ABSTRACT
Introduction: Despite significant scientific advances over the past six decades toward the development of safe and effective radiation countermeasures for humans using animal models, only two pharmaceutical agents have been approved by United States Food and Drug Administration (US FDA) for hematopoietic acute radiation syndrome (H-ARS). Additional research efforts are needed to further develop large animal models for improving the prediction of clinical safety and effectiveness of radiation countermeasures for ARS and delayed effects of acute radiation exposure (DEARE) in humans.
Area covered: The authors review the suitability of animal models for the development of radiation countermeasures for ARS following the FDA Animal Rule with a special focus on nonhuman primate (NHP) models of ARS. There are seven centers in the United States currently conducting studies with irradiated NHPs, with the majority of studies being conducted with rhesus monkeys.
Expert opinion: The NHP model is considered the gold standard animal model for drug development and approval by the FDA. The lack of suitable substitutes for NHP models for predicting response in humans serves as a bottleneck for the development of radiation countermeasures. Additional large animal models need to be characterized to support the development and FDA-approval of new radiation countermeasures.
1. Introduction
There are several animal models to study radiation injury and the development of radiation countermeasures for ARS [Citation1]. The syndromic nature of ARS lends itself to a number of animal models being used in research studies based on the injury progression, severity, and percentage of the total body irradiated. Small animals, such as mice and rats, are useful to investigate specific facets of the radiation injury and are also useful for countermeasure screening or early efficacy evaluation for ARS. There are additional animal models such as ferrets, swine (specifically minipig), canine, and rat that are being used to study the pathophysiology of radiation injury and countermeasure efficacy assessment. Some species are specifically suitable for certain end points such as ferrets for GI (emesis) and swine for cutaneous sub-syndrome [Citation2,Citation3]. However, the NHPs are considered the animal models that reproduce the most appropriate representation of human disease. The NHP model is considered the gold standard of animal models for drug development and approval by the FDA and has a high degree of resemblance in terms of pathways of physiological responses and targets that are relevant to human disease.
NHP model has several advantageous attributes such as an organ structure similar to humans, genetic homology, metabolism, long life span, easy sequential sampling, suitable for GI symptoms (vomiting), and this model is as close as possible to humans. Disadvantages of this model include difficulties faced based on ethical consideration, risks associated with handling, a longer breeding period, increased costs associated with housing, feeding, and animal acquisition, and its hirsute nature makes cutaneous studies difficult. The most commonly used NHPs in biomedical research are from the genus Macaca (rhesus and cynomolgus). Other species that are used in drug development include African green monkeys (Chlorocebus aethiops), squirrel monkeys (Saimiri spp.), and baboons (Papio spp.) [Citation4]. The macaques comprise more than 20 species diverging from each other over a long period of time (). The genus Macaca is closely related to humans, sharing 95+% DNA sequence homology with humans. Such a close relationship between humans and macaques has made these animals attractive as animal models for biomedical research, specifically for drug development under the Animal Rule. This model most closely reproduces the histopathological, clinical, and pathophysiological attributes of radiation injury in humans. In addition, because of the longer life span and similar supportive care requirements for ARS, it is possible to link the dose–effect relationships between NHP models and humans following the known medical management and treatment.
Table 1. New World and Old World NHPs.
Moreover, all investigated species of NHPs are susceptible to radiation injury, although most of the in vivo studies involving radiation injury and efficacy of radiation countermeasures for ARS to date have been conducted in the rhesus macaque [Citation1,Citation5–Citation7]. This species has been selected merely based on convenience, cost, and availability rather than due to any scientific reason. Since historical data for comparison is frequently required for disease-specific studies, it is difficult to change from one species to another during the course of studies. Therefore, the sheer number of studies using Rhesus macaques for the development of radiation countermeasures continues to grow. These macaques have contributed significantly to advances in biomedical research. For example, rhesus antigens found in macaques led to the identification of the different blood groups in humans. Depending on the country of origin, rhesus macaques (Macaca mulatta) are known either as Chinese- or Indian origin. Although the subspecies of Indian-origin rhesus macaques (Macaca mulatta mulatta) was the model of choice in biomedical research, restriction on the export of this macaque has significantly reduced the use of these animals in current research and has also led to the increased use of other species/subspecies, specifically macaque of Chinese-origin rhesus macaques (Macaca mulatta lasiota) and crab eating macaque, cynomolgus (Macaca fasicularis).
With respect to Macaca fasicularis, it is an important NHP model in biomedical research; it is specifically used in the area of pharmacology, oncology, diabetes, cardiovascular, and other diseases. Macaca fasicularis is closely related to Macaca mulatta. Although they are distinct in body size, Macaca fasicularis being smaller than Macaca mulatta, these two have been known to form hybrids in the wild. Because of its smaller size, Macaca fasicularis has been extensively used in drug development [Citation8]. In addition to rhesus and cynomolgus macaques, several studies have been conducted using baboons (Papio spp.) to investigate radiation injuries resulting from exposure to X-rays [Citation9], 60Co γ-radiation [Citation10,Citation11], and mixed field (neutron and γ-photon) irradiation [Citation12]. In recent years, various studies have reported significant biomarkers for radiation injuries using the baboon model [Citation13–Citation17]. Furthermore, a number of promising radiation countermeasures under development for H-ARS are being tested in NHPs for efficacy and pharmacokinetics/pharmacodynamics (). A few of these radiation countermeasures at advanced stages of development are discussed below.
Table 2. Some important radiation countermeasures evaluated in NHP models.
2. FDA’s animal rule
Issued by the US FDA in 2002, the ‘Animal Rule’ was intended to expedite the development of new drugs and biologics that can act as countermeasures against chemical, biological, radiological, and nuclear (CBRN) threats. The rule applies exclusively to new agents for which definitive human efficacy studies cannot be conducted as it would be unethical to knowingly expose humans to lethal doses of radiation [Citation1]. The US FDA can grant marketing approval to new products that have been demonstrated in well-controlled animal studies to be safe and capable of producing clinical benefit in humans. The criteria of the FDA’s Animal Rule relevant to development using animal models are as follows:
There is a reasonably well-understood pathophysiological mechanism for toxicity of the agent (radiation), and its prevention, or substantial reduction by the drug;
The effect of the drug or biologic is demonstrated in more than one animal species, for which the expected effect is predictive for humans. The multiple animal requirement can be abrogated if the effect can be demonstrated in a single well-characterized animal model which sufficiently predicts the response in humans;
The animal study’s end point is related to a desired benefit in humans, generally involving the enhancement of survival or prevention of major morbidity;
Pharmacokinetics and pharmacodynamics, or other relevant data or information of the product, in animals and humans, allows selection of an effective human dose [Citation50].
Data from animal efficacy studies is not as conclusive as human efficacy data because it is rare for an animal model to exactly, that is, without approximation mirror human disease. For this reason, it is important to understand how and why a countermeasure works in order to assure that such agent will be effective in humans. Biomarkers for radiation injury and countermeasure efficacy are particularly important in this context.
3. Suitability of NHPs as animals models for studying radiation injury and development of countermeasure
As stated above, the NHP is considered the most suitable large animal model for drug development. In regard to the development of radiation countermeasures for ARS following the Animal Rule, the NHP model has been shown to be particularly relevant.
3.1. Radiation injury and sub-syndromes
Ionizing radiation includes particles (α- and β-particles, neutrons) and high-energy electromagnetic waves (γ- and X-rays), as well as other particles capable of producing ions by knocking electrons off atoms in addition to being capable of breaking chemical bonds in molecules that they interact with. Radiosensitivity is the relative susceptibility of cells, tissues, and organ systems to the injurious action of radiation. In general, the radiosensitivity of cells is directly proportional to the rate of cell division and inversely proportional to the degree of cell differentiation [Citation51]. During cell division, cells in the S phase are the least sensitive and cells in M phase are the most sensitive. Cellular radiosensitivity estimated in ascending order from least sensitive to most sensitive are (1) muscle, brain, and spinal cord cells; (2) bone cells; (3) connective tissue cells; (4) endothelial cells (pleura and peritoneum); (5) kidney epithelial cells; (6) epithelium of lung alveoli and biliary passages; (7) hepatic cells; (8) epidermal stem cells; (9) intestinal epithelial cells; (10) proliferating bone marrow cells; (11) germ cells; (12) lymphoid cells [Citation52] . In brief, the most radio-resistant cells are differentiated cells and the most radiosensitive cells are those that are undifferentiated and have high mitotic rates (e.g. tumor cells, stem cells). However, radiosensitivity at the cellular level does not necessarily translate into higher order effects as in neurovascular, lung, and renal injury after exposure to radiation. The acute effects of radiation apply when the whole body is uniformly irradiated. Furthermore, the sensitivity of organ systems is more complex and not clearly related to cellular differentiation and mitotic rates. Effects can differ significantly when only portions of the body or an individual organ system are exposed to radiation.
