Abstract
A solution-based processing method has been used to synthesise nanostructured titanium dioxide (TiO2) with different crystalline forms ratio (anatase, rutile and their mixtures) by controlling the hydrolysis of titanium tetrachloride in aqueous solution. The contents of anatase and rutile phases in the synthesised TiO2 powders have been successfully controlled by simply changing the proportion of in the aqueous phase. The prepared materials were characterised by X-ray diffraction, transmission electron microscopy, scanning electron microscope and Brunauer–Emmett–Teller techniques. The experimental results showed that the average size of the synthesised particles was in the range of 6–11 nm. The photocatalytic performance of the synthesised TiO2 nanoparticles was evaluated by removal of the dye, C. I. Acid Blue 9 (AB9), under UV light illumination (30 W). According to the results, rutile appeared to be a poor photocatalyst while the mixed-phase TiO2 (rutile–anatase) demonstrated the highest photoactivity. The efficiency parameters such as apparent quantum yield and electrical energy per order were estimated and compared for different crystalline forms of TiO2. It was found that the photocatalysis process with synthesised mixed-phase TiO2 nanoparticles, containing 70% anatase, had lower electrical energy consumption and higher quantum yield compared with others.
1. Introduction
Titanium dioxide (TiO2) has attracted significant attention from researchers because of the many interesting physical and chemical properties that make it suitable for a variety of applications. For instance, TiO2 has high corrosion resistance and chemical stability and an excellent optical transparency in the visible and near infrared regions, as well as a high refractive index that makes it useful for anti-reflection coatings in optical devices Citation1. It has been used mostly as a pigment in paints, sunscreens, ointments and toothpaste since its commercial production in the early twentieth century. TiO2 pigments are inorganic chemical products used for imparting whiteness, brightness and opacity to a diverse range of applications and end-use markets, including coatings, plastics, paper and other industrial and consumer products Citation2,Citation3. TiO2 is obtained from a variety of ores that contain ilmenite, rutile, anatase and leucoxene, which are mined from deposits located throughout the world. Crystals of TiO2 can exist in one of the three crystalline forms: rutile, anatase and brookite. Only anatase and rutile forms have good pigmentary properties, and rutile is more thermally stable than anatase. The crystallographic properties of rutile and anatase have been reported in Citation4. Most TiO2 pigments have been produced from titanium mineral concentrates by the so-called chloride or sulphate process, either as the rutile or the anatase form.
Table 1. Crystallographic properties of rutile and anatase Citation4.
Since the discovery of photocatalytic splitting of water on TiO2 electrodes Citation5, efforts have been devoted to the development of efficient water and air purification technologies, based on TiO2 photocatalysis. Such treatments typically oxidise toxic organic compounds to non-toxic inorganic compounds, such as carbon dioxide, water, ammonium or nitrates, and chloride ions Citation6.
In the framework of the rapid development of nanoscience and nanotechnology, the domain of nanostructured materials, such as nanostructured TiO2, is necessitating more research, both of an academic and of an industrial nature. Synthesis methods are a major prerequisite for achievement in this rapidly evolving field. The essential first step in the use of nanomaterials in various technologies is their production Citation7,Citation8.
There are many methods of producing nanostructured TiO2, such as the hydrothermal method Citation9,Citation10, sol–gel technique Citation11,Citation12, chemical vapour deposition (CVD) Citation13–15, physical vapour deposition Citation16,Citation17, solvothermal method Citation18,Citation19, electrochemical approaches (e.g. anodising of Ti) Citation20–22, solution combustion method Citation23–25, microemulsion Citation26,Citation27, micelle and inverse micelle methods Citation28,Citation29, ball milling Citation30, a flame by vapour phase Citation31, sonochemical reactions Citation32 and plasma evaporation Citation33,Citation34. It should be noted that as precursors of nanocrystalline oxide powders, however, inorganic compounds are more economical than alkoxides. Since the phases and morphologies of TiO2 are critical parameters in determining their suitability for particular applications, it is still necessary to explore new methods for synthesising nanostructured TiO2, and especially to explore the methods fit for selectively preparing various TiO2 phases (anatase, rutile and their mixtures).
Therefore, in this work, the solution-based processing method has been used to synthesise various crystalline phases of nanostructured TiO2 including anatase, rutile and their mixtures, by controlling the hydrolysis of TiCl4 in aqueous solution. The photocatalytic activity of the synthesised nanostructured TiO2 samples has been tested in decolorisation of the dye solution containing C. I. Acid Blue 9.
C. I. Acid Blue 9, which belongs to the acidic dyes group, is soluble in cold water and methanol. It can be found in textile (as a dye for wool and silk), foodstuff and pharmaceutical wastewaters. In addition, C. I. Acid Blue 9 is the principal active ingredient in Aquashade, which can be used as an aquatic algaecide/herbicide, in natural or manmade ponds, lakes, fountains, fish farms and fish hatcheries, and may be applied by both professional applicators and homeowners. It is hazardous in case of ingestion, skin contact (irritant), eye contact (irritant), and inhalation Citation35,Citation36.
