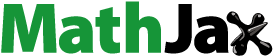
ABSTRACT
Introduction: Both ischaemic preconditioning (IPC) and muscle heat maintenance can be effective in enhancing repeated-sprint performance (RSA) when applied individually, acting mechanisms of these interventions, however, likely differ. It is unclear if, when combined, these interventions could further improve RSA. Methods: Eleven trained cyclists undertook experimental test sessions, whereby IPC (4 × 5-min at 220 mmHg) and SHAM (4 × 5-min at 20 mmHg) were each performed on two separate visits, each combined with either passive muscle heating or thermoneutral insulation prior to an “all-out” repeated-sprint task (10 × 6-s sprints with 24-s recovery). Primary outcome measures were peak and average power output (W), whist secondary measures were muscular activation and muscular oxygenation, measured via Electromyography (EMG) and Near-infrared spectroscopy (NIRS), respectively. Results: IPC did not enhance peak [6 (−14–26)W; P = 0.62] or average [12 (−7–31)W; P = 0.28] power output versus SHAM. Additionally, no performance benefits were observed when increasing muscle temperature in combination with IPC [5 (−14–19) watts; P = 0.67], or in isolation to IPC [9 (−9–28)W; P = 0.4] versus SHAM. No changes in EMG or microvascular changes were present (P > 0.05, respectively) between conditions. Conclusion: Overall, neither IPC, muscle heating, or a combination of both enhances RSA cycling performance in trained individuals.
Highlights
Neither IPC, passive muscle heating, nor combining both strategies, resulted in significant improvements peak or average power output during RSA performance.
No main effect of condition was observed on neuromuscular (EMG) or microvascular (NIRS) function during RSA performance.
After an optimised, intense warm-up, RSA performance is therefore unlikely further enhanced by IPC, passive heating, or a combining both strategies, versus a SHAM condition in trained cyclists.
Introduction
Actively warming-up is vital to enhance maximal speed and/or power production (Racinais, Cocking, & Périard, Citation2017), however, certain interventions exist that aim to enhance performance beyond that offered from actively warming-up. These interventions have been termed “preconditioning strategies” (Kilduff, Finn, Baker, Cook, & West, Citation2013). One exercise mode that could potentially benefit from preconditioning strategies is the repeated-sprint ability (RSA). RSA usually involves repeated, short-duration (<10 s) sprints, followed by incomplete (<60 s) recoveries (Bishop, Girard, & Mendez-Villanueva, Citation2011) and is often limited by two key factors including the ability to elicit peak power/velocity, and the onset of (peripheral and central) fatigue development during the performance (Bishop et al., Citation2011).
Commencing RSA with elevated muscle temperature (>38.5°C) may help to improve peak power production (Ferguson, Ball, & Sargeant, Citation2002; Girard, Brocherie, & Bishop, Citation2015; Racinais & Oksa, Citation2010; Ranatunga, Citation1982). Whilst a well-designed active warm-up can raise muscle temperature to >39°C, the rate of temperature loss is rapid in thermoneutral environments, with muscle losing ∼1–1.5°C within 7–15-min, respectively, post-warm-up (laboratory observations). If athletes are placed into holding areas for longer than 15-min prior to competing, the benefit of elevated muscle temperatures may be lost, therefore compromising supramaximal exercise performance. One method to combat muscle temperature loss post warm-up is to passively heat the limbs. Heating elements have been shown to maintain muscle temperatures by over 1°C post-exercise compared with natural cooling conditions (Raccuglia et al., Citation2016).
One caveat to elevating muscle temperature may be a faster onset of (peripheral and central) fatigue development during intense exercise (Beaven & Kilduff, Citation2018; Girard et al., Citation2015 Gregson, Batterham, Drust, & Cable, Citation2005). When aiming to maximise peak power production yet minimise the onset of fatigue, a previous review discussed the potential to use passive heating in combination with ischaemic preconditioning (IPC) (Kilduff, Finn, et al., Citation2013). IPC is a technique involving repetitive (3-4) intermittent cycles of brief (∼5 min) arterial limb occlusions performed before an active warm-up (Bailey et al., Citation2012; de Groot, Thijssen, Sanchez, Ellenkamp, & Hopman, Citation2010). In the past decade, research into the effect IPC has on exercise performance has increased dramatically (Salvador et al., Citation2016). Whilst observations in running tasks show no changes on RSA following IPC (Gibson, White, Neish, & Murray, Citation2013), recent research indicates IPC can augment peak power and force production in humans (Paradis-Deschênes, Joanisse, & Billaut, Citation2016; Patterson, Bezodis, Glaister, & Pattison, Citation2015), in addition to enhancing certain parameters of oxidative metabolism, including oxidative capacity, muscle oxygenation, oxygen extraction and aerobic metabolism in humans (Bailey et al., Citation2012; Cruz, de Aguiar, Turnes, Pereira, & Caputo, Citation2015;; de Groot et al., Citation2010; Kido et al., Citation2015 Paradis-Deschênes et al., Citation2016). Enhancement of oxidative metabolism may improve RSA performance, given RSA induces maximal muscular reoxygenation rates (Rodriguez, Townsend, Aughey, & Billaut, Citation2019) and oxygen availability is a limiting factor in repeated sprinting (Girard, Mendez-Villanueva, & Bishop, Citation2011) which can significantly impact RSA outcomes (Balsom, Ekblom, & Sjödin, Citation1994; Balsom, Gaitanos, Ekblom, & Sjödin, Citation1994).