ARS manifests in humans following exposure to total-body or partial-body radiation exposure at doses >1 Gy, delivered at a relatively high dose rate. The hematopoietic, gastrointestinal, pulmonary, cutaneous, and central nervous systems are more susceptible to radiation injury than the remaining organ systems [Citation5]. Clinical indications of ARS include hematopoietic (H-ARS; 2–6 Gy), gastrointestinal (GI-ARS; >6 Gy), and neurovascular (>10 Gy) sub-syndromes [Citation53]. These sub-syndromes are somewhat oversimplified because ARS is a continuum, with incremental degrees of physiological imbalances in multiple organs across the dose range [Citation54–Citation56]. There are two additional organ-specific sub-syndromes; pulmonary and cutaneous. The neurovascular ARS is considered incurable, but individuals exposed to lower radiation doses that result in H-ARS or GI-ARS are more likely to benefit from the intervention of medical countermeasures. Hence, these two sub-syndromes are specific targets for the development of radiation countermeasures. Because of the effects of disease progression and symptom severity, the mitigation of GI-ARS alone is not expected to benefit victims because an individual exposed to this level of radiation will likely suffer damage to various organs, including bone marrow, kidney, and lung. Therefore, since the majority of radiation countermeasures are being developed for specific sub-syndromes of ARS, it is reasonable to investigate each sub-syndrome individually in the NHP model.
3.1.1. H-ARS
H-ARS is characterized by a severe loss of hematopoietic stem cells followed by cytopenia and includes the development of neutropenia, thrombocytopenia, and anemia. It occurs due to the radiosensitivity of committed progenitors for various lineages. The time it takes for symptoms to become noticeable is dependent on the rate of stem cells maturation and differentiation. Several animal models of H-ARS have been characterized to study radiation injury and development of radiation countermeasures. The three animal models that have been used in the majority of H-ARS studies are mice, canines, and NHPs [Citation7,Citation57,Citation58]. The commonly used end point for H-ARS assessment is the LD50 (lethal dose of radiation that results in the death of 50% of the affected population). To assess radiation countermeasures for various sub-syndromes, surrogate end points for death and/or elaborate euthanasia criteria are needed for the development of animal models. The time to onset, nadir depth, and rapidity of the recovery for each blood component, specifically neutrophils and platelets, are useful secondary end points as alternative to death as an end point for evaluating the efficacy of countermeasures. It is important to note that the FDA requires that a countermeasure for GI-ARS or H-ARS demonstrates improved survival in order to gain approval. The approval of two radiomitigators for H-ARS has been based on demonstrated efficacy and improved survival as determinants [Citation18,Citation19,Citation59].
Hematopoietic stem cells in bone marrow can survive doses of radiation yet result in H-ARS. As the radiation dose increases, the number of surviving stem cells decreases and the recovery time for blood cells (lymphocytes, neutrophils, monocytes) lineages become protracted. The extent of the radiation-induced cell deficit (nadir) and the time to recovery to the baseline of the blood cell lineages have usually been used as secondary end points to assess the levels of hematopoietic injury. To reduce the periods of severe neutropenia and thrombocytopenia, hematopoietic cell transplantation has been used in radiological accident and clinical settings. To this end, the NHP is a well-characterized model for H-ARS for which the lethal dose–response relationship is well established [Citation60].
3.1.2. GI-ARS
The GI tract is particularly sensitive to irradiation. The GI-ARS is characterized by massive apoptotic cell death in the lower GI epithelium followed by break down of the mucosal epithelial barrier wall and death from electrolyte imbalance and fluid loss; intestinal hemorrhage, and sepsis [Citation61]. There are three different phases for GI-ARS: an initial or prodromal phase occurring during first few hours following radiation exposure, a latent phase which shortens with increasing dose, and the manifest phase. The prodromal phase is characterized by onset of nausea, vomiting, and diarrhea (NVD) as common GI-related symptoms of radiation exposure which can be exacerbated by fluid and electrolyte loss [Citation51]. NVD occurs due to enteritis (damage to the lining of the intestine) as a result of radiation exposure. At radiation doses below the threshold for GI-ARS, mucosal barrier break down allows bacteria to translocate, which can cause sepsis and death [Citation62]. In the long run, tissue repair after radiation exposure alters the structure, motility, and fluid absorption capability of the gut, and resulting fibrosis makes the GI tract more rigid and disposed to stenosis, adhesions, and perforation [Citation63]. Lethality as a result of intestinal failure is the primary end point for GI-ARS studies. The histopathological end point for GI-ARS is the number of regenerating crypts measured in the intestine at defined times [Citation64]. The NHP model is well characterized for investigating various parameters of GI-ARS. Physiological assays of GI injury include GI motility, bacterial translocation into the blood stream, permeability, and levels of plasma citrulline [Citation65–Citation67]. In addition to these end points, radiation-induced vomiting/emesis has been extensively studied in other animals, and the mechanisms underlying this indication may vary between models [Citation68,Citation69]. Vomiting is the reflexive act of forcefully ejecting the stomach contents through the mouth by coordinated muscle contraction. Clinical studies have demonstrated that patients receiving total-body irradiation (TBI) or upper-abdominal irradiation frequently display nausea, retching, and vomiting as side effects.
3.1.3. Neurovascular syndrome
Neurovascular sub-syndrome, also known as cerebrovascular (or cardiovascular and central nervous system; CNS) sub-syndrome, occurs when an individual is exposed to >8 or 10 Gy as TBI. Signs and symptoms include severe nausea and vomiting followed by headache, confusion, disorientation, loss of balance, neurologic deficits, and seizures [Citation70]. Localized changes in the central nervous system including impaired capillary circulation, acute inflammation, inflammation of the meninges, interstitial edema, hypertrophy of perivascular astrocytes, damage to the blood–brain barrier, and petechial hemorrhages may denote the neurovascular syndrome [Citation57]. Since injury resulting from such extremely high radiation doses has been considered incurable, scientists have focused their endeavors on finding prophylactic and mitigating therapies for only H-ARS, GI-ARS, pulmonary, and cutaneous sub-syndromes.
While neurovascular syndrome is considered untreatable because it arises from extremely high radiation doses, long-term cognitive dysfunction, following exposure to moderate levels of acute radiation, is a viable area for countermeasure development. NHPs play an important role in the study of radiation-induced cognitive effects because of their close evolutionary relationship to humans. This animal model possesses sophisticated cognitive abilities that other animal models do not. One study exposed male rhesus macaques to 40 Gy (two 5 Gy fractions/wk for 4 wk) to examine the effect of fractionated whole-brain irradiation on cognitive function [Citation71]. Results from this study indicated a significant reduction in higher-order cognitive function after radiation exposure [Citation71]. The report showed that the NHPs exhibited a progressive decline in cognitive performance over the 11-month period. Further, trials conducted using a higher cognitive load demonstrated a more dramatic decline over the period. At 9 months post-irradiation, cognitive tasks were markedly reduced compared to pre-irradiation values [Citation71]. Another study examined the effects of long-term cognitive functioning in rhesus macaques exposed to single TBI (6.75–8.05 Gy) and tested using simple visual discrimination with reversal tasks [Citation72]. Irradiated animals were significantly less likely to engage in at least one task than age-matched, nonirradiated controls. The biggest difference in cognitive performance between irradiated and nonirradiated animals was at the re-reversal phase, suggesting that irradiated animals lacked the cognitive flexibility to learn to adapt to the task’s changing rules. The changes reflected in both studies reflect those reported in brain cancer patients following radiotherapy. In brief, NHP is of critical importance for long-term cognitive dysfunction study following irradiation and for the development of countermeasures.
3.1.4. Pulmonary syndrome
High-dose radiation exposure may cause ARS with GI and/or hematologic morbidity and mortality. In due course of time, survivors of ARS may experience the DEARE, which typically manifest as a chronic illnesses affecting multiple organ systems [Citation73]. Development of DEARE take months and years and usually manifest in the lung as pneumonitis and fibrosis. A better understanding of the mechanisms mediating the long-term ailment that develops in multiple organs after radiation exposure is important for countermeasure development for such indications. The challenges of studying DEARE are complex due to the absence of suitable animal models and essential understanding of the disease and its radiation dose-response relationships.
The lung is a very sensitive organ, and when exposed to radiation, it may demonstrate acute and chronic inflammation that can result in lung fibrosis, an interstitial lung disease that can result in lung scarring that can be fatal [Citation74]. Lung injury manifests a few months following radiation exposure as a delayed effect of irradiation. For example, pneumonitis (lung inflammation) can develop 2–4 months after radiation exposure while fibrosis may occur after 4–6 months [Citation75–Citation78]. Pneumonitis is demonstrated by a loss of epithelial cells, interstitial and airspace edema, and inflammatory infiltrate of macrophages [Citation74]. Radiation exposure immediately triggers a cascade of cellular and molecular changes that occur during the latent period, though clinical indications may not be immediately apparent [Citation79,Citation80]. Development of the clinical features of pulmonary syndrome, for potential countermeasures approach, involves the participation of macrophages, endothelial and epithelial cells, fibroblasts, profibrotic and proinflammatory cytokines as well as the expression of large numbers of genes and transcription factors [Citation6,Citation79].
The NHP model has its advantages and disadvantages for studying radiation-induced pulmonary injury as DEARE. Despite the close similarities of NHPs to humans with respect to their physiology, little information is available regarding radiation-induced pulmonary damage in NHPs [Citation5]. There is a report of delayed lung injury where the NHP thorax was exposed to ionizing radiation. Medical management was provided, and the primary end point was mortality at 180 d post-irradiation. This study yielded the lethal dose response characterized by a LD50/180 of 10.27 Gy and slope of 1.112 probits per linear dose [Citation81]. Despite the paucity of data, the NHP remains the model of choice for investigating pulmonary radiation injury; however, this needs to be further validated for radiation-induced lung injury to better understand the mechanism of damage and cellular/organ responses to the injury [Citation6,Citation82].
For partial body irradiation (PBI), researchers have opted for high-dose exposure in which only a part of the animal is irradiated. Such models include those in which only thorax is irradiated (known as whole-thorax lung irradiation, WTLI) [Citation6].