2. Experimental details
2.1. Reagents
All inorganic compounds were obtained from Merck, Germany. C. I. Acid Blue 9 was obtained from Shimi Keshavarz Company, Iran. Its structure and characterisation are given in .
Table 2. Structure and characteristics of C. I. Acid Blue 9.
2.2. Preparation of TiO2 nanoparticles
The nanocrystalline TiO2 reported in this study was prepared by TiCl4 hydrolysis. Titanium tetrachloride (98% TiCl4) was used as a main starting material without any further purification. The appreciated amount of TiCl4 was dissolved in distilled water in an ice-water bath (). The concentration of titanium was adjusted to 3 M. This aqueous solution was then mixed with (NH4)2SO4 solution for preparation of anatase in a temperature-controlled bath. The mixture was stirred at high speed while the amount of TiCl4 solution necessary for the desired [H2O]: [Ti] molar ratio was added dropwise. Having maintained it at the hydrolysis temperature (70°C) for 1 h, the mixed solution was treated with 2.5 M dilute NH4OH until the pH value was 7. For preparation of rutile crystalline, the TiCl4 solution was diluted with 0.5 M HCl solution (70°C) and was not treated with NH4OH solution. Then, the mixed solution was placed into a constant temperature (70°C) bath for 5 h. Subsequently, the precipitated titanium oxide (TiO2–nH2O) was separated from the solution by using centrifugation and repeatedly washed with distilled water to make TiO2–nH2O that was free of chloride ions. The hydrous titanium oxide was dried at 110°C under vacuum and ground to fine powder (TiO2 powder 1), then was calcined at 400°C for 2 h (). For preparation of a mixture of anatase and rutile phase TiO2 powder, a small amount of (NH4)2SO4 was added and the concentration of TiCl4 was adjusted to 0.5 M with distilled water. The rest of the preparation steps are just like the preparation method of anatase in a temperature-controlled bath.
2.3. Characterisation of the nanostructured TiO2
To determine the crystal phase composition and average crystalline size of synthesised nanostructured TiO2 samples, X-ray diffraction (XRD) measurements were carried out at room temperature by using Siemens XRD D5000, with Cu Kα radiation. The accelerating voltage of 40 kV and emission current of 30 mA was used. The average crystalline size of the samples was calculated according to Debye–Scherrer formula Citation37:
The samples used for transmission electron microscopy (TEM) observations were prepared by dispersing the powders in distilled water followed by ultrasonic vibration (Sonoplus Ultrasonic Homogeniser HD 2200, Germany) for 15 min, then placing a drop of the dispersion onto a copper grid coated with a layer of amorphous carbon. A LEO 906 (60 kV) TEM was used to study the morphology. The surface morphology of the catalysts was observed by scanning electron microscope (SEM). The samples for SEM imaging were coated with a thin layer of gold film to avoid charging. A LEO 440i SEM was used to study the morphology.
Nitrogen sorption analyses were obtained with a Micromeritics Tristar sorptometer using standard continuous procedures at 77.15 K on calcined samples that had been degassed at 363 K for 1 h and then at 403 K under high vacuum for at least 10 h. Surface area was calculated according to the Brunauer–Emmett–Teller (BET) model Citation39 over a relative pressure range of 0.05–0.30. Pore diameter distribution was calculated according to the Barret–Joyner–Halenda (BJH) method Citation39, modified by the Halsey thickness curve correction Citation40 on the desorption branch.
2.4. Photocatalytic experiments
The photocatalytic test-reaction chosen to characterise the different synthesised nanostructured TiO2 samples was the decolorisation of the dye solution containing C. I. Acid Blue 9, selected as a model organic pollutant. In the UV/TiO2 process, irradiation was carried out with a 30 W (UV-C) mercury lamp (Philips, Holland), which was put above a batch photocatalytic reactor of 500 mL in volume. On the surface of the solution, the light intensity was measured by Cassy Lab, Germany. The desired concentration of C. I. Acid Blue 9 (20 mg L−1) and TiO2 were fed into the pyrex reactor. The TiO2 suspension was sonicated for 15 min, before illumination, to disperse TiO2 uniformly in the solution by sonoplus ultrasonic homogeniser HD 2200, Germany. The value of incident photon flux by reactor volume unit at 254 nm was 2.33 × 10−6 Einstein L−1 s−1, which was calculated on the base of ferrioxalate actinometry measurement Citation41. The pH of the solution was adjusted using dilute solutions of sulphuric acid and aqueous sodium hydroxide when necessary and measured by pH meter (Metrohm, Switzerland). The UV lamp was switched on to initiate the reaction. During the irradiation, agitation was maintained to keep the solution homogenous and to increase mass transfer coefficient and, as a result, the overall dye decomposition rate. At specific time intervals, the concentration of the dye in each sample was determined using a Lightwave S2000 (England) UV/Vis spectrophotometer at λ max = 625 nm. A linear correlation was established between the dye concentration and the absorbance at λ max = 625 nm, in the range of 0–35 mg L−1 with a coefficient of correlation r 2 = 0.9993. The equation used to calculate the photocatalytic removal efficiency (X) in the treatment experiments was:
3. Results and discussion
3.1. Preparation mechanism of different crystallite phases of TiO2
The mechanism of forming different crystallite phases of nanostructured TiO2 has been showed in . From metal halide to the formation of metal oxide, there are two processes: hydrolysis and polycondensation Citation42. When the titanic halide reacts with water, the titanium ion first increases its coordination by using its vacant d orbitals to accept oxygen electron pairs from nucleophilic ligands (such as −OH groups) Citation43. Consequently, the titanium ion forms an octahedral structure of (Ti(O) m (OH) n (H2O)6 − m − n )(2m + n − 4) −. Because the reaction is performed under an acidic medium, only −OH and −OH2 groups will be present, and the octahedral structure of the titanium ion becomes (Ti(OH) m (H2O)6 − m )(m − 4) − Citation44. Then, the structures dehydrate each other and polycondensed into the final precipitate.