To date, it appears that both IPC and local muscle heating strategies can significantly impact sprint-type exercises (Beaven & Kilduff, Citation2018; Patterson et al., Citation2015), but the mechanisms mediating these performance improvements are likely different and remain poorly understood. It is plausible, however, that combining both IPC and muscle heating into an applied preconditioning strategy could elicit ergogenic responses in RSA performance, especially as RSA tasks are highly taxing of both anaerobic and aerobic energy systems (Girard et al., Citation2011). The primary aim of this study was to therefore examine whether IPC, post warm-up local muscle temperature maintenance or a combination of both strategies, implemented around an active warm-up, improves RSA performance. The second aim of this study was to gain some mechanistic insight into how these preconditioning effects may act on performance outcomes; we therefore measured both muscle oxygenation and muscle activation responses during the RSA task.
Methods
Participants
Eleven trained males (mean ± SD: age, 33.7 ± 4.8 years; body mass, 81.1 ± 10 kg; height 181 ± 7 cm; maximal oxygen uptake , 57.4 ± 5.0 ml·kg−1·min−1) undertaking regular high-intensity interval cycling training (1–2 sessions/week) were recruited. Mean training experience was 9 ± 8 years and mean weekly cycling training volume was 7 ± 3 h. All data were collected within Aspetar Sports Medicine Hospital. All participants provided written informed consent prior to commencing experimental testing (Anti-Doping Lab Qatar: IRB approval number #F2017000217).
Experimental design and protocols
All participants completed a preliminary session involving a maximal graded cycling test to establish and maximal aerobic power (
), at least 2 repeated-sprint familiarisation sessions and four experimental sessions (). During the experimental sessions, participants were administered one of four different preconditioning strategies in a randomised counterbalanced order; (1) IPC only (IPC), (2) IPC and passive muscle heating of the lower limbs (IPC + HEAT), (3) SHAM only (SHAM) and (4) SHAM and passive muscle heating of the lower limbs (HEAT), that were applied before an RSA test.
Preliminary assessment
At least 7 days prior to the first familiarisation session, participants performed a continuous incremental step test on a cycle ergometer (SRM, Julich, Germany) to determine . The protocol consisted of 3-min stages and commenced at a power output of 95 W. An increase in 35 W per step occurred until volitional exhaustion was attained. Throughout the incremental test, breath-by-breath expired gases were monitored for oxygen consumption, ventilation and respiratory exchange ratio (Oxycon Pro™, Carefusion, Germany) and the highest 30-s average, determined from 3 consecutive 10-s bins were taken to determine
. Heart rate was also monitored continuously (Polar HI, Kempele, Finland).
was calculated as reported previously (Jeukendrup, Saris, Brouns, & Kester, Citation1996).
Familiarisation
At least 2 familiarisation trials of 10 × 6-s all-out sprints, separated by 24-s of recovery, were undertaken prior to experimental visits. This determined the natural variation in participant performance. Further familiarisation sessions were implemented if the coefficient of variation (CV) remained outside 3% for average sprint power, given the previous smallest worthwhile change calculations during repeated sprinting (Patterson et al., Citation2015). Data from familiarisation testing revealed CV of 3.1 ± 1.5 and 2.5 ± 1.7% peak and average power during 10 × 6-s repeated-sprints, respectively.
Experimental sessions
During the experimental visits, participants entered the laboratory and firstly performed either ischaemic preconditioning (IPC) or a control (SHAM). Immediately following IPC or SHAM, in non-heated conditions, insulation (elasticated tubigrips) was administered on the lower limb musculature. In heated (i.e. HEAT and IPC + HEAT) conditions, 16 heated pads (secured with elasticated tubigrips) were placed on the lower limb musculature and participants rested for 20-min. A 19-min standardised cycling warm-up was then performed whilst wearing the insulation on the lower limbs. The warm-up protocol aimed to elevate muscle temperature to above 39°C in both the thermoneutral and heated conditions (validated during pilot testing). Post-warm-up, participants rested for 12-min with the lower limb insulation still attached (either thermoneutral or heated). After 12-min of passive rest, electromyography (EMG) electrodes (×3) and near-infrared spectroscopy (×1) probes were placed on the left and right leg to assess muscle activation and muscle oxygenation, respectively. At minute-14 post-warm-up, participants remounted the cycle ergometer and began pedalling at 75W, aiming to reach a cadence of 105 revolutions per minute (RPM) within 1-min. At minute-15 post warm-up, a 10 × 6-s RSA commenced. All participants were instructed to elicit peak power for the entire duration of each 6-s effort. Sprints were repeated 10 times interspersed with 24-s of recovery (9-s stationary recovery; 15-s spinning at 75 W). The primary performance outcomes were peak and average power output. Sprint-by-sprint muscular activation and muscle oxygenation properties were secondary measures.
IPC protocols
For both the IPC and SHAM preconditioning strategies, 13.5 cm wide cuffs were placed bilaterally on the most proximal portions of the upper thighs. Participants lay in a supine position and cuff inflation pressure was standardised at 220 mmHg for the IPC condition and 20 mmHg in the SHAM condition, respectively, via the use of a rapid cuff inflator (Hokanson, Washington, USA). Occlusion occurred for a duration of 5-min, interspersed with 5-min of complete deflation (0 mmHg). This process was repeated 4 times in total.