3.1.5. Cutaneous radiation syndrome
Skin exposure to ionizing radiation greater than 3 Gy may result in a distinct clinical indication that is characterized by a faint and transient erythema followed by blistering, then severe erythema, and ultimately necrosis. Depending on the dose of radiation and severity of injury, necrosis occurs 10–30 d after radiation exposure. In some severe incidents, necrosis may appear within two days. Since cutaneous syndrome runs concurrently as a part of a multiorgan injury, it has not been studied thoroughly. The degree to which multi-organ injury is affected by cutaneous syndrome has been investigated to a limited extent [Citation83].
The cutaneous data from radiation studies in NHPs are limited, and swine have been commonly used as a large animal models to study the cutaneous syndrome of radiation exposure [Citation5]. Though NHPs have been used extensively for TBI and PBI studies, their hirsute nature affects their applicability for cutaneous radiation studies.
In addition to the organ systems discussed above, cardiac and renal systems are significantly affected by the same radiation dose range affecting lung and skin [Citation51]. NHP model has been used to study TBI-induced cardiac and renal injury. Heart disease is an increasingly recognized, serious late effect of radiation exposure. A recent study conducted in NHPs demonstrated that single total-body doses of 6.5–8.4 Gy produced long-term effects including a high incidence of myocardial fibrosis, reduced left ventricular diameter, and elevated systemic inflammation [Citation84]. Also, the kidney has been shown to be one of the most radiosensitive late-responding organs in humans with chronic renal injuries occurring after single doses as low as 4–6 Gy. Histopathological evidence of radiation-induced nephropathy as a result of single-dose exposure with 7.2–8.5 Gy has been demonstrated in rhesus NHPs [Citation85].
3.2. Use of NHPs in the development and FDA approval of radiation countermeasures for ARS
Radioprotectors are prophylactic countermeasures that are administered prior to radiation exposure to prevent radiation-induced damage [Citation57]. Radiation mitigators are agents which are administered shortly after radiation exposure and accelerate repair or recovery of radiation injury. Radiation therapeutics are drugs administered once overt symptoms appear, to stimulate repair or regeneration. Since radiomitigators are effective when administered after radiation exposure, such agents are optimal for mass casualty scenarios.
As discussed above, various macaques have been used for the development of radiation countermeasures for ARS. Rhesus macaques were used in recently reviewed studies to assess the efficacy of neupogen (granulocyte colony-stimulating factor (G-CSF), filgrastim) and neulasta (PEGylated G-CSF, PEGfilgrastim), leading to their FDA approval for H-ARS [Citation19,Citation59,Citation86]. There are several promising radiation countermeasures which are being investigated in NHPs for efficacy () [Citation18,Citation20,Citation21,Citation32–Citation37,Citation39–Citation46,Citation49]. Here, we discussed a few countermeasures that are being investigated in NHP model for efficacy; the majority of known radiation countermeasure studies have observed hematopoietic recovery and survival benefits in countermeasure-treated, irradiated NHPs. In addition, leukine (GM-CSF, sargramostim) has FDA approval for five indications, three of which are for the acceleration of neutrophil recovery in myelosuppressed individuals [Citation87,Citation88]. Although leukine is well recognized to be effective in treating acute, radiation-induced hematopoietic injuries, it has not been fully approved by the FDA for the specific treatment of the ARS indication. A review of the literature showed GM-CSF, unlike G-CSF, to have generally species-specific biological activity. Unlike neupogen and neulasta, leukine showed significant improvement in survival when administered as late as 48 h post-irradiation with minimal supportive care (i.e. single antibiotic (baytril) and without blood products) against two different doses of radiation (6.55 and 7.18 Gy) [Citation20]. HemaMax (a recombinant human IL-12) is under development by Neumedicines as a radiation countermeasure for the treatment of H-ARS and has a pending BLA request to the FDA [Citation24–Citation26,Citation89]. Furthermore, entolimod (CBLB502) is an agonist of toll-like receptor 5 (TLR5) that activates the nuclear factor-κB (NF-κB) signaling pathway. It has been shown to protect NHPs from H- and GI-ARS [Citation22,Citation23]. The preemergency use authorization (EUA) application for CBLB502 is presently pending before the FDA. AEOL 10150 (a meso-porphyrin mimetic) is currently studied as a countermeasure against the pulmonary effects of ARS and the DEARE [Citation90–Citation93]. It has been shown to be a well-tolerated, broad-spectrum catalytic antioxidant capable of extending survival and minimizing acute pathology in NHPs [Citation94]. Recent investigations demonstrated that AEOL 10150 administration reduced lung injury and increased survival after 11.5 Gy of (whole-thorax lung lethal dose (LD100/180)) radiation exposure in the NHP [Citation95]. Results from recent studies demonstrated AEOL 10150 efficacy and biomarkers [Citation29,Citation30]. AEOL 10150 was able to mitigate radiation-induced lung injury and effect was dependent on the schedule of administration. Recilisib Sodium (Ex-RAD, a synthetic chlorobenzylsulfone derivative) is a low-molecular-weight, protein kinase inhibitor. Onconova Therapeutics has recently initiated advanced studies in NHPs with interim results obtained but not yet published. 5-Androstenediol (5-AED)/Neumune was the first radiation countermeasure for ARS to receive FDA IND status. It has been evaluated both as a radioprotector and radiomitigator [Citation27,Citation96]. The radiomitigative efficacy of 5-AED was confirmed in NHPs [Citation96,Citation97]. Gamma-tocotrienol (GT3) aided in the recovery of radiation-induced neutropenia and thrombocytopenia compared to untreated control NHPs. These observations were especially significant after lethal exposures to 5.8 or 6.5 Gy γ-TBI. In addition, a single dose of GT3, without any supportive care, was comparable to multiple doses of neupogen and two doses of neulasta with full supportive care in terms of improving hematopoietic recovery in NHPs. B-190 (indralin) is a small-molecule, adrenomimetic that induces radioprotective effects through activation of α1B-adrenoceptors subtype (a subset of the G-protein-coupled receptors family). This agent has been studied in seven species and has shown consistent efficacy [Citation38,Citation98]. Recently, its efficacy was investigated in NHPs exposed to 6.8 Gy of whole-body γ-radiation [Citation38]. In this study, Indralin protected five out of six NHPs compared to the control group, in which all 10 animals died.
In addition to the above radiation countermeasures, several cytokines, growth factors, and cell therapeutic agents have been used in the NHP model to investigate their radioprotective and radiomitigative potential as presented in [Citation31,Citation43,Citation47,Citation48].
4. Biomarkers for radiation injury and efficacy of radiation countermeasures
The Animal Rule calls for a sound understanding of mechanisms of injury, drug efficacy, and efficacy biomarkers. In this context, it is important to identify biomarkers for radiation injury and countermeasure efficacy that can be used to extrapolate animal efficacy results as well as to select effective dose and regimen in humans. In brief, biomarkers are needed to assess absorbed radiation dose and to serve as reporters for the efficacy of radiation countermeasures [Citation99]. Currently, several biomarkers are approved for specific individual injuries; the FDA has biomarkers for about 150 drug interactions validated, the European Medicines Agency (EMEA) has biomarkers for four injuries approved, and the Pharmaceuticals and Medical Devices Agency (PMDA, Japan) has biomarkers for one injury accepted [Citation100–Citation102]. However, none of these identified biomarkers are for radiation injury.
4.1. Biomarkers to assess absorbed radiation dose
Evaluation of biomarkers needed to assess the absorbed radiation dose is known as biodosimetry. The basic principle of biodosimetry is to exploit the changes in biomarkers induced by radiation exposure, leading to an estimate of the dose of radiation absorbed. This directly predicts the biological consequences of the radiation exposure and helps to explore available treatment options. There are several biomarkers which have been identified and validated using the NHP model, and this effort is continuing. Such studies are briefly discussed below.
4.1.1. Peripheral blood counts
Blood cell counts can serve as robust indicators of absorbed radiation dose. The cells analyzed include neutrophils, white blood cells, lymphocytes, monocytes, and platelets. The correlation exists in the early time window (12–48 h) as well as in the late phase (up to 4 wk) after irradiation [Citation103]. However, for accuracy in absorbed dose assessment, a complete blood cell count should be performed soon after radiation exposure. Blood cell count data from extensive studies with NHPs or samples from humans exposed to known dose of radiation or from radiological accident victims back the use of this test [Citation1,Citation104]. presents the data of average neutrophil, platelet, white blood cell, monocyte, and lymphocyte counts for groups of NHPs that either survived (blue) or died (orange) after exposure to various doses of γ-radiation over time. This figure provides a visual representation of the trends of various peripheral blood parameters of NHPs that survived versus those that died. Presented data suggest that increasing radiation doses increase the severity of neutropenia and thrombocytopenia. In addition, the results suggest that dying versus surviving animals differ when presenting with thrombocytopenia and neutropenia; thrombocytopenia was observed to be a better predictor of mortality from reported data. Furthermore, several radiation countermeasures have demonstrated improvement of blood cell counts in NHPs [Citation19,Citation22–Citation24,Citation26,Citation28,Citation42,Citation59,Citation105]. Based on the above reports, blood components have been used in multiparametric analysis to assess absorbed radiation dose in NHPs as well as in humans [Citation106,Citation107].
Figure 1. Comparison of NHP neutrophils, platelets, WBC, monocytes, and lymphocytes of survivors and non-survivors after exposure to various doses of radiation. NHPs were exposed to 60Co γ-irradiation (5.0, 5.8, 6.5, or 7.2 Gy). Blood was collected at various time points post-radiation, and cells were counted using a Bayer Advia-120 cell counter. The data for each time point are shown as the mean ± standard error for those which survived until 60 d (survivors, blue) and those who succumbed to the effects of radiation before 60 d (non-survivors, orange). It should be noted that all animals surived after exposure to 5.0 Gy radiation.