Figure 2. Proposed mechanism for preparation of TiO2 with rutile or anatase phases: (a) the orientation of the third octahedron determines whether a rutile or an anatase nucleus is formed; (b) interaction between and
octahedral hydroxyls; (c) two
octahedra share edge in the presence of
; (d) formation of anatase in the presence of
.
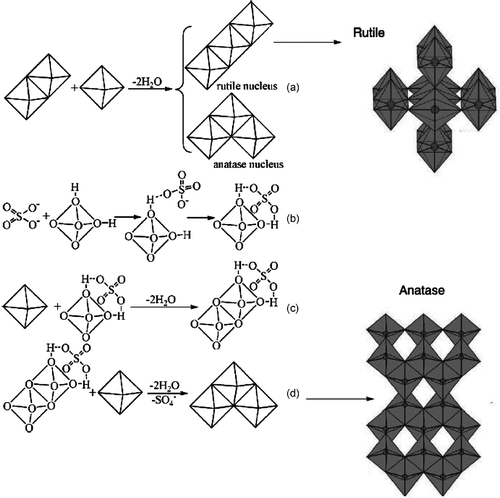
Crystals of TiO2 can exist in one of the three crystalline forms: rutile, anatase or brookite (). In their structures, the basic building block consists of a titanium atom surrounded by six oxygen atoms in a more or less distorted octahedral configuration. In all three TiO2 structures, the stacking of the octahedra results in three-fold coordinated oxygen atoms. The fundamental structural units in these three TiO2 crystals forming from TiO6 octahedron units and having different modes of arrangement and links as presented in . In the rutile form, TiO6 octahedra link by sharing an edge along the c-axis to form chains. These chains are then interlinked by sharing corner oxygen atoms to form a three-dimensional framework, whereas in anatase, the three-dimensional framework is formed only by edge-shared bonding among TiO6 octahedrons. It means that octahedra in anatase share four edges and are arranged in zigzag chains along Citation44–48.
As can be seen in , for forming anatase and rutile nuclei, the placement of the third octahedron is very important and determines whether a rutile or an anatase nucleus is formed. However, the presence of would influence the orientation of the third octahedron. When
ions exist in the acid reaction media, these ions would interact with octahedral hydroxyls by static electricity. Because of the steric effect of
, the octahedron with
and another octahedron would polycondense along the converse direction in order to decrease the repulsion, and the orientation of the third octahedron is more conducive to the formation of an anatase nucleus. The more the
, the more anatase nuclei can be formed. TiO2 clusters grow further on the nucleus and then form the anatase phase. It has been reported that the presence of
accelerated the growth of TiO2 clusters to anatase Citation47,Citation48. As a result, different rutile/anatase mixtures can be prepared by changing the proportion of
in the aqueous phase. On the other hand, there is a weak steric effect for Cl− because of its small radius. Meanwhile, the addition of Cl− generally favours the formation of rutile crystallites Citation47–49.
As was mentioned, TiO2 is known to possess three crystalline structures, which include rutile, anatase and brookite. In coming up with these crystal structures and to estimate the crystal grain size of anatase, rutile and brookite, the XRD experimental method is used. The XRD patterns of the synthesised anatase, rutile and their mixture have been reported in . It can be observed from that anatase peaks in XRD are at 2θ = 25.3°, 37.8°, and 48.1°, while the rutile peaks are at 2θ = 27.5°, 36.2°, and 54.4°, where θ is the XRD angle. This result confirmed that the addition of sulphate was quite effective in promoting the formation of the anatase phase as the pH value rose as a result of the neutralisation of NH4OH. The characteristics of the synthesised nanostructured TiO2 have been reported in . The TEM images of the synthesised TiO2 have been shown in . These show the size distribution of TiO2 particles. The average diameter of the particles measured from the TEM images is in good agreement with XRD results.