Skin temperature
Immediately following IPC or SHAM, a skin temperature sensor/data logger (iButtonTM, Maxim Integrated Products, Sunnyvale, CA USA) was placed on the upper right thigh. Skin temperature was continuously recorded thereafter at a sampling rate of 60 (1 sample per min) and exported for analysis at seven time points (1: Start warm-up, 2: Warm-up 5-min, 3: Warm-up 10-min, 4: Warm-up 15-min, 5: Finish warm-up (Warm-up 19-min), 6: Start Sprint and 7: Finish Sprint).
Lower limb insulation
After placement of the skin temperature button, lower limb insulation was placed on the lower limbs. In heated (HEAT and IPC + HEAT) conditions, 16 (8 pads per limb) air-activated adhesive heat pads (THE HEAT COMPANY GmbH, Altenmarkt, Austria), reaching ∼41°C in temperature were placed on the lower limb musculature (VM; vastus medialis, VL; vastus lateralis, RF; rectus femoris, Gastrocnemius, Soleus) and secured with elasticated tubigrips (12 cm Tubular support bandage, Bastos Viegas, s.a. Guilhufe, Penafiel-Portugal). For thermoneutral (IPC and SHAM) conditions, no muscle heat pads were used, instead, elasticated tubigrips were placed directly on the skin. Indwelling muscle temperature measurements during pilot testing revealed that the warm-up elicited muscle temperature at 2 cm depth of 39.4 ± 0.1°C and 39.2 ± 0.3°C in heated and control legs, respectively. Following 15-min of passive rest following the warm-up protocol, muscle temperature was reduced by 1.7 ± 0.6°C in heated legs and 4.1 ± 0.6°C in control legs, confirming better maintenance of muscle temperature (2.4 ± 0°C; 59 ± 8%) in heated versus thermoneutral legs. Likewise, mean skin temperature value for heated legs was 41.2 ± 0.8°C versus 32.7 ± 1°C for thermoneutral legs.
Cycling warm-up
The warm-up was conducted on an SRM ergometer, lasting 19 min 10 s in total. The value attained from the incremental test (Jeukendrup et al., Citation1996) was used to prescribe 3 × 5-min submaximal warm-up stages at 40, 50 and 60%
, respectively. At minute-15 of the warm-up, participants increased cadence to 105 RPM, and at 15:30, began progressive sprint efforts consisting of 2 × 5-s at 50% peak power, 2 × 5-s at 75% peak power and 2 × 5-s at 100% peak power. The 50%, 75% and 100% 5-s efforts were separated by 10-, 30- and 60-s of rest, respectively. Heart rate was monitored throughout the warm-up (Polar H1, Kemple, Finland).
Electromyography
Skin sites of interest were shaved with a safety razor (Double Edge shaving Comb razor, National Medical Products Co. Ltd. KSA) and cleaned with a disposable sterile isopropyl alcohol swab (China Meheco Co, Ltd) prior to unit placement. Site positioning was standardised for each of the three muscles of interest (RF: 50% from ASIS to patella border; VL: 2/3 distance from ASIS to lateral patella; Biceps Femoris (BF): 50% from ischial tuberosity to lateral patella). Electrodes were secured using black adhesive tape (Kinesio Co., Ltd, Tokyo) and were reinforced with elasticated Tubigrip on the upper limb to prevent unit movement and signal contamination. One electrode was placed on the cycle ergometer crank and used as an accelerometer. All data were recorded at 2000Hz using a Delsys Trigno Wireless System (Boston, MA), rectified and low-pass filtered with a Butterworth third-order low-pass zero-lag filter and a cut-off frequency of 20 Hz. To assess relative muscle activation across the different sprints, the EMG signal was normalised to its respective maximal contraction value obtained during the 10-sprints for each participant and condition and the respective peak amplitude calculated. To account for a slight variation in placement between experimental visits and signal variance, a root mean squared (RMS) value, which reflects the mean power of the EMG signal, recommended for smoothing EMG data, was calculated for each sprint effort to derive muscle activation. The maximum RMS value for each muscle group was classified as 100%, all other RMS values were normalised to this value (% of maximum RMS).
Near-infrared spectroscopy
Changes in muscle oxygenation during the RSA test were determined using a wireless, continuous-wave near-infrared spectroscopy (NIRS) system (Portamon; Artinis Medical Systems, BV, Netherlands) via measurement of tissue oxygenation index [TOI (%)] (McCully & Hamaoka, Citation2000). The Portamon unit consisted of three emitter diodes positioned 30, 35, and 40 mm from the detector, and emitted infrared light at wavelengths of 760 and 850 nm. All analyses were undertaken on data gathered from the 35 mm emitter-detector distance, corresponding to a NIRS signal penetration depth of approximately 17.5 mm (Ferrari, Muthalib, & Quaresima, Citation2011 McCully & Hamaoka, Citation2000;). The Portamon unit was secured on the right limb VL muscle using black adhesive tape reinforced with elasticated Tubigrip to prevent movement and signal contamination from external light sources. The probe site was standardised on the VL for accurate re-positioning (see Electromyography). The skin area of interest was prepared as previously described. All NIRS data were collected at 10 Hz using a dedicated software (Oxysoft, Artinis Medical Systems) and down sampled to 1 Hz for further analyses. Finally, a differential pathlength factor of 4 was implemented to account for the scattering of the light signal. A description of the analysis procedure is provided in , with the sprint-by-sprint analysis described in detail in (b). Muscle deoxygenation (Deoxyslope) and reoxygenation rates (Reoxyslope) were determined using simple regression. Briefly, a linear model was fitted between TOImax and TOImin, and between recTOImax and recTOImin ((a)), where the slope of the linear model fit was retained as indices of de- and reoxygenation rates, respectively (Ihsan, Abbiss, Lipski, Buchheit, & Watson, Citation2013). Changes in amplitude ((b)) during deoxygenation (DeoxyAMP = TOImax − TOImin) and reoxygenation (ReoxyAMP = recTOImax − recTOImin) were determined according to previous methods (Ihsan et al., Citation2013). Linear modelling and analysis using minimum and maximum values have been shown to demonstrate the best reliability for muscle deoxygenation/reoxygenation rates and amplitude changes, respectively (Ihsan et al., Citation2013).