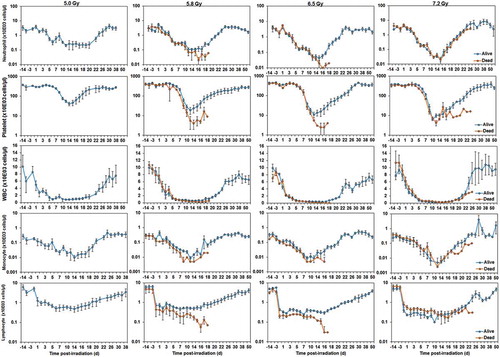
4.1.2. Cytokines, chemokines, and other proteins
The upregulation of G-CSF has been demonstrated in irradiated NHPs and shown to play an important role in mediating radiation injury [Citation22,Citation108]. Additionally, several cytokines, chemokines, and other proteins have been identified as candidate protein biomarkers of radiation injury over the last few years in NHP models () [Citation23,Citation109,Citation110]. Among these proteins are IL-6, C-reactive protein (CRP), serum amyloid A (SAA), growth arrest and DNA damage-inducible 45 (GADD45) proteins, FMS-like tyrosine kinase 3 ligand (FLT3L), and salivary α-amylase. Recently, IL-18 has been reported as a biomarker for radiation injury [Citation111–Citation113]. We evaluated serum samples for IL-18 collected from a large number of NHPs exposed to three different doses of radiation (5.8, 6.5, and 7.2 Gy) at various time points after exposure, though serum from irradiated animals had higher levels of IL-18, it showed no specific correlation with radiation dose and time course [Citation99]. In addition, several proteins such as CRP, amylase, various cytokines, and growth factors have been investigated using NHP models for their possible contributions as biomarkers for radiation injury [Citation99]. However, these molecular biomarkers have large variations due to several factors such as inflammation and infection [Citation106,Citation114].
Figure 2. Radiation-induced changes in NHP cytokines. NHPs were exposed to various doses (5.0, 5.8, 6.5, or 7.2 Gy) of 60Co γ-irradiation. Blood was collected at various time points post-irradiation, and cytokines were analyzed using Multiplex Luminex. The data for each time point are shown as the mean ± standard error for each radiation group. *indicates the time points when the radiation dose dependent first order correlation were significant and when equal variance between groups were assumed (p ≤ 0.05, n = 8 for 5.8, 6.5, 7.2 irradiated groups and n = 4 for the 5.0 Gy radiation group).
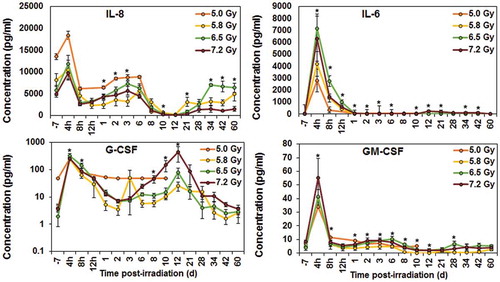
4.1.3. Citrulline
Citrulline has been identified as a biomarker for radiation-induced GI injury and epithelial cell loss; it is a nitrogen end product of glutamine metabolism of enterocytes in the small bowel. Its plasma concentration has been shown to be inversely proportional to GI tissue injury and loss [Citation115,Citation116]. The correlation between epithelial cell loss as a result of radiation-exposure and plasma citrulline level has been well validated in NHPs [Citation11,Citation116–Citation119]. In NHPs exposed to lower doses of radiation (5.8 and 6.5 Gy), data reported showed no reduction in citrulline levels [Citation28]. In this case, enterocyte loss may not have been significant enough to lower citrulline levels. However, exposure to 7.2 Gy of radiation was shown to lower circulating citrulline levels in NHPs.
4.1.4. MicroRNA (miRNA) and long non-coding RNAs (lncRNAs)
miRNA are a conserved, short, noncoding, regulatory RNAs that control gene expression by inducing mRNA cleavage or inhibiting translation. A single miRNA can interact with a large number of mRNAs, thereby regulating diverse cellular processes. Several studies have detected and identified circulating miRNA in the body fluids and tissues of irradiated NHPs as candidate biomarkers [Citation120,Citation121]. There are several advantages to using miRNA as biomarkers: they are relatively stable and small in size, also present in the exosome, their expression levels is altered in response to disease/organ injury, they are tissue specific, and their expression levels can be reproducible in individuals of the same species. These attributes make miRNA an important biomarker.
For example, circulating levels of miR-150-5p have been shown to demonstrate a dose- and time-dependent decrease in the plasma of NHPs. Plasma miR-150-5p levels were found to correlate well with lymphocyte and neutrophil depletion kinetics [Citation122]. Also, miR-574-5p exhibited a dose-dependent increase 24-h post-irradiation in NHPs with lethal versus sub-lethal exposure and returned to the baseline level by d 3. In an elegant study using baboons, miR-342-3p demonstrated a sustained and 10-fold down-regulation on d 1 and 2 after irradiation [Citation13]. In addition, a recent study showed that seven microRNAs are altered by irradiation in NHPs (mir-150, miR-215, miR-30A, miR-126, miR-133a, miR-375, and miR-133b) [Citation123]. Furthermore, the genomic studies revealed that there is a combination of seven transcription factors that are predicted to regulate these miRNAs in mice, NHPs, and humans. This study showed that a combination of three microRNAs (miR-133b, miR-215, and miR-375) can be used to identify NHPs exposed to radiation versus unexposed NHPs, and that females appeared to be more sensitive to radiation. Also, a classifier based on two microRNAs (miR-30a and miR-126) that can predict radiation-induced mortality was developed from this work; and thus established a 5-microRNA composite signature that can identify irradiated individuals and predict their probability of survival. Concisely, this research effort demonstrated that miRNA can be predictive of radiation exposure and can be used to assess radiation exposure in the context of a mass casualty scenario.
Long noncoding RNAs (lncRNAs) are mRNA-like transcripts that do not code for protein but are present in about 80% of transcriptions [Citation124]. lncRNAs are divided into five classes: sense, antisense, bidirectional, intronic, and intergenic. This field may hold promise for future biodosimetry as more lncRNA are identified as radiation biomarkers [Citation125].
4.1.5. Physical dosimeters
Generation of free radicals in response to radiation exposure induces oxidative stress and results in damage to DNA, proteins, and lipids. In most of the biological tissues, the life span of these unpaired electrons is nanoseconds. Such signals induced as a result of radiation exposure can be fixed for extended periods in calcified tissues and can later be detected by electron paramagnetic resonance (EPR). EPR-based radiation dosimetry is a non- or minimally-invasive technique without the need for conventional tissue processing like other organ dosimetry methods. Although free radicals can be incorporated into tooth enamel, hair, and α-keratin of fingernails [Citation126] and the EPR spectroscopy is well established for measuring free radicals in biological samples; nevertheless, the clinical application of EPR for human dosimetry still needs additional work [Citation127,Citation128]. Moreover, EPR is relevant for only certain types of exposure and cannot be used to detect exposure to neutrons or internalized radioisotopes.
4.1.6. Additional important biomarkers
In spite of the biomarkers discussed above, the need remains for the identification and validation of novel, robust, and stable biomarkers that are sensitive to radiation and can be evaluated in a minimally invasive or noninvasive manner. In the recent past, radiation biologists have redirected their efforts to investigate chemical alternatives in biological fluid metabolome, biological alternatives in the gut microbiome, and other molecules of interest. There are several studies identifying proteins [Citation129,Citation130], metabolites as biomarkers for radiation exposure in blood [Citation131–Citation133], urine [Citation131,Citation134,Citation135], and the gut using NHP models [Citation135–Citation139]. In addition, there are ample studies with lipidomics [Citation132], microbiome [Citation140], and mRNA [Citation141]. Considering space limitations for this article, it is not possible to discuss this aspect in depth and readers may go through recent reviews [Citation99,Citation137].
4.2. Biomarkers for radiation countermeasure efficacy
The Animal Rule requires the establishment of human drug dose based on biomarker-guided bioequivalence of effective animal dose. Thus, biomarkers are an important aspect of ARS radiation countermeasure development and can be used as a trigger for intervention as well as in selecting a drug dose and treatment regimen in humans. Biomarkers may also be used to correlate with the mechanism by which the radiation countermeasure ameliorates the injury or to correlate with the desired clinical result (reduction in morbidity and mortality). Three microRNAs (miR-30a, miR-126, and miR-375) have recently been demonstrated as biomarkers for the radioprotective efficacy of γ-tocotrienol as a countermeasure [Citation123].
To estimate the doses of countermeasures required to yield therapeutic advantage in humans, one needs to use drug-induced biomarker responses as proxies for agent efficacy. Thus, the human efficacious dose for a radiation countermeasure is defined as the drug dose that activates the same biomarker responses to the same degree in humans as those that accompany and mediate the drug’s therapeutic benefit in animals. A limited number of biomarkers discussed above for the assessment of absorbed radiation doses have been used for various countermeasure development efforts in NHPs [Citation19,Citation22–Citation24,Citation26,Citation28,Citation42,Citation59,Citation99,Citation105].