Figure 3. X-ray diffraction (XRD) patterns of synthesised TiO2 samples: (a) anatase, (b) rutile (c) rutile–anatase.
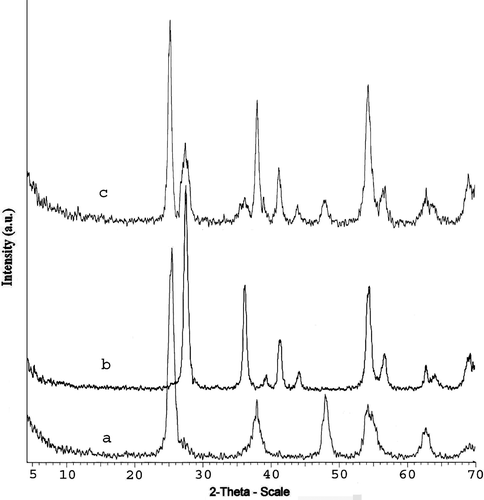
The prepared TiO2 powders, either in the presence of ions or not, dried at room temperature under vacuum, were dominantly amorphous (). The morphology of the amorphous TiO2 powder observed by SEM is shown in .
3.2. Photocatalytic activity of the synthesised TiO2 nanoparticles
The photocatalytic decolorisation of the solution containing C.I. Acid Blue 9 under UV illumination was used to evaluate the photoactivity of the synthesised TiO2 nanoparticles. We found that TiO2 and UV light had a negligible effect when they were used on their own. The reason for this observation is due to the fact that when TiO2 is illuminated with light of energy greater than its optical band gap (λ < 390 nm), an electron is transferred to the conduction band, leaving a hole in the valance band. This is called production of an electron-hole (e − /h + ) pair. At the solid liquid interface, electron transfer can occur both from the conduction band to an electron acceptor (O2, H+, etc.) in the solution and from a donor (OH−, H2O, organic pollutants (P), etc.) in the solution to the valence band hole. Moreover, the hydroxyl radicals formed are strong oxidants and attack organic pollutants present at or near the surface of TiO2. This causes photodegradation of pollutants Citation50–52. The mechanism is assumed up in .
The characteristic absorbance at 625 nm of AB9 solution was monitored during the reaction process. Comparison of the photocatalytic activity of different synthesised TiO2 nanoparticles has been shown in . It is clear that the photocatalytic activity of amorphous TiO2 is negligible. As can be seen from , rutile appeared to be a poor photocatalyst while the mixed-phase TiO2 (rutile–anatase) demonstrated the highest photoactivity. In a recent study by Gray et al. Citation53, mixed-phase TiO2 nanocomposites were synthesised using a hydrothermal method for photooxidation and photoreduction applications. These authors found that the anatase–rutile nanocomposite exhibited the highest photoactivity among the catalysts tested. They also claim that a synergic effect exists between the anatase and rutile particles, in which spatial charge separation hinders charge recombination Citation53. In addition, it has long been empirically observed that mixed-phase TiO2, i.e. TiO2 containing both anatase and rutile, tends to exhibit higher photocatalytic activities than pure-phase TiO2. When Bacsa and Kiwi Citation54 prepared TiO2 samples with different ratios of anatase : rutile, the highest photoactivity was obtained with a 70 : 30 ratio, which is similar to that of Degussa P25. In our study, the anatase : rutile ratio of the mixed-phase TiO2 was 70 : 30 ().
Figure 7. Comparison of the photocatalytic activity of different synthesised TiO2 nanoparticles. [TiO2]0 = 150 mg L−1, [AB9]0 = 20 mg L−1, pH = 6.2, V = 50 mL, I = 11.2 W m−2: (a) during different irradiation times, (b) at the irradiation time of 90 min.
![Figure 7. Comparison of the photocatalytic activity of different synthesised TiO2 nanoparticles. [TiO2]0 = 150 mg L−1, [AB9]0 = 20 mg L−1, pH = 6.2, V = 50 mL, I = 11.2 W m−2: (a) during different irradiation times, (b) at the irradiation time of 90 min.](/cms/asset/c9bb81c4-be33-4a25-b42e-3ee78e9f15de/tjen_a_393166_o_f0007g.gif)
Table 3. Physicochemical characteristics of the synthesised TiO2 nanoparticles.
A possible reason for the improved performance of such mixed-phase TiO2 samples is that rutile acts as a sink for the electrons generated in anatase, serving to physically separate the electron-hole and thereby depress rates of recombination Citation55 (). This model is consistent with the fact that the band edges of rutile lie within those of anatase; i.e. the potential of the conduction band edge of anatase is more negative than that of rutile. However, it has been recently shown that just the opposite occurs: rutile undergoes band gap activation, and electrons are shuttled from rutile to anatase sites, which must be of lower energy (). This implies that one or more trap sites exist on anatase at potentials more positive than the conduction band edge of either anatase or rutile. This was recently confirmed by a photoacoustic spectroscopy study of anatase, which found trap sites on anatase at an average of 0.8 eV below the conduction band edge Citation55,Citation56.