Figure 2. (a) Typical NIRS muscle oxygenation data during 10 × 6-s all-out sprints and (b), an example of a single 6-s sprint, followed by a 24-s recovery. Assessment of NIRS data during sprints was obtained from the first 7 s of effort, accounting for any additional pedal strokes. Deoxygenation amplitude (DeoxyAMP) and slope (Deoxyslope) were calculated from the maximal (TOImax) and minimal (TOImin) values (horizontal solid lines). Time Delay (TD) was defined as the time (s) from post-sprint to the beginning of muscle reoxygenation. Mean TD was 2.11 ± 0.95 s. Recovery amplitude (ReoxyAMP) and rate (Reoxyslope) were calculated from maximal (recTOImax) and minimal (recTOImin) values (horizontal dashed lines). TOI; Tissue oxygenation index, S1-10; Sprint number.
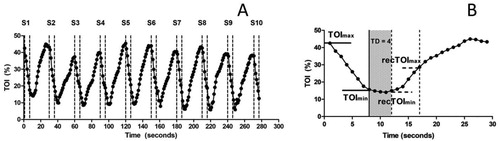
Repeated-sprint ability test
During the repeated-sprint protocol, the SRM ergometer was set to isokinetic mode, whereby cadence was clamped at 105 RPM. Participants completed 10 maximal sprint efforts in total, with each sprint lasting 6-s separated by 24-s recovery. Participants were instructed to produce ‘all-out’ effort from time point 0 until the end of the sprint. To ensure repeated-sprint efforts were not paced, we compared the average power from the first repetition of each RSA trial against the maximal 5-s sprint power attained in the warm-up protocol. Mean differences were −1.3 ± 8.7% across all conditions which were deemed acceptable. Participants began building cadence 15-s prior to each subsequent sprint to ensure cadence was at 105 RPM for the start of each repetition. Sprint power output was determined by analysing SRM files via custom training software (Golden Cheetah, version 3.3). Peak and average power output values were recorded for each sprint.
Statistical analysis
Using a magnitude-based inference framework, the mean effect of each sprint comparison was presented for the primary outcomes, with the uncertainty of the estimates presented as 90% confidence intervals (appropriate SI units for a given variable). The mean difference between each comparison were evaluated for their practical significance by pre-specifying the smallest worthwhile change (SWC) (Batterham & Hopkins, Citation2006). For session average power output, and session peak power output, the SWC was calculated using 0.3× CV from familiarisation data (Hopkins, Marshall, Batterham, & Hanin, Citation2009), which equated to 6W and 9W, respectively. The signal:noise ratio was determined by calculating the typical error (TE; the SD of between-trial differences divided by √2). The TE for peak and average power output was 16 and 20W, respectively. Between each comparison, together with its uncertainty, the probability (percent chances) that the true population effect was beneficial (>SWC), harmful (>SWC with opposite sign), or trivial (within ± SWC) was calculated. In combination with magnitude-based inference statistics, the peak and average power output for each sprint, in addition to NIRS and EMG data for each sprint were analysed using a repeated measures 2-way general linear model (Condition [4 levels: IPC vs IPC + HEAT vs SHAM vs HEAT], Time [10 levels]) with 95% confidence intervals, using statistical software (SPSS, version 22). The least significant (LSD) method was employed for pairwise comparisons. All data were checked for normal distribution prior to statistical analysis, using the Shapiro–Wilk test.
Results
Average and peak power output
IPC resulted in a 12 W increase (90% CI: −7, 31W; P = 0.3) in average power output versus SHAM, which was greater than the SWC (6W) but lower than the calculated typical error (16W) and was therefore deemed an “unclear” change (). HEAT versus SHAM [9 W (90% CI: −9, 28W); P = 0.3] and IPC versus IPC + HEAT [7W (90% CI: −11, 26W); P = 0.4] also resulted in changes greater than the calculated SWC but again were lower than the calculated typical error so were reported as “unclear” (). There was a significant decrease over time in both peak and average power output (P < 0.005, respectively). The mean reduction in peak power from sprint 1–7 was 40 ± 8 W, and mean reduction in average power from sprint 1–8 was 42 ± 23W (P < 0.006, respectively). Both peak and average power output plateaued at sprint number 8 and 9, respectively (P > 0.05, respectively). There was no statistically significant effect of condition on either peak (P = 0.98) or average power (P = 0.64) across the 10 sprints and no condition x time interaction effect was observed for either peak (P = 0.67) or average (P = 0.3) power output.
Table I. Peak and average power comparisons between conditions, accompanied with the likelihood of “beneficial”, “trivial” or “unlikely” performance outcomes.