5. Conclusion
Animal models are required to provide a better understanding of the basic mechanisms of radiation injury which will be valuable for designing unique classes of countermeasures for ARS. The NHP model, favored by the Animal Rule requirements, offers an avenue for both acute and long-term studies of multiple organ syndromes or late/delayed effects and is probably the most appropriate model for predicting effects of radiation exposure on the human body. NHPs are needed for preclinical development due to the similarities of drug pharmacokinetics/metabolism and physiology between humans and NHPs. Earlier experience has demonstrated that canine pharmacokinetics are not always translatable to primate efficacy [Citation142]. Accordingly, the NHP model is usually the large animal species of choice for toxicity, pharmacokinetics, biomarker, radiation injury, and countermeasure efficacy.
The Animal Rule imposes the additional burden on investigators to establish the effectiveness of drug candidate in more than one animal species that sufficiently represents and predicts ARS in humans. In cases where a single animal model is well characterized and proven to be predictive, the Animal Rule does not require that additional animal models be used; although, this is unlikely to occur when assessing radiation countermeasures for efficacy. It is important to note that as long as the mechanisms of ARS and DEARE have not been completely understood, more species will be required for use. Since this regulatory pathway is exceptionally used, the FDA offers only general guidelines for steering this regulatory mechanism. Accessibility of suitable animal models is one of the constraining factors in developing countermeasures using the Animal Rule. Development of adequate large animal models will facilitate and also expedite the development and approval of radiation countermeasures for ARS currently being investigated and newly identified.
We have discussed the suitability of NHPs as animal models for the development of radiation countermeasures for ARS based on similarity between NHP and human pathophysiology. Published results investigating NHP and human clinical parameters, histopathological findings, and immune response have demonstrated similarities. The NHP model is considered the most reliable predictor of therapeutic efficacy of drugs in humans, and this model is most likely to satisfy the Animal Rule requirements for radiation countermeasure approval by the FDA. The radiomitigative potential of two FDA-approved radiomitigators for H-ARS was investigated in the NHP model [Citation19,Citation59]. Although the NHP model has been reasonably characterized for H-ARS, additional effort is needed to further characterize this animal model for various sub-syndromes of ARS.
6. Expert opinion
As stated above, only two radiomitigators have been approved by the FDA for H-ARS [Citation143,Citation144]. These are repurposed drugs, neupogen and neulasta, from their original therapeutic indications to new treatment for H-ARS indication. Both drugs are growth factors already in clinical use extensively for other related indications such as chemotherapy- and radiotherapy-induced neutropenia as well as for mobilization of stem cells for stem cell transplant patients, specifically for donors undergoing plasmapheresis [Citation104]. Also, Neupogen was already in the Strategic National Stockpile based on its pre-EUA status. The number of fully approved radiation countermeasures for human use remains remarkably limited. Nevertheless, the basic research component is progressing well with the continuous production of new and promising agents. It may just be that the problem lies at the other end of the drug development process: the complexity and burdensome nature of the regulatory approval process is often referred to as a ‘drawback.’ More likely, the ‘transitional stages of drug development’ are the major logjams and are rate limiting in terms of taking a new drug from the bench-top to the trading floor. Transitioning a new radiation countermeasure from the initial preclinical evaluation (using both in vitro tests and small animal models for efficacy and toxicology assessments) to later preclinical or early clinical investigation (using appropriate large animal models) requires an experienced class of sponsor, one with sufficient resources available to carry out the crucial and mandated investigations. Very often, promising and exciting new drugs, fail to see the light-of-day because of a lack of appropriate transitioning through various stages of the research and development pathway. Furthermore, there are agents (e.g. the angiotensin-converting enzyme inhibitors) that are approved for human use, and for which there is laboratory and clinical evidence for countermeasure efficacy but such agents are not developed as they are off-patent. Though repurposing of already-approved agents is getting attention recently, the lack of a developmental path for such approved agents is a barrier to practical development of radiation countermeasures. Additional support from private organizations, academic institutions, and government agencies to fully resourced and capable pharmaceutical sponsors that are capable of handling these critical transitional stages would go a long way to relieve the current impasse and deficits in available safe and effective radiation countermeasures for future radiological and nuclear contingencies.
No radiation countermeasure has yet been approved by the FDA for use as a radioprotector (agents which can be used prior to radiation exposure and provide benefit) for H-ARS following the Animal Rule; both recently approved radiation countermeasures are radiomitigators (to be used after exposure to radiation). Amifostine has been approved by FDA for limited indications (mucositis and renal toxicity as a result of radiotherapy and chemotherapy) due to its toxicity at doses required to treat ARS [Citation145]. Another radioprotector which has FDA approval for limited indication is palifermin [Citation145]. In addition, no radiation countermeasure has been approved for GI-ARS, either as a radioprotector or radiomitigator. There are several reasons for such failure and one notable reason is the shortage of multiple large animal models suitable to sufficiently represent the pathophysiology of humans for investigating radiation injury. Further, the mechanism of radiation injury is not fully understood, and there is toxicity associated with radiation countermeasures currently under development. The advanced development and FDA approval of radiation countermeasures for ARS requires demonstration that the mechanism of action of such countermeasures in animal models is predictive of protective response in humans. The Animal Rule highlights the need for a good understanding of pathophysiology of radiation injury as well as the underpinning mechanism of action of radiation countermeasures under investigation. In addition, it requires the identification and validation of biomarkers to serve as a predictive marker for the efficacy in humans and for drug dose conversion (animal to human, beyond allometric conversion). This is particularly important because countermeasures will not be clinically investigated for their efficacy under phase II and III due to ethical consideration. Accomplishing the above tasks to advance ARS countermeasures for FDA approval following the Animal Rule is not easy. As a result, several countermeasures at advanced stages of development become stagnant for a long time. Due to such impediments, corporate partners sometimes lose interest for developing such countermeasures. The above concerns highlight the necessity for a combined endeavor between corporate partners, academia, and government agencies. All parties involved in the development of radiation countermeasures may learn from a few successes of medical countermeasures development partnership and FDA approval process for biological threats. Although NHP models of human diseases are generally considered the gold standard for drug development following FDA regulation, given that NHPs are the closest animal species to humans, additional work is still needed to characterize this model for ARS, delayed, late, and chronic effects of radiation.
Article highlights
Radiation countermeasures for ARS are being developed following the FDA Animal Rule because human efficacy studies are unethical and unfeasible being that the etiologic agent of ARS is the radiation damage to cells and tissues over time.
For the Animal Rule, the FDA stresses the selection of a sufficiently well-characterized animal model with good understanding of the pathophysiology of injury for adequate and well-controlled animal studies when the results of those animal studies are used for predicting the response in humans.
For studying radiation injury and ARS, only two large animal the models (NHP and canine) have been well characterized. Lately, minipig model is being characterized.
NHP represents the preferred animal models for the efficacy testing of countermeasures for ARS because NHP data provide a gold standard that frequently cannot be adequately achieved from alternative models. NHP provides 95+% DNA sequence homology with humans, and a high level of similarity in terms of response to physiological pathways and cell receptors.
Two radiation countermeasures (Neupogen and Neulasta) for H-ARS have been approved by FDA based on efficacy data generated in NHP model following Animal Rule. A third radiation countermeasure (Leukine) for H-ARS may be approved by FDA soon following the Animal Rule.
Additional studies are needed to characterize various sub-syndromes and organ involvement in NHPs as a result of radiation exposure. Also, there is a need to further investigate acute, delayed, late, and chronic effects of radiation exposure in NHP model.
This box summarizes key points contained in the article
Declaration of interest
The authors have no relevant affiliations or financial involvement with any non-governmental entities or organization, nor have any financial conflict with the subject matter or materials discussed in the manuscript. This includes employment, consultancies, honoraria, stock ownership or options, expert testimony, grants or patents received or pending, or royalties.
Acknowledgments
The opinions or assertions expressed herein are those of the authors and are not endorsements from Armed Forces Radiobiology Research Institute, Uniformed Services University of the Health Sciences, or the US Department of Defense. The authors are thankful to Paola Santiago, Melissa Garcia, Briana Hanlon, and Eric Lee for graphics, literature searches, and editorial assistance.
Additional information
Funding
References
- Singh VK, Newman VL, Berg AN, et al. Animal models for acute radiation syndrome drug discovery. Expert Opin Drug Discov. 2015;10:497–517. .
- Kim JW, Lee DW, Choi WH, et al. Development of a porcine skin injury model and characterization of the dose-dependent response to high-dose radiation. J Radiat Res. 2013;54:823–831.
- King GL, Rabin BM, Weatherspoon JK. 5-HT3 receptor antagonists ameliorate emesis in the ferret evoked by neutron or proton radiation. Aviat Space Environ Med. 1999;70:485–492.
- Lankau EW, Turner PV, Mullan RJ, et al. Use of nonhuman primates in research in North America. J Am Assoc Lab Anim Science. 2014;53:278–282.
- Williams JP, Brown SL, Georges GE, et al. Animal models for medical countermeasures to radiation exposure. Radiat Res. 2010;173:557–578.
- Williams JP, Jackson IL, Shah JR, et al. Animal models and medical countermeasures development for radiation-induced lung damage: report from an NIAID Workshop. Radiat Res. 2012;177:e0025–39. .
- Augustine AD, Gondre-Lewis T, McBride W, et al. Animal models for radiation injury, protection and therapy. Radiat Res. 2005;164:100–109.
- Flynn JL, Capuano SV, Croix D, et al. Non-human primates: a model for tuberculosis research. Tuberculosis (Edinb). 2003;83:116–118.