Figure 8. Models of mixed-phase TiO2: (a) rutile islands surround anatase particles, and rutile is an electron sink; (b) a small rutile core surrounded by anatase crystallites, where electrons are shuttled from rutile to anatase.
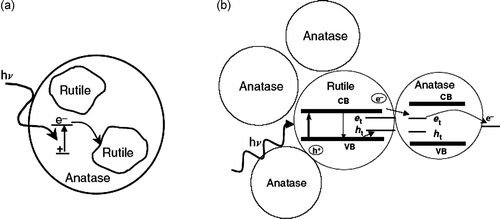
It can be concluded that the size and morphology of rutile and anatase nanocrystals are critical to the separation and enhanced activity of mixed-phase TiO2. As was illustrated in , an emerging model of mixed-phase TiO2 particles describes an atypically small rutile core surrounded by anatase crystallites. Catalytic ‘hot spots’ are believed to exist at the intersection of the two phases, where distorted geometry gives rise to unique surface chemistry.
The photocatalytic activity of TiO2 materials can be influenced by many factors, including crystal structure, particle size, surface area, porosity, density of surface hydroxyl groups, surface acidity, number and nature of trap sites, and adsorption–desorption characteristics Citation35,Citation53. For a catalyst, a key requirement to demonstrate high activity is to have the small primary particle size and high surface area. As can be seen in , the primary particle size of the synthesised mixed-phase TiO2 (6–10 nm) is smaller than that others.
shows a typical time-dependent UV-Vis spectrum of AB9 solution during photocatalysis. The spectrum of AB9 in the visible region exhibits a main band with a maximum at 625 nm. As is clear from this figure, the absorption peaks diminished and finally disappeared under reaction, which indicated that the AB9 had been destructed. No new absorption bands appear in either the visible or ultraviolet regions. Complete decolorisation of the dye solution was observed after 90 min in the optimised conditions. Since photocatalysis of aqueous organic pollutant is an electric-energy-intensive process, and electric energy can represent a major fraction of the operating costs, simple figures-of-merit based on electric energy consumption can be very useful and informative. The photochemistry commission of the International Union of Pure and Applied Chemistry (IUPAC) proposed a figure-of-merit (or more appropriately, an Efficiency Index, as it compares electrical efficiency of different AOPs) for UV-based AOPs Citation57. Electrical energy per order (E EO) compares electrical efficiency of different UV-based AOPs and is a measure of the electrical efficiency of an AOP system. It is defined (for low concentration of pollutants) as the electrical energy in kilowatt hours (kWh) required bringing about the degradation of a contaminant by one order of magnitude in 1m3 (1000 L) of contaminated water. Generally, the higher the energy efficiency of a process, the lower the E EO. The values of E EO can be calculated using the following Equation Citation57,Citation58:
Figure 9. UV-Vis spectrum of AB9 (20 mg L−1) during photocatalysis in the presence of the synthesised mixed-phase TiO2 nanoparticles. [TiO2]0 = 150 mg L−1, pH = 6.2, I = 11.2 W m−2.
![Figure 9. UV-Vis spectrum of AB9 (20 mg L−1) during photocatalysis in the presence of the synthesised mixed-phase TiO2 nanoparticles. [TiO2]0 = 150 mg L−1, pH = 6.2, I = 11.2 W m−2.](/cms/asset/5f551818-55ea-4aa8-9974-4974895122a8/tjen_a_393166_o_f0009g.gif)
The electric dose (kWh m−3 order−1) required to oxidise C. I. Acid Blue 9 (20 mg L−1) in the presence of synthesised TiO2 nanoparticles has been reported in . It is clear that a photocatalytic process with synthesised mixed-phase TiO2 nanoparticles offered the best energy efficiency. Finally, it is useful to relate the E EO values found in this study to the treatment costs. For instance, if the cost of the electricity in Iran and France is $0.0065 and $0.092 per kWh, the contribution to treatment cost of 20 mg L−1 AB9 solution, in the presence of the mixed-phase TiO2, from electrical energy will be $ 4.0 and $ 56.7 per m3, respectively. In addition there will be smaller cost factors for the photocatalyst used and for UV lamp replacement.
Table 4. Rate constants, half-lives, electrical energy per order and apparent quantum yield for photocatalytic decolorisation of AB9 in the presence of the synthesised TiO2 nanoparticles. [AB9]0 = 20 mg L−1, [TiO2]0 = 150 mg L−1, pH = 6.2.