Muscle deoxygenation
There was a significant reduction over time (P < 0.005, respectively) in DeoxyAMP (%) and Deoxyslope (%·s−1) with sprint 1 resulting in the largest DeoxyAMP and Deoxyslope when compared to all other sprints (P < 0.03 and P < 0.006, respectively). There was no statistically significant change between conditions for either DeoxyAMP (P = 0.11) or Deoxyslope (P = 0.08). Higher DeoxyAMP after both HEAT and IPC + HEAT versus SHAM [4.5 (−0.9, 9.8)% and 4.8 (−0.7, 10.3)%; P = 0.1 and P = 0.08, respectively] was also not statistically significant. SHAM likely resulted in lower mean Deoxyslope compared to IPC + HEAT [0.77 (−0.001, 1.54)%·s−1; P = 0.05] and HEAT [0.76 (−0.1, 1.63)%·s−1; P = 0.08] conditions. No condition x time interaction effect was reported for either DeoxyAMP or Deoxyslope (P > 0.05, respectively).
Muscle reoxygenation
There was no statistically significant effect of time for either ReoxyAMP (%) (P = 0.08) or Reoxyslope (%·s−1) (P = 0.47). No main effect for the condition was observed for ReoxyAMP (P = 0.18) or Reoxyslope (P = 0.19), respectively. IPC + HEAT showed a trend for greater (2.2–2.4%) increases in ReoxyAMP compared to HEAT, IPC and SHAM preconditioning strategies (P = 0.09, P = 0.19 and P = 0.07, respectively; ) but this was not statistically significant, whilst IPC + HEAT showed statistically greater Reoxyslope compared with SHAM (0.5%·s−1; P = 0.05). No condition x time interaction was observed for either ReoxyAMP or Reoxyslope (P > 0.05, respectively).
Table II. Muscle NIRS (deoxygenation [DeoxyAMP (%) and Deoxyslope (% s−1)] and reoxygenation [ReoxyAMP (%) and Reoxyslope (% s−1)] characteristics) and EMG (RMS% activity) for 10 × 6-s repeated sprints between conditions.
EMG RMS (%)
No time (P = 0.17), condition (P = 0.55), or condition x time interaction (P = 0.57) effect was observed for VL RMS. For both RF and BF, a significant reduction in RMS was observed over time (P = 0.02 and P < 0.005, respectively). There was no main effect of condition for either RF (P = 0.9) or BF (P = 0.87). Finally, no interaction effect was observed for either RF or BF (P = 0.74 and P = 0.67, respectively; ).
Skin temperature
There was a main effect of time (P = 0.01) with skin temperature at post-sprint significantly lower than all other time points (P < 0.05, respectively). Skin temperature was significantly different between heated (HEAT and IPC + HEAT) and thermoneutral preconditioning strategies, with HEAT and IPC + HEAT inducing greater skin temperature versus IPC and SHAM conditions [7.4 (6.2, 8.5)°C; P < 0.0005]. There was no difference in skin temperature between HEAT and IPC + HEAT [0.2 (−0.8, 0.3)°C; P = 0.33]. Likewise, there was no change in skin temperature between IPC and SHAM [0.1 (−0.4, 0.6)°C; P = 0.23] conditions. A significant time x condition interaction effect was present for skin temperature, with heated (HEAT and IPC + HEAT) conditions showing clear reductions once heating pads were removed at “Start sprint” and “finish sprint” time points (P < 0.05, respectively).
Discussion
The primary aim of this study was to compare IPC, passive local muscle heating or a combination of both preconditioning strategies on RSA performance. Specifically, this study explored for the first time, whether a local IPC strategy in combination with local muscle heat maintenance following a standardised cycling warm-up could enhance RSA capacity. We demonstrate that RSA performance was not enhanced by either IPC, local muscle heating, or a combination of both strategies when compared to a control (SHAM) condition.
Effects of IPC
We observed no significant effect of IPC on power output during 10 × 6-s RSA cycling. Whilst early research suggested IPC did not alter RSA (5 × 6-s sprints off 24-s recovery) performance in team-sport athletes (Gibson et al., Citation2013), more recent work reported benefits of IPC on power output during an RSA cycling task (Patterson et al., Citation2015). In that study (Patterson et al., Citation2015), peak power improvements occurred during the first 3 sprints versus SHAM, suggesting IPC had a positive impact on anaerobic energy provision (Spencer, Bishop, Dawson, & Goodman, Citation2005). During a 6-s cycling sprint effort, anaerobic glycolysis and PCr degradation account for roughly 94% of total energy contribution (Spencer et al., Citation2005). However, it is clear that aerobic contribution to each sprint increases as RSA sets progress (Dupont, McCall, Prieur, Millet, & Berthoin, Citation2010).
Previous work suggests IPC meaningfully enhances performance by approximately 1.5% in well-trained individuals (Ferreira et al., Citation2016), however, the current data show the difference in average power output between IPC and SHAM was just 1.2%, possibly supporting the “unclear” inference. It may be that IPC is less effective in highly-trained athletes (Hittinger et al., Citation2015) such as the current study cohort, compared to lesser-trained individuals (de Groot et al., Citation2010), although individual responders to IPC interventions should also be considered, regardless of training status (Tomschi, Niemann, Bloch, Predel, & Grau, Citation2018). The degree to which training status impacts the efficacy of IPC remains to be determined and should be further investigated.