- Mahmud N, Pang W, Cobbs C, et al. Studies of the route of administration and role of conditioning with radiation on unrelated allogeneic mismatched mesenchymal stem cell engraftment in a nonhuman primate model. Exp Hematol. 2004;32:494–501.
- Norol F, Drouet M, Mathieu J, et al. Ex vivo expanded mobilized peripheral blood CD34+ cells accelerate haematological recovery in a baboon model of autologous transplantation. Br J Haematol. 2000;109:162–172.
- Herodin F, Richard S, Grenier N, et al. Assessment of total- and partial-body irradiation in a baboon model: preliminary results of a kinetic study including clinical, physical, and biological parameters. Health Phys. 2012;103:143–149.
- Herodin F, Mestries JC, Janodet D, et al. Recombinant glycosylated human interleukin-6 accelerates peripheral blood platelet count recovery in radiation-induced bone marrow depression in baboons. Blood. 1992;80:688–695.
- Port M, Herodin F, Valente M, et al. MicroRNA expression for early prediction of late occurring hematologic acute radiation syndrome in baboons. PLoS One. 2016;11:e0165307.
- Port M, Herodin F, Valente M, et al. First generation gene expression signature for early prediction of late occurring hematological acute radiation syndrome in baboons. Radiat Res. 2016;186:39–54.
- Valente M, Denis J, Grenier N, et al. Revisiting biomarkers of total-body and partial-body exposure in a baboon model of irradiation. PLoS One. 2015;10:e0132194.
- Port M, Herodin F, Valente M, et al. Gene expression signature for early prediction of late occurring pancytopenia in irradiated baboons. Ann Hematol. Forthcoming 2017.
- Port M, Herodin F, Valente M, et al. Pre-exposure gene expression in baboons with and without pancytopenia after radiation exposure. Int J Mol Sci. 2017;18:E541.
- Farese AM, MacVittie TJ. Filgrastim for the treatment of hematopoietic acute radiation syndrome. Drugs Today (Barc). 2015;51:537–548.
- Hankey KG, Farese AM, Blaauw EC, et al. Pegfilgrastim improves survival of lethally irradiated nonhuman primates. Radiat Res. 2015;183:643–655. .
- Clayton NP, Charpentier EJJ, LaCasse ER, et al. Sargramostim significantly improved the mortality rate at Day 60 in a non-human primate model of hematopoietic acute radiation syndrome with minimal supportive care when administered 48 h after total body irradiation. 42nd Conference of the European Radiation Reasearch Society; 2016; Amsterdam, Netherlands; p. 190
- Farese AM, Williams DE, Seiler FR, et al. Combination protocols of cytokine therapy with interleukin-3 and granulocyte-macrophage colony-stimulating factor in a primate model of radiation-induced marrow aplasia. Blood. 1993;82:3012–3018.
- Krivokrysenko VI, Shakhov AN, Singh VK, et al. Identification of granulocyte colony-stimulating factor and interleukin-6 as candidate biomarkers of CBLB502 efficacy as a medical radiation countermeasure. J Pharmacol Exp Ther. 2012;343:497–508. .
- Krivokrysenko VI, Toshkov IA, Gleiberman AS, et al. The Toll-like receptor 5 agonist Entolimod mitigates lethal acute radiation syndrome in non-human primates. Plos One. 2015;10:e0135388.
- Gluzman-Poltorak Z, Mendonca SR, Vainstein V, et al. Randomized comparison of single dose of recombinant human IL-12 versus placebo for restoration of hematopoiesis and improved survival in rhesus monkeys exposed to lethal radiation. J Hematol Oncol. 2014;7:31.
- Basile LA, Ellefson D, Gluzman-Poltorak Z, et al. HemaMax, a recombinant human interleukin-12, is a potent mitigator of acute radiation injury in mice and non-human primates. Plos One. 2012;7:e30434. .
- Gluzman-Poltorak Z, Vainstein V, Basile LA. Recombinant interleukin-12, but not granulocyte-colony stimulating factor, improves survival in lethally irradiated nonhuman primates in the absence of supportive care: evidence for the development of a frontline radiation medical countermeasure. Am J Hematol. 2014;89:868–873.
- Stickney DR, Dowding C, Authier S, et al. 5-androstenediol improves survival in clinically unsupported rhesus monkeys with radiation-induced myelosuppression. Int Immunopharmacol. 2007;7:500–505. .
- Singh VK, Kulkarni S, Fatanmi OO, et al. Radioprotective efficacy of gamma-tocotrienol in nonhuman primates. Radiat Res. 2016;185:285–298.
- MacVittie TJ, Gibbs A, Farese AM, et al. AEOL 10150 mitigates radiation-induced lung injury in the nonhuman primate: morbidity and mortality are administration schedule-dependent. Radiat Res. 2017;187:298–318.
- Carter CL, Jones JW, Barrow K, et al. A MALDI-MSI approach to the characterization of radiation-induced lung injury and medical countermeasure development. Health Phys. 2015;109:466–478.
- Faller DV, Castaneda SA, Zhou D, et al. An oral HemokineTM, alpha-methylhydrocinnamate, enhances myeloid and neutrophil recovery following irradiation in vivo. Blood Cells Mol Dis. 2017;63:1–8.
- Herodin F, Roy L, Grenier N, et al. Antiapoptotic cytokines in combination with pegfilgrastim soon after irradiation mitigates myelosuppression in nonhuman primates exposed to high irradiation dose. Exp Hematol. 2007;35:1172–1181.
- Drouet M, Mourcin F, Grenier N, et al. Single administration of stem cell factor, FLT-3 ligand, megakaryocyte growth and development factor, and interleukin-3 in combination soon after irradiation prevents nonhuman primates from myelosuppression: long-term follow-up of hematopoiesis. Blood. 2004;103:878–885.
- Zeidler C, Kanz L, Hurkuck F, et al. In vivo effects of interleukin-6 on thrombopoiesis in healthy and irradiated primates. Blood. 1992;80:2740–2745.
- MacVittie TJ, Farese AM, Patchen ML, et al. Therapeutic efficacy of recombinant interleukin-6 (IL-6) alone and combined with recombinant human IL-3 in a nonhuman primate model of high-dose, sublethal radiation-induced marrow aplasia. Blood. 1994;84:2515–2522.
- Chen BJ, Deoliveira D, Spasojevic I, et al. Growth hormone mitigates against lethal irradiation and enhances hematologic and immune recovery in mice and nonhuman primates. PLoS One. 2010;5:e11056.
- Farese AM, Smith WG, Giri JG, et al. Promegapoietin-1a, an engineered chimeric IL-3 and Mpl-L receptor agonist, stimulates hematopoietic recovery in conventional and abbreviated schedules following radiation-induced myelosuppression in nonhuman primates. Stem Cells. 2001;19:329–338.
- Vasin MV, Semenov LF, Suvorov NN, et al. Protective effect and the therapeutic index of indralin in juvenile rhesus monkeys. J Radiat Res. 2014;55:1048–1055.
- Laver J, Abboud M, Gasparetto C, et al. Effects of IL-1 on hematopoietic progenitors after myelosuppressive chemoradiotherapy. Biotherapy. 1989;1:293–300.
- Berenson RJ, Andrews RG, Bensinger WI, et al. Antigen CD34+ marrow cells engraft lethally irradiated baboons. J Clin Invest. 1988;81:951–955.
- Farese AM, Casey DB, Smith WG, et al. Leridistim, a chimeric dual G-CSF and IL-3 receptor agonist, enhances multilineage hematopoietic recovery in a nonhuman primate model of radiation-induced myelosuppression: effect of schedule, dose, and route of administration. Stem Cells. 2001;19:522–533.
- Farese AM, Casey DB, Vigneulle RM, et al. A single dose of pegylated leridistim significantly improves neutrophil recovery in sublethally irradiated rhesus macaques. Stem Cells. 2001;19:514–521.
- MacVittie TJ, Farese AM, Smith WG, et al. Myelopoietin, an engineered chimeric IL-3 and G-CSF receptor agonist, stimulates multilineage hematopoietic recovery in a nonhuman primate model of radiation-induced myelosuppression. Blood. 2000;95:837–845.
- Farese AM, Hunt P, Grab LB, et al. Combined administration of recombinant human megakaryocyte growth and development factor and granulocyte colony-stimulating factor enhances multilineage hematopoietic reconstitution in nonhuman primates after radiation-induced marrow aplasia. J Clin Invest. 1996;97:2145–2151.
- Farese AM, Herodin F, McKearn JP, et al. Acceleration of hematopoietic reconstitution with a synthetic cytokine (SC-55494) after radiation-induced bone marrow aplasia. Blood. 1996;87:581–591.
- MacVittie TJ, Farese AM, Herodin F, et al. Combination therapy for radiation-induced bone marrow aplasia in nonhuman primates using synthokine SC-55494 and recombinant human granulocyte colony-stimulating factor. Blood. 1996;87:4129–4135.
- Ma YC, Li M, Xing S, et al. [Therapeutic effect of combined cytokines on nonhuman primate model of severe haemopoietic acute radiation sickness]. Zhongguo Shi Yan Xue Ye Xue Za Zhi. 2016;24:573–579.
- Wagemaker G, Neelis KJ, Hartong SCC, et al. The efficacy of recombinant TPO in murine And nonhuman primate models for myelosuppression and stem cell transplantation. Stem Cells. 1998;16(Suppl 2):127–141.
- Farese AM, MacVittie TJ, Roskos L, et al. Hematopoietic recovery following autologous bone marrow transplantation in a nonhuman primate: effect of variation in treatment schedule with PEG-rHuMGDF. Stem Cells. 2003;21:79–89.