The efficiency of the heterogeneous photocatalytic process can be quantified in term of quantum yield, which may be defined as the rate at which reactant molecules disappear or product molecules form, divided by the number of photons absorbed per unit time. In our study, the apparent quantum yield of photocatalysis process has been calculated by Equations (Equation5) and (Equation6) Citation59,Citation60:
4. Conclusions
TiO2 nanoparticles in anatase, rutile and their mixture phases have been synthesised from aqueous solution of TiCl4. The addition of a small amount of (NH4)2SO4 promoted occurrence of anatase phase and inhibited the anatase–rutile transformation such that the powder was completely anatase after calcining at 400°C for 2h. The anatase–rutile mixture (70 : 30) was prepared by controlling the proportion of in the aqueous phase. The synthesised TiO2 samples have narrow size distribution and their average particle size was 6–11 nm. The mixed-phase TiO2 nanoparticles have reasonable photocatalytic efficiencies, and nearly complete of C. I. Acid Blue 9 was removed in 90 min. The values of electric energy per order and apparent quantum yield calculated in the presence of different synthesised TiO2 samples, demonstrated that the most efficient process was that using mixed-phase TiO2 nanoparticles containing 70% anatase.
Acknowledgements
The authors thank the University of Tabriz, Iran for financial and other supports.
References
- Shan , CX , Hou , X and Choy , K . 2008 . Corrosion resistance of TiO2 films grown on stainless steel by atomic layer deposition . Surf. Coat. Tech. , 202 : 2399 – 2402 .
- Boisvert , P , Persello , J , Foissy , A , Castaing , J and Cabane , B . 2000 . Effect of surface charge on the adsorption mode of sodium poly(acrylate) on alumina-coated TiO2 used as coating pigment . Colloid Surf. A , 168 : 287 – 296 .
- Zallen , R and Moret , MP . 2006 . The optical absorption edge of brookite TiO2 . Solid State Commun. , 137 : 154 – 157 .
- Zheng , M , Gu , M , Jin , Y and Jin , G . 2000 . Preparation, structure and properties of TiO2–PVP hybrid films . Mat. Sci. Eng. B: Solid , 77 : 55 – 59 .
- Fujishima , A and Honda , K . 1972 . Electrochemical photolysis of water at a semiconductor electrode . Nature , 238 : 37 – 38 .
- Daneshvar , N , Salari , D and Khataee , AR . 2003 . Photocatalytic degradation of azo dye acid red 14 in water: investigation of the effect of operational parameters . J. Photochem. Photobiol. A , 157 : 111 – 116 .
- Yates , T Jr and Thompson , L . 2006 . Surface science studies of the photoactivation of TiO2− new photochemical processes . Chem. Rev. , 106 : 4428 – 4453 .
- Chen , X and Mao , SS . 2007 . Titanium dioxide nanomaterials: synthesis, properties, modifications, and applications . Chem. Rev. , 107 : 2891 – 2959 .
- Mori , K , Maki , K , Kawasaki , S , Yuan , S and Yamashita , H . 2008 . Hydrothermal synthesis of TiO2 photocatalysts in the presence of NH4F and their application for degradation of organic compounds . Chem. Eng. Sci. , 63 : 5066 – 5070 .
- Wang , G . 2007 . Hydrothermal synthesis and photocatalytic activity of nanocrystalline TiO2 powders in ethanol–water mixed solutions . J. Molecular Catal. , 274 : 185 – 191 .
- Kao , L , Hsu , T and Lu , H . 2007 . Sol-gel synthesis and morphological control of nanocrystalline TiO2 via urea treatment . J. Colloid Interf. Sci. A , 316 : 160 – 167 .
- Xiao , Q , Si , Z , Yu , Z and Qiu , G . 2007 . Sol-gel auto-combustion synthesis of samarium-doped TiO2 nanoparticles and their photocatalytic activity under visible light irradiation . Mat. Sci. Eng. B: Solid , 137 : 189 – 194 .
- Zhang , X , Zhou , M and Lei , L . 2005 . Preparation of anatase TiO2 supported on alumina by different metal organic chemical vapor deposition methods . Appl. Catal. A , 282 : 285 – 293 .
- Jung , S , Kim , S , Imaishi , N and Cho , Y . 2005 . Effect of TiO2 thin film thickness and specific surface area by low-pressure metal–organic chemical vapor deposition on photocatalytic activities . Appl. Catal. B , 55 : 253 – 257 .
- Su , Y , Zhang , X , Han , S , Chen , X and Lei , L . 2007 . F-B-codoping of anodized TiO2 nanotubes using chemical vapor deposition . Electrochem. Commun. , 9 : 2291 – 2298 .
- Giolli , C , Borgioli , F , Credi , A , Fabio , A , Fossati , A , Miranda , M , Parmeggiani , S , Rizzi , G , Scrivani , A and Troglio , S . 2007 . Characterization of TiO2 coatings prepared by a modified electric arc-physical vapour deposition system . Surf. Coat. Tech. , 202 : 13 – 22 .