Muscle heating
Higher muscle temperatures when commencing exercise did not result in enhanced power output during the RSA task. Importantly, whilst it is suggested a 1°C elevation in muscle temperature can enhance cycling peak power by almost 10% (Faulkner, Ferguson, Gerrett, Hupperets, & Hodder, Citation2013), the current study protocol employed a passive rest period of 14-min between the warm-up and RSA cycling, whereas the study of Faulkner et al. (Faulkner et al., Citation2013) employed a 30-min passive rest period. This longer rest duration could explain the variance in findings between theirs, and the present study. In addition to elevating muscle temperature, it is possible that passively maintaining core temperature with blizzard jackets in cool conditions could enhance sprint running performance in team-sport athletes (Kilduff, West, Williams, & Cook, Citation2013). Importantly, previous work reports greater sprint decrement scores (%) in cycling versus running protocols (Girard et al., Citation2011) so a comparison between modalities should be cautioned. The current findings suggest that after 14-min of passive rest from a 19-min incremental warm-up, consisting of sprint efforts, there is no need to passively maintain muscle temperature prior to performing 10 × 6-s repeated sprints on a cycle ergometer. It should be acknowledged that muscle temperature was only collected during pilot work prior to experimental trials. Future work may wish to investigate these measures in more detail.
Effects of combining interventions
No benefit was observed from combining preconditioning strategies in the current study. The warm-up protocol in the current study was designed in line with recent recommendations to optimise warm-up (Racinais et al., Citation2017) whilst aiming to emulate real-world practice. Previous laboratory studies assessing the impact of either IPC or muscle heating interventions on anaerobic performance may have designed warm-up protocols that lacked sufficient exercise intensity (Cruz, de Aguiar, Turnes, Salvador, & Caputo, Citation2016), duration (Patterson et al., Citation2015) or enforced a rest period beyond optimal (Faulkner et al., Citation2013). Previously, Faulkner et al. (Faulkner et al., Citation2013) investigated muscle temperature elevations on sprint performance and conducted a warm-up involving 5-min of steady state cycling followed by 5 × 10-s sprint efforts. The time between warm-up cessation and the sprint task was 30-min, likely negating any warm-up potentiation effect (Rassier & MacIntosh, Citation2000). Additionally, Patterson et al. (Patterson et al., Citation2015) assessed the effect of IPC on RSA cycling and employed a 3-min steady state cycling warm-up followed by 2 x maximal 6-s efforts. The rest period in this study (5-min) was much more optimal (Sargeant & Dolan, Citation1987); however, the duration of the warm-up itself was likely too-short to induce sufficient elevations in muscle temperature (Racinais et al., Citation2017). The current study warm-up, lasting for 19-min in duration, was designed to emulate how an athlete may try to optimise physiological readiness prior to competition (Bishop, Citation2003; Racinais et al., Citation2017). Therefore, whilst the current design is unable to answer this question, it may be that a sufficient warm-up stimulus negates the beneficial response of IPC during predominantly anaerobic exercise tasks. It could therefore be speculated that muscle heating may be most effective in sub-optimal preparation environments, where either an insufficient warm-up has been undertaken, or indeed if the rest duration between warm-up and competition is too long. Future research should aim to explore anaerobic tasks that are preceded by more appropriate warm-up procedures.
Muscle characteristics to RSA
Muscle oxygenation kinetics are likely important in RSA, due to the previously highlighted aerobic component to performance (Girard et al., Citation2011). Although trends for enhanced muscle oxygenation responses were evident in heated conditions, “unclear” changes in power output were present. We observed that IPC + HEAT enhanced the reoxygenation rate between sprints versus SHAM, but again, this did not translate to changes in power output. Previously, it has been postulated that beneficial responses to IPC are induced via enhanced microvascular changes in the muscle (Paradis-Deschênes et al., Citation2016), however, based on current data, those assumptions may be challenged. The current data also show no impact on EMG RMS (%) between conditions. Patterson et al. (Patterson et al., Citation2015) reported a possibly higher median frequency in EMG after IPC versus SHAM. In the current study, RMS was analysed to control for the differences in muscle temperature between preconditioning strategies. Greater muscle temperatures during exercise can alter neurophysiological excitatory during contractions (Racinais et al., Citation2017). Current data suggest muscle activation did not differ between conditions, which when combined with no clear change in power output, would indicate neither preconditioning strategy alters neurophysiological excitatory responses to RSA cycling. Our study was not designed to further validate potential placebo responses to IPC (Ferreira et al., Citation2016; Sabino-Carvalho et al., Citation2017), however this should be considered in future research designs. Finally, whilst the rigour in use of MBI analysis on performance endpoints in the current manuscript was less strict than analysis used on secondary measures, use of more stringent statistical approaches would not have altered the interpretation of the current data set.
Conclusion
Our data demonstrate that following an optimised active warm-up strategy, RSA is unlikely further enhanced by implementing IPC, passive heating, or combining both strategies, versus a SHAM condition. This observation is supported with no documented changes to either neuromuscular (EMG) or microvascular (NIRS) changes during RSA cycling. Our data provide greater clarity on the efficacy of IPC when integrated and compared with other popular preconditioning strategies.
Acknowledgements
We would like to thank all participants for attending all required familiarisation sessions and performing maximally exerting experimental sessions. The publication of this article was funded by the Qatar National Library.
Disclosure statement
No potential conflict of interest was reported by the author(s).