- U.S. Food and Drug Administration. Guidance for industry: product developoment under the animal rule. 2015. [cited 2016 Jul 5]. Available from: http://www.fda.gov/downloads/Drugs/GuidanceComplianceRegulatoryInformation/Guidances/UCM399217.pdf
- Hall EJ, Giaccia AJ. Radiobiology for the radiobiologist. 7th ed. Philadelphia (PA): Lippincott Williams and Wilkins; 2012.
- Bushberg JT. Radiation exposure and contamination - Merck manual for health care professionals. Rahway (NJ): Merck Publishing Group; 2016.
- McCann DGC. Radiation poisoning: current concepts in the acute radiation syndrome. Am J Clin Med. 2006;3:13–21.
- Fliedner TM, Dorr DH, Meineke V. Multi-organ involvement as a pathogenetic principle of the radiation syndromes: a study involving 110 case histories documented in SEARCH and classified as the bases of haematopoietic indicators of effect. Br J Radiol Suppl. 2005;27:1–8.
- Hill RP. Radiation effects on the respiratory system. Br J Radiol Suppl. 2005;27:75–81.
- Moulder JE, Cohen EP. Radiation-induced multi-organ involvement and failure: the contribution of radiation effects on the renal system. Br J Radiol Suppl. 2005;27:82–88.
- Stone HB, Moulder JE, Coleman CN, et al. Models for evaluating agents intended for the prophylaxis, mitigation and treatment of radiation injuries. Report of an NCI Workshop, December 3-4, 2003. Radiat Res. 2004;162:711–728. .
- Dumont F, Le Roux A, Bischoff P. Radiation countermeasure agents: an update. Expert Opin Ther Pat. 2010;20:73–101. .
- Farese AM, Cohen MV, Katz BP, et al. Filgrastim improves survival in lethally irradiated nonhuman primates. Radiat Res. 2013;179:89–100. .
- Farese AM, Cohen MV, Katz BP, et al. A nonhuman primate model of the hematopoietic acute radiation syndrome plus medical management. Health Phys. 2012;103:367–382.
- MacVittie TJ, Farese AM, Bennett A, et al. The acute gastrointestinal subsyndrome of the acute radiation syndrome: a rhesus macaque model. Health Phys. 2012;103:411–426. .
- Geraci JP, Jackson KL, Mariano MS. The intestinal radiation syndrome: sepsis and endotoxin. Radiat Res. 1985;101:442–450.
- Carr KE. Effects of radiation damage on intestinal morphology. Int Rev Cytol. 2001;208:1–119.
- Mason KA, Withers HR, McBride WH, et al. Comparison of the gastrointestinal syndrome after total-body or total-abdominal irradiation. Radiat Res. 1989;117:480–488.
- Krimsky M, Dagan A, Aptekar L, et al. Assessment of intestinal permeability in rats by permeation of inulin-fluorescein. J Basic Clin Physiol Pharmacol. 2000;11:143–153.
- Kobayashi T, Ohmori T, Yanai M, et al. The analysis of the defense mechanism against indigenous bacterial translocation in X-irradiated mice. Microbiol Immunol. 1991;35:315–324.
- Lutgens LC, Blijlevens NM, Deutz NE, et al. Monitoring myeloablative therapy-induced small bowel toxicity by serum citrulline concentration: a comparison with sugar permeability tests. Cancer. 2005;103:191–199.
- Lang IM, Sarna SK, Condon RE. Gastrointestinal motor correlates of vomiting in the dog: quantification and characterization as an independent phenomenon. Gastroenterology. 1986;90:40–47.
- Danquechin Dorval E, Mueller GP, Eng RR, et al. Effect of ionizing radiation on gastric secretion and gastric motility in monkeys. Gastroenterology. 1985;89:374–380.
- Lopez M, Martin M. Medical management of the acute radiation syndrome. Rep Pract Oncol Radiother. 2011;16:138–146.
- Robbins ME, Bourland JD, Cline JM, et al. A model for assessing cognitive impairment after fractionated whole-brain irradiation in nonhuman primates. Radiat Res. 2011;175:519–525.
- Hanbury DB, Peiffer AM, Dugan G, et al. Long-term cognitive functioning in single-dose total-body gamma-irradiated rhesus monkeys (Macaca mulatta). Radiat Res. 2016;186:447–454.
- MacVittie TJ. The MCART consortium animal model series: MCART animal model refinement and MCM development: defining organ dose, organ-specific tissue imaging, model validation and the natural history between the acute radiation syndrome (ARS) and the delayed effects of acute radiation exposure (DEARE). Health Phys. 2015;109:335–341.
- Travis EL. Organizational response of normal tissues to irradiation. Semin Radiat Oncol. 2001;11:184–196.
- Marks LB, Yu X, Vujaskovic Z, et al. Radiation-induced lung injury. Semin Radiat Oncol. 2003;13:333–345.
- Sharplin J, Franko AJ. A quantitative histological study of strain-dependent differences in the effects of irradiation on mouse lung during the intermediate and late phases. Radiat Res. 1989;119:15–31.
- Sharplin J, Franko AJ. A quantitative histological study of strain-dependent differences in the effects of irradiation on mouse lung during the early phase. Radiat Res. 1989;119:1–14.
- McLaughlin RF Jr., Tyler WS, Canada RO. Subgross pulmonary anatomy of the rabbit, rat, and guinea pig, with additional notes on the human lung. Am Rev Respir Dis. 1966;94:380–387.
- Vujaskovic Z, Marks LB, Anscher MS. The physical parameters and molecular events associated with radiation-induced lung toxicity. Semin Radiat Oncol. 2000;10:296–307.
- Haston CK, Zhou X, Gumbiner-Russo L, et al. Universal and radiation-specific loci influence murine susceptibility to radiation-induced pulmonary fibrosis. Cancer Res. 2002;62:3782–3788.
- Garofalo M, Bennett A, Farese AM, et al. The delayed pulmonary syndrome following acute high-dose irradiation: a rhesus macaque model. Health Phys. 2014;106:56–72.
- DiCarlo AL, Jackson IL, Shah JR, et al. Development and licensure of medical countermeasures to treat lung damage resulting from a radiological or nuclear incident. Radiat Res. 2012;177:717–721.
- Peter RU. Cutaneous radiation syndrome in multi-organ failure. BJR Suppl. 2005;27:180–184.
- DeBo RJ, Lees CJ, Dugan GO, et al. Late effects of total-body gamma irradiation on cardiac structure and function in male rhesus macaques. Radiat Res. 2016;186:55–64.
- van Kleef EM, Zurcher C, Oussoren YG, et al. Long-term effects of total-body irradiation on the kidney of Rhesus monkeys. Int J Radiat Biol. 2000;76:641–648.
- Singh VK, Romaine PL, Newman VL, et al. Medical countermeasures for unwanted CBRN exposures: part II radiological and nuclear threats with review of recent countermeasure patents. Expert Opin Ther Pat. 2016;26:1399–1408.
- Sanofi-aventis U.S. LLC. Leukine. 2013. [cited 2016 Oct 20]. Available from: http://products.sanofi.us/Leukine/Leukine.html
- Gupta S. FDA advisory committee meeting: study drug Leukine. 2013. [cited 2016 Oct 2]. Available from: http://www.fda.gov/downloads/advisorycommittees/committeesmeetingmaterials/drugs/medicalimagingdrugsadvisorycommittee/ucm350156.pdf
- Neumedicines. HemaMax™ (rHuIL-12) for acute radiation syndrome. 2014. [cited 2014 Oct 27]. Available from: http://www.neumedicines.com/
- Gridley DS, Makinde AY, Luo X, et al. Radiation and a metalloporphyrin radioprotectant in a mouse prostate tumor model. Anticancer Res. 2007;27:3101–3109.
- Pearlstein RD, Higuchi Y, Moldovan M, et al. Metalloporphyrin antioxidants ameliorate normal tissue radiation damage in rat brain. Int J Radiat Biol. 2010;86:145–163.
- Zhang Y, Zhang X, Rabbani ZN, et al. Oxidative stress mediates radiation lung injury by inducing apoptosis. Int J Radiat Oncol Biol Phys. 2012;83:740–748.
- Batinic-Haberle I, Tovmasyan A, Roberts E, et al. SOD therapeutics: latest insights into their structure-activity relationships and impact upon the cellular redox-based pathways. Antioxid Redox Signal. 2013;20:2372–2415.
- Orrell RW. AEOL-10150 (Aeolus). Curr Opin Investig Drugs. 2006;7:70–80.
- Garofalo MC, Ward AA, Farese AM, et al. A pilot study in rhesus macaques to assess the treatment efficacy of a small molecular weight catalytic metalloporphyrin antioxidant (AEOL 10150) in mitigating radiation-induced lung damage. Health Phys. 2014;106:73–83.
- Stickney DR, Dowding C, Garsd A, et al. 5-androstenediol stimulates multilineage hematopoiesis in rhesus monkeys with radiation-induced myelosuppression. Int Immunopharmacol. 2006;6:1706–1713.
- Singh VK, Newman VL, Romaine PL, et al. Radiation countermeasure agents: an update (2011-2014). Expert Opin Ther Pat. 2014;24:1229–1255. .
- Vasin MV, Antipov VV, Chernov GA, et al. Studies of the radiation-protective effects of indralin on the hematopoietic system of different species of animals. Radiats Biol Radioecol. 1996;36:168–189.
- Singh VK, Newman VL, Romaine PL, et al. Use of biomarkers for assessing radiation injury and efficacy of countermeasures. Expert Rev Mol Diagn. 2016;16:65–81. .