- Chiu , S , Chen , Z , Yang , K , Hsu , Y and Gan , D . 2007 . Photocatalytic activity of doped TiO2 coatings prepared by sputtering deposition . J. Mater. Process. Tech. , 192–193 : 60 – 67 .
- Kang , M . 2005 . The superhydrophilicity of Al–TiO2 nanometer sized material synthesized using a solvothermal method . Mater. Lett. , 59 : 3122 – 3127 .
- Wahi , RK , Liu , Y , Falkner , CJ and Colvin , LV . 2006 . Photocatalytic activity of doped TiO2 coatings prepared by sputtering deposition . J. Colloid Interf. Sci. , 302 : 530 – 536 .
- Sankapal , BR , Sartale , SD , Lux-Steiner , MC and Ennaoui , A . 2006 . Chemical and electrochemical synthesis of nanosized TiO2 anatase for large-area photon conversion . Compt. Rendus Chim. , 9 : 702 – 707 .
- Prakasam , EH , Shankar , K , Paulose , M , Varghese , KO and Grimes , CA . 2007 . A new benchmark for TiO2 nanotube array growth by anodization . J. Phys. Chem. C , 111 : 7235 – 7241 .
- Tacconi , NR , Chenthamarakshan , CR , Yogeeswaran , G , Watcharenwong , A , Zoysa , RS , Basit , AN and Rajeshwar , K . 2006 . Nanoporous TiO2 and WO3 films by anodization of titanium and tungsten substrates: influence of process variables on morphology and photoelectrochemical response . J. Phys. Chem. A , 110 : 25347 – 25355 .
- Nagaveni , K , Sivalingam , G , Hegde , MS and Madras , G . 2004 . Photocatalytic degradation of organic compounds over combustion-synthesized nano-TiO2 . Environ. Sci. Technol. , 38 : 1600 – 1604 .
- Sivalingam , G , Priya , MH and Madras , G . 2004 . Kinetics of the photodegradation of substituted phenols by solution combustion synthesized TiO2 . Appl. Catal. B , 51 : 67 – 76 .
- Sivalingam , G and Madras , G . 2004 . Photocatalytic degradation of poly(bisphenol-A-carbonate) in solution over combustion-synthesized TiO2: mechanism and kinetics . Appl. Catal. A , 269 : 81 – 90 .
- Mishra , T . 2008 . Anion supported TiO2–ZrO2 nanomaterial synthesized by reverse microemulsion technique as an efficient catalyst for solvent free nitration of halobenzene . Catal. Commun. , 9 : 21 – 26 .
- Lee , M , Lee , G , Ju , C and Hong , S . 2005 . Preparations of nanosized TiO2 in reverse microemulsion and their photocatalytic activity . Sol. Energ. Mat. Sol. C. , 88 : 389 – 401 .
- Ohno , T , Inaba , R , Fukahori , T and Hamamoto , M . 2006 . Preparation of S-doped TiO2 photocatalysts and their photocatalytic activities under visible light . J. Molecular Catal. A , 260 : 247 – 254 .
- Sui , X , Chu , Y , Xing , S , Yu , M and Liu , C . 2004 . Self-organization of spherical PANI/TiO2 nanocomposites in reverse micelles . Colloid. Surface. A , 251 : 103 – 107 .
- Shifu , C , Lei , C , Shen , G and Gengyu , C . 2005 . The preparation of nitrogen-doped photocatalyst TiO2−xNx by ball milling . Chem. Phys. Lett. , 413 : 404 – 409 .
- Kim , BK , Lee , GG , Park , MH and Kim , NJ . 1999 . Characteristics of nanostructured TiO2 powders synthesized by combustion flame-chemical vapor condensation process . Nanostruct. Mater. , 12 : 637 – 640 .
- Guo , W , Lin , Z , Wang , X and Song , G . 2003 . Sonochemical synthesis of nanocrystalline TiO2 by hydrolysis of titanium alkoxides . Microelectron. Eng. , 66 : 95 – 101 .
- Miyata , T , Tsukada , S and Minami , T . 2006 . Preparation of anatase TiO2 thin films by vacuum arc plasma evaporation . Thin Solid Films , 496 : 136 – 140 .
- Huang , H and Yao , X . 2005 . Preparation and characterization of rutile TiO2 thin films by mist plasma evaporation . Surf. Coat. Tech. , 191 : 54 – 58 .
- Daneshvar , N , Salari , D , Niaei , A and Khataee , AR . 2006 . Photocatalytic degradation of the herbicide erioglaucine in the presence of nanosized titanium dioxide: comparison and modeling of reaction kinetics . J. Environ. Sci. Health B , 41 : 1273 – 1290 .
- Flury , M and Flühler , H . 1995 . Tracer characteristics of brilliant blue FCF . Soil Sci. Soc. Am. J. , 59 : 22 – 27 .
- Patterson , AL . 1939 . The scherrer formula for x-ray particle size determination . Phys. Rev. , 56 : 978 – 982 .