References
- Bailey, T. G., Jones, H., Gregson, W., Atkinson, G., Cable, N. T., & Thijssen, D. H. J. (2012 November). Effect of Ischemic preconditioning on lactate accumulation and running performance. Medicine & Science in Sports & Exercise, 44(11), 2084–2089. doi: 10.1249/MSS.0b013e318262cb17
- Balsom, P. D., Ekblom, B., & Sjödin, B. (1994 April). Enhanced oxygen availability during high intensity intermittent exercise decreases anaerobic metabolite concentrations in blood. Acta Physiologica Scandinavica, 150(4), 455–456. doi: 10.1111/j.1748-1716.1994.tb09711.x
- Balsom, P. D., Gaitanos, G. C., Ekblom, B., & Sjödin, B. (1994 November). Reduced oxygen availability during high intensity intermittent exercise impairs performance. Acta Physiologica Scandinavica, 152(3), 279–285. doi: 10.1111/j.1748-1716.1994.tb09807.x
- Batterham, A. M., & Hopkins, W. G. (2006 March). Making meaningful inferences about magnitudes. International Journal of Sports Physiology and Performance, 1(1), 50–57. doi: 10.1123/ijspp.1.1.50
- Beaven, C. M., & Kilduff, L. P. (2018 August 3). Cook CJ. Lower-limb passive heat maintenance combined With Pre-cooling improves repeated sprint ability. Front Physiology [Internet], 9. [cited 2018 August 19]. Retrieved from https://www.frontiersin.org/article/10.3389/fphys.2018.01064/full
- Bishop, D. (2003). Warm up I. Sports Medicine, 33, 439–454. doi: 10.2165/00007256-200333060-00005
- Bishop, D., Girard, O., & Mendez-Villanueva, A. (2011 September 1). Repeated-sprint ability – part II: Recommendations for training. Sports Medicine, 41(9), 741–756. doi: 10.2165/11590560-000000000-00000
- Cruz, R. S., de Aguiar, R. A., Turnes, T., Pereira, K. L., & Caputo, F. (2015 November 1). Effects of ischemic preconditioning on maximal constant-load cycling performance. Journal of Applied Physiology, 119(9), 961–967. doi: 10.1152/japplphysiol.00498.2015
- Cruz, R. S., de Aguiar, R. A., Turnes, T., Salvador, A. F., & Caputo, F. (2016 August). Effects of ischemic preconditioning on short-duration cycling performance. Appl Physiol Nutr Metab Physiol Appl Nutr Metab, 41(8), 825–831. doi: 10.1139/apnm-2015-0646
- de Groot, P. C. E., Thijssen, D. H. J., Sanchez, M., Ellenkamp, R., & Hopman, M. T. E. (2010 January). Ischemic preconditioning improves maximal performance in humans. European Journal of Applied Physiology, 108(1), 141–146. doi: 10.1007/s00421-009-1195-2
- Dupont, G., McCall, A., Prieur, F., Millet, G. P., & Berthoin, S. (2010 October). Faster oxygen uptake kinetics during recovery is related to better repeated sprinting ability. European Journal of Applied Physiology, 110(3), 627–634. doi: 10.1007/s00421-010-1494-7
- Faulkner, S. H., Ferguson, R. A., Gerrett, N., Hupperets, M., Hodder, S. G., & Havenith, G. (2013 February). Reducing muscle temperature drop after warm-up improves sprint cycling performance. Medicine & Science in Sports & Exercise, 45(2), 359–365. doi: 10.1249/MSS.0b013e31826fba7f
- Ferguson, R. A., Ball, D., & Sargeant, A. J. (2002). Effect of muscle temperature on rate of oxygen uptake during exercise in humans at different contraction frequencies. Journal of Experimental Biology, 205(7), 981–987.
- Ferrari, M., Muthalib, M., & Quaresima, V. (2011 November 28). The use of near-infrared spectroscopy in understanding skeletal muscle physiology: Recent developments. Philos Trans R Soc Math Phys Eng Sci, 369(1955), 4577–4590.
- Ferreira, T. N., Sabino-Carvalho, J. L. C., Lopes, T. R., Ribeiro, I. C., Succi, J. E., Da Silva, A. C., et al. (2016 October). Ischemic preconditioning and repeated sprint Swimming: A placebo and Nocebo study. Medicine & Science in Sports & Exercise, 48(10), 1967–1975. doi: 10.1249/MSS.0000000000000977
- Gibson, N., White, J., Neish, M., & Murray, A. (2013). Effect of ischemic preconditioning on land-based sprinting in team-sport athletes. International Journal of Sports Physiology and Performance, 8(6), 671–676. doi: 10.1123/ijspp.8.6.671
- Girard, O., Brocherie, F., & Bishop, D. J. (2015 June). Sprint performance under heat stress: A review: Sprinting in hot ambient temperature. Scandinavian Journal of Medicine & Science in Sports, 25, 79–89. doi: 10.1111/sms.12437
- Girard, O., Mendez-Villanueva, A., & Bishop, D. (2011 August). Repeated-Sprint ability – Part I: Factors contributing to fatigue. Sports Medicine, 41(8), 673–694. doi: 10.2165/11590550-000000000-00000
- Gregson, W., Batterham, A., Drust, B., & Cable, N. (2005 May). The influence of pre-warming on the physiological responses to prolonged intermittent exercise. Journal of Sports Sciences, 23(5), 455–464. doi: 10.1080/02640410410001730214
- Hittinger, E. A., Maher, J. L., Nash, M. S., Perry, A. C., Signorile, J. F., Kressler, J., et al. (2015 January). Ischemic preconditioning does not improve peak exercise capacity at sea level or simulated high altitude in trained male cyclists. Applied Physiology, Nutrition and Metabolism, 40(1), 65–71. doi: 10.1139/apnm-2014-0080
