates were rinsed well in EtOH and then in ultra high quality (UHQ) water (resistivity = 18 M Ω cm). The substrates were then immersed in Piranha solution (7 parts H2SO4 (98%): three parts H2O2 (30%)) at 90–100°C for 30 min and then rinsed thoroughly with UHQ water (Caution: Piranha solution should be handled with extreme caution as it can be explosive upon contact with organic materials).
The substrates were then immersed in NaOH (0.5 M) for 20 min, HCl (0.1 M) for 10 min and NaOH (0.5 M) for 10 min and rinsed with UHQ water between each immersion. The substrates were then rinsed with HCl (0.1 M), water and then purged with N2(g) for 30 min.
The resonating flaps were cut using focused ion beam etching at beam energy of 30 keV in a Dualbeam Strata 235 FIB/SEM system from FEI Co.
4.2.2. Formation of APTMS SAMs
The vapour deposition process used for the Si3N4 substrate modification with APTMS involves the use of a glass vapour chamber as described previously Citation22. The vapour chamber consists of two parts with the top section consisting of a gas tap. The bottom section of the chamber consists of an Al gauze, on which the Si3N4 substrates are placed, and an inlet, in which a Subaseal stopper is placed allowing for the injection of APTMS. After the Si3N4 substrates have been placed in the chamber, and the top section attached, the chamber was purged with N2(g). The chamber was heated under vacuum, using a heat gun, to encourage desorption of water adsorbed on the inside of the chamber. After the chamber had cooled to room temperature, the APTMS liquid (2 mL) was injected through the Subaseal, whilst the chamber was continuously evacuated for a further 30 min. The gas tap was closed leaving the substrates in an atmosphere of APTMS vapour. After a further 30 min the vacuum was released and the substrates were removed from the chamber. The modification of the Si3N4 substrates was performed at a deposition pressure of 168 mbar.
After the vapour deposition the substrates were removed from the chamber and rinsed sequentially in EtOH, CHCl3 and EtOH then cured in a vacuum oven at 120°C for 30 min.
4.3. Au nanoparticle adsorption studies
Aqueous solutions of NaOH (1 mM) (Fisher Scientific) and HCl (1 mM) (37%, Fisher Scientific) were prepared and used as stock solutions for the pH adjustment of the citrate-passivated gold nanoparticle solutions. The pH values of the solutions were adjusted by adding minimal amounts of the stock solutions to citrate-passivated gold nanoparticle solution (4 mL) with the use of a micropipette. The pH values of the solutions were monitored using a digital pH meter (IQ Scientific Instruments). The APTMS-modified silicon nitride surfaces were immersed in one of the pH altered citrate-passivated Au nanoparticle solutions for 2 h and rinsed well with UHQ water and dried under a stream of N2(g).
4.4. Characterisation
4.4.1. AFM
AFM images were obtained using a MultiMode Scanning Probe Microscope (Veeco) and the images analysed using Nanoscope III v5.12r software. All AFM images were obtained by operating the AFM in non-contact mode with the use of RTESP–Tap300 Metrology Probes (Veeco) (nominal spring constant = 20–80 Nm−1, nominal resonant frequency = 288–328 kHz).
4.4.2. XPS
XPS measurements were performed using a VG ESCALab 250 equipped with an Al Kα X-ray source (1486.68 eV), which was operated at 15 kV. Peak fitting and analysis of the data was carried out using Avantage software. All XPS peaks have been fitted by setting the C1s peak to 284.6 eV.