- Spurr , RA and Myers , H . 1957 . Quantitative analysis of anatase-rutile mixtures with an x-ray diffactometer . Anal. Chem. , 29 : 760 – 762 .
- Brunauer , S , Emett , PH and Teller , EJ . 1938 . Adsorption of gases in multimolecular layers . J. Am. Chem. Soc. , 60 : 309 – 319 .
- Halsey , GD Jr . 1948 . Physical adsorption on nonuniform surfaces . J. Chem. Phys. , 16 : 931 – 937 .
- Kuhn , H , Braslavsky , SE and Schmidt , R . 2004 . Chemical actinometry, IUPAC technical report . Pure Appl. Chem. , 76 : 2105 – 2146 .
- Wang , C and Ying , JY . 1999 . Sol-gel synthesis and hydrothermal processing of anatase and rutile titania nanocrystals . Chem. Mater. , 11 : 3113 – 3120 .
- Livage , J , Henry , M and Sanchez , C . 1988 . Sol-gel chemistry of transition metal oxides . Prog. Solid State Chem. , 18 : 259 – 341 .
- Zhang , J , Yan , M , Chen , F and Anpo , M . 2005 . Preparation of controllable crystalline titania and study on the photocatalytic properties . J. Phys. Chem. B , 109 : 8673 – 8678 .
- Li , Y , White , TJ and Lim , SH . 2004 . Low-temperature synthesis and microstructural control of titania nano-particles . J. Solid State Chem. , 177 : 1372 – 1381 .
- Janisch , R , Gopal , P and Spaldin , N . 2005 . Transition metal-doped TiO2 and ZnO-present status of the field . J. Phys.: Condens. Matter. , 17 : 657 – 689 .
- Zhang , Q , Gao , L and Guo , J . 1999 . Preparation and characterization of nanosized TiO2 powders from aqueous TiCl4 solution . Nanostruct. Mater. , 11 : 1293 – 1300 .
- Diebold , U . 2003 . The surface science of titanium dioxide . Surf. Sci. Rep. , 48 : 53 – 229 .
- Kittaka , S , Matsuno , K and Takahara , S . 1997 . Transformation of ultrafine titanium dioxide particles from rutile to anatase at negatively charged colloid surfaces . J. Solid State Chem. , 132 : 447 – 450 .
- Khataee , AR , Vatanpour , V and Amani , AR . 2009 . Decolorization of C.I. acid blue 9 solution by UV/nano-TiO2, fenton, electro-fenton and electrocoagulation processes: a comparative study . J. Hazard. Mater. , 161 : 1225 – 1233 .
- Daneshvar , N , Salari , D and Khataee , AR . 2003 . Photocatalytic degradation of azo dye acid red 14 in water on ZnO as an alternative catalyst to TiO2 . J. Photochem. Photobiol. A , 162 : 317 – 3221 .
- Daneshvar , N , Salari , D , Niaie , A , Rasoulifard , MH and Khataee , AR . 2005 . Immobilization of TiO2 nanopowder on glass beads for the photocatalytic decolorization of an azo dye C.I. direct red 23 . J. Environ. Sci. Health A , 40 : 1605 – 1617 .
- Gray , K , Li , G , Ciston , S , Saponjic , Z , Chen , L , Dimitrijevic , M and Rajh , T . 2008 . Photooxidation and photoreduction using mixed-phase titanium dioxide . J. Catal. , 253 : 105 – 110 .
- Bacsa , RR and Kiwi , J . 1998 . Effect of rutile phase on the photocatalytic properties of nanocrystalline titania during the degradation of p-coumaric acid . Appl. Catal. B , 16 : 19 – 29 .
- Bickley , RI , Gonzalez-Carreno , T , Lees , JS , Palmisano , L and Tilley , RJD . 1991 . A structural investigation of titanium dioxide photocatalysts . J. Solid State Chem. , 92 : 178 – 190 .
- Grassian , V . 2005 . Environmental Catalysis , USA : CRC Press, Taylor & Francis Group .
- Bolton , JR , Bircger , KG , Tumas , W and Tolman , CA . 2001 . Figure-of merit for the technical development and application of advanced oxidation technologies for both electric and solar-derived systems . Pure Appl. Chem. , 73 : 627 – 637 .
- Daneshvar , N , Aleboyeh , A and Khataee , AR . 2005 . The evaluation of electrical energy per order (EEo) for photooxidative decolorization of four textile dye solutions by the kinetic model . Chemosphere , 59 : 761 – 767 .
- Dijkstra , MFJ , Buwalda , H , Jong , AWF , Michorius , A , Winkelman , JGM and Beenackers , AACM . 2001 . Experimental comparison of three reactor designs for photocatalytic water purification . Chem. Eng. Sci. , 56 : 547 – 555 .
- Lin , YJ , Lee , A , Teng , LS and Lin , HT . 2002 . Effect of experimental factors on nitrobenzaldehyde photoisomerization . Chemosphere , 48 : 1 – 8 .