- U.S. Food and Drug Administration. Table of pharmacogenomic biomarkers in drug labeling. 2015. [cited 2015 Oct 25]. Available from: http://www.fda.gov/drugs/scienceresearch/researchareas/pharmacogenetics/ucm083378.htm
- European Medicines Agency. Qualification of novel methodologies for medicine development. 2015. [cited 2015 Oct 25]. Available from: http://www.ema.europa.eu/ema/index.jsp?curl=pages/regulation/document_listing/document_listing_000319.jsp&mid=WC0b01ac0580022bb0
- Pharmaceutical and Medical Devices Agency. Record of consultations on pharmacogenomics/biomarkers. 2010. [cited 2015 Oct 25]. Available from: https://www.pmda.go.jp/english/review-services/consultations/0001.html
- Hu S, Blakely WF, Cucinotta FA. HEMODOSE: a biodosimetry tool based on multi-type blood cell counts. Health Phys. 2015;109:54–68.
- Singh VK, Newman VL, Seed TM. Colony-stimulating factors for the treatment of the hematopoietic component of the acute radiation syndrome (H-ARS): a review. Cytokine. 2015;71:22–37.
- Gluzman-Poltorak Z, Vainstein V, Basile LA. Association of hematological nadirs and survival in a nonhuman primate model of hematopoietic syndrome of acute radiation syndrome. Radiat Res. 2015;184:226–230.
- Blakely WF, Ossetrova NI, Whitnall MH, et al. Multiple parameter radiation injury assessment using a nonhuman primate radiation model-biodosimetry applications. Health Phys. 2010;98:153–159.
- Blakely WF, Madrid JP, Sandgren DJ. Biodosimetry medical recording-use of the biodosimetry assessment tool. Health Phys. 2010;99(Suppl 5):S184–191.
- Singh VK, Fatanmi OO, Singh PK, et al. Role of radiation-induced granulocyte colony-stimulating factor in recovery from whole body gamma-irradiation. Cytokine. 2012;58:406–414.
- Ossetrova NI, Sandgren DJ, Blakely WF. Protein biomarkers for enhancement of radiation dose and injury assessment in nonhuman primate total-body irradiation model. Radiat Prot Dosimetry. 2014;159:61–76.
- Redon CE, Nakamura AJ, Martin OA, et al. Recent developments in the use of gamma-H2AX as a quantitative DNA double-strand break biomarker. Aging (Albany NY). 2011;3:168–174.
- Ha CT, Li X, Fu D, et al. Circulating IL-18 binding protein (IL-18BP) and IL-18 as dual biomarkers of total-body irradiation in mice. Radiat Res. 2016;185:375–383.
- Xiao M. The role of proinflammatory cytokine interleukin-18 in radiation injury. Health Phys. 2016;111:212–217.
- Ha CT, Li X-H, Fu D, et al. Circulating interleukin-18 as a biomarker of total-body radiation exposure in mice, minipigs, and nonhuman primates (NHP). PLoS One. 2014;9:e109249.
- Moroni M, Lombardini E, Salber R, et al. Hematological changes as prognostic indicators of survival: similarities between Gottingen minipigs, humans, and other large animal models. PLoS One. 2011;6:e25210.
- Bujold K, Hauer-Jensen M, Donini O, et al. Citrulline as a biomarker for gastrointestinal-acute radiation syndrome: species differences and experimental condition effects. Radiat Res. 2016;186:71–78.
- Jones JW, Bennett A, Carter CL, et al. Citrulline as a biomarker in the non-human primate total- and partial-body irradiation models: correlation of circulating citrulline to acute and prolonged gastrointestinal injury. Health Phys. 2015;109:440–451.
- Wang J, Shao L, Hendrickson HP, et al. Total body irradiation in the “hematopoietic” dose range induces substantial intestinal injury in non-human primates. Radiat Res. 2015;184:545–553.
- Jones JW, Tudor G, Bennett A, et al. Development and validation of a LC-MS/MS assay for quantitation of plasma citrulline for application to animal models of the acute radiation syndrome across multiple species. Anal Bioanal Chem. 2014;406:4663–4675.
- Jones JW, Scott AJ, Tudor G, et al. Identification and quantitation of biomarkers for radiation-induced injury via mass spectrometry. Health Phys. 2014;106:106–119.
- Cortez MA, Bueso-Ramos C, Ferdin J, et al. MicroRNAs in body fluids–the mix of hormones and biomarkers. Nat Rev Clin Oncol. 2011;8:467–477.
- Chim SS, Shing TK, Hung EC, et al. Detection and characterization of placental microRNAs in maternal plasma. Clin Chem. 2008;54:482–490.
- Menon N, Rogers CJ, Lukaszewicz AI, et al. Detection of acute radiation sickness: a feasibility study in non-human primates circulating miRNAs for triage in radiological events. PLoS One. 2016;11:e0167333.
- Fendler W, Malachowska B, Meghani K, et al. Evolutionarily conserved serum microRNAs predict radiation-induced fatality in nonhuman primates. Sci Transl Med. 2017;9:eaal2408.
- Zhang C, Peng G. Non-coding RNAs: an emerging player in DNA damage response. Mutation Res Rev Mutat Res. 2015;763:202–211.
- Guo L, Zhao Y, Yang S, et al. An integrated evolutionary analysis of miRNA-lncRNA in mammals. Mol Biol Rep. 2014;41:201–207.
- Rana S, Kumar R, Sultana S, et al. Radiation-induced biomarkers for the detection and assessment of absorbed radiation doses. J Pharm Bioallied Sci. 2010;2:189–196.
- Swartz HM, Williams BB, Zaki BI, et al. Clinical EPR: unique opportunities and some challenges. Acad Radiol. 2014;21:197–206.
- Swartz HM, Williams BB, Flood AB. Overview of the principles and practice of biodosimetry. Radiat Environ Biophys. 2014;53:221–232.
- Byrum SD, Burdine MS, Orr L, et al. Time- and radiation-dose dependent changes in the plasma proteome after total body irradiation of non-human primates: implications for biomarker selection. PLoS One. 2017;12:e0174771.
- Byrum SD, Burdine MS, Orr L, et al. A quantitative proteomic analysis of urine from gamma-irradiated nonhuman primates. J Proteomics Bioinform. 2016;9.
- Pannkuk EL, Laiakis EC, Authier S, et al. Gas chromatography/mass spectrometry metabolomics of urine and serum from nonhuman primates exposed to ionizing radiation: impacts on the tricarboxylic acid cycle and protein metabolism. J Proteome Res. Forthcoming 2017.
- Pannkuk EL, Laiakis EC, Mak TD, et al. A lipidomic and metabolomic serum signature from nonhuman primates exposed to ionizing radiation. Metabolomics. 2016;12:pii: 80.
- Pannkuk EL, Laiakis EC, Authier S, et al. Targeted metabolomics of nonhuman primate serum after exposure to ionizing radiation: potential tools for high-throughput biodosimetry. RSC Advances. 2016;6:51192–51202.
- Laiakis EC, Pannkuk EL, Diaz-Rubio ME, et al. Implications of genotypic differences in the generation of a urinary metabolomics radiation signature. Mutat Res. 2016;788:41–49.
- Pannkuk EL, Laiakis EC, Authier S, et al. Global metabolomic identification of long-term dose-dependent urinary biomarkers in nonhuman primates exposed to ionizing radiation. Radiat Res. 2015;184:121–133.
- Chen Z, Coy SL, Pannkuk EL, et al. Rapid and high-throughput detection and quantitation of radiation biomarkers in human and nonhuman primates by differential mobility spectrometry-mass spectrometry. J Am Soc Mass Spectrom. 2016;27:1626–1636.
- Pannkuk EL, Fornace AJ Jr., Laiakis EC. Metabolomic applications in radiation biodosimetry: exploring radiation effects through small molecules. Int J Radiat Biol. 2017;1–26.
- Johnson CH, Gonzalez FJ. Challenges and opportunities of metabolomics. J Cell Physiol. 2012;227:2975–2981.
- Johnson CH, Patterson AD, Krausz KW, et al. Radiation metabolomics. 5. Identification of urinary biomarkers of ionizing radiation exposure in nonhuman primates by mass spectrometry-based metabolomics. Radiat Res. 2012;178:328–340.
- Goudarzi M, Mak TD, Jacobs JP, et al. An Integrated multi-omic approach to assess radiation injury on the host-microbiome axis. Radiat Res. 2016;186:219–234.
- Amundson SA, Do KT, Shahab S, et al. Identification of potential mRNA biomarkers in peripheral blood lymphocytes for human exposure to ionizing radiation. Radiat Res. 2000;154:342–346.
- Ahlem CN, White SK, Page TM, et al. Differential metabolism of androst-5-ene-3beta,17beta-diol between rats, canines, monkeys and humans. Steroids. 2011;76:669–674.
- Amgen Inc. Neupogen (filgrastim) injection for subcutaneous or intravenous use. 2015. [cited 2015 Apr 2]. Available from: http://pi.amgen.com/united_states/neupogen/neupogen_pi_hcp_english.pdf
- Amgen Inc. Neulasta (pegfilgrastim) injection for subcutaneous use. 2015. [cited 2015 Nov 19]. Available from: http://pi.amgen.com/united_states/neulasta/neulasta_pi_hcp_english.pdf
- Johnke RM, Sattler JA, Allison RR. Radioprotective agents for radiation therapy: future trends. Future Oncol. 2014;10:2345–2357.