- Hopkins, W. G., Marshall, S. W., Batterham, A. M., & Hanin, J. (2009 January). Progressive statistics for studies in sports medicine and exercise science. Medicine & Science in Sports & Exercise, 41(1), 3–13. doi: 10.1249/MSS.0b013e31818cb278
- Ihsan, M., Abbiss, C., Lipski, M., Buchheit, M., & Watson, G. (2013 March 22). Muscle oxygenation and blood volume reliability during continuous and intermittent running. International Journal of Sports Medicine, 34(07), 637–645. doi: 10.1055/s-0032-1331771
- Jeukendrup, A., Saris, W. H., Brouns, F., & Kester, A. D. (1996 February). A new validated endurance performance test. Medicine & Science in Sports & Exercise, 28(2), 266–270. doi: 10.1097/00005768-199602000-00017
- Kido, K., Suga, T., Tanaka, D., Honjo, T., Homma, T., Fujita, S., et al. (2015 May 6). Ischemic preconditioning accelerates muscle deoxygenation dynamics and enhances exercise endurance during the work-to-work test. Physiological Reports, 3(5), e12395–e12395. doi: 10.14814/phy2.12395
- Kilduff, L. P., Finn, C. V., Baker, J. S., Cook, C. J., & West, D. J. (2013). Preconditioning strategies to enhance physical performance on the day of competition. International Journal of Sports Physiology and Performance, 8(6), 677–681. doi: 10.1123/ijspp.8.6.677
- Kilduff, L. P., West, D. J., Williams, N., & Cook, C. J. (2013 September). The influence of passive heat maintenance on lower body power output and repeated sprint performance in professional rugby league players. Journal of Science and Medicine in Sport, 16(5), 482–486. doi: 10.1016/j.jsams.2012.11.889
- McCully, K. K., & Hamaoka, T. (2000 July). Near-infrared spectroscopy: What can it tell us about oxygen saturation in skeletal muscle? Exercise and Sport Sciences Reviews, 28(3), 123–127.
- Paradis-Deschênes, P., Joanisse, D. R., & Billaut, F. (2016 September). Ischemic preconditioning increases muscle perfusion, oxygen uptake, and force in strength-trained athletes. Applied Physiology, Nutrition, and Metabolism, 41(9), 938–944. doi: 10.1139/apnm-2015-0561
- Patterson, S. D., Bezodis, N. E., Glaister, M., & Pattison, J. R. (2015 August). The effect of ischemic preconditioning on repeated sprint cycling performance. Medicine & Science in Sports & Exercise, 47(8), 1652–1658. doi: 10.1249/MSS.0000000000000576
- Raccuglia, M., Lloyd, A., Filingeri, D., Faulkner, S. H., Hodder, S., & Havenith, G. (2016 February). Post-warm-up muscle temperature maintenance: Blood flow contribution and external heating optimisation. European Journal of Applied Physiology, 116(2), 395–404. doi: 10.1007/s00421-015-3294-6
- Racinais, S., Cocking, S., & Périard, J. D. (2017 July 3). Sports and environmental temperature: From warming-up to heating-up. Temperature, 4(3), 227–257. doi: 10.1080/23328940.2017.1356427
- Racinais, S., & Oksa, J. (2010 October 4). Temperature and neuromuscular function: Temperature and neuromuscular function. Scandinavian Journal of Medicine & Science in Sports, 20, 1–18. doi: 10.1111/j.1600-0838.2010.01204.x
- Ranatunga, K. W. (1982). Temperature-dependence of shortening velocity and rate of isometric tension development in rat skeletal muscle. Journal of Physiology, 329(1), 465–483. doi: 10.1113/jphysiol.1982.sp014314
- Rassier, D. E., & MacIntosh, B. R. (2000 May). Coexistence of potentiation and fatigue in skeletal muscle. Brazilian Journal of Medical and Biological Research, 33(5), 499–508. doi: 10.1590/S0100-879X2000000500003
- Rodriguez, R. F., Townsend, N. E., Aughey, R. J., & Billaut, F. (2019 September 19). Muscle oxygenation maintained during repeated-sprints despite inspiratory muscle loading. PLOS ONE, 14(9), e0222487. doi: 10.1371/journal.pone.0222487
- Sabino-Carvalho, J. L., Lopes, T. R., Obeid-Freitas, T., Ferreira, T. N., Succi, J. E., Silva, A. C., et al. (2017 January). Effect of Ischemic preconditioning on Endurance performance does Not Surpass placebo. Medicine & Science in Sports & Exercise, 49(1), 124–132. doi: 10.1249/MSS.0000000000001088
- Salvador, A. F., De Aguiar, R. A., Lisba, F. D., Pereira, K. L., Cruz RS, O., & Caputo, F. (2016 January). Ischemic preconditioning and exercise performance: A systematic review and meta-analysis. International Journal of Sports Physiology and Performance, 11(1), 4–14. doi: 10.1123/ijspp.2015-0204
- Sargeant, A. J., & Dolan, P. (1987). Effect of prior exercise on maximal short-term power output in humans. Journal of Applied Physiology, 63(4), 1475–1480. doi: 10.1152/jappl.1987.63.4.1475
- Spencer, M., Bishop, D., Dawson, B., & Goodman, C. (2005). Physiological and metabolic responses of repeated-sprint activities: Specific to field-based team sports. Sports Medicine, 35(12), 1025–1044. doi: 10.2165/00007256-200535120-00003
- Tomschi, F., Niemann, D., Bloch, W., Predel, H.-G., & Grau, M. (2018 July). Ischemic preconditioning enhances performance and erythrocyte deformability of responders. International Journal of Sports Medicine, 39(08), 596–603. doi: 10.1055/a-0631-2887