ABSTRACT
The main aim of this study was to examine the influence of ischemic preconditioning (IPC) on maximal accumulated oxygen deficit (MAOD). We conducted a three-arm and assessor-blinded randomized, controlled crossover study. Sixteen 400-meter running male athletes (19.9 ± 1.3 years; 1.78 ± 0.05 m; 67.9 ± 5.5 kg) completed three supramaximal intensity tests separated with Control, Local (legs), and Remote (arms) IPC interventions. IPC was induced on the limbs on both sides (4×5 min alternating unilateral occlusion 220 mmHg and reperfusion; arms or thighs; right side first) before participants performed the supramaximal intensity test on a treadmill at 110% VO2max intensity to exhaustion. During each test, indices of respiratory gas exchange, blood lactate, and heart rate were determined. The MAOD was calculated as the difference between the theoretical VO2 demand and the actual VO2 during the supramaximal intensity test. Differences from three trials were analyzed using ANOVA with repeated measures. IPC increased MAOD (RIPC, 59 ± 17 ml/kg/min, p = 0.018; LIPC, 57 ± 15 ml/kg/min, p = 0.037; p < 0.05) compared with Control (49 ± 9 ml/kg/min). Time to exhaustion was enhanced after IPC (Control: 257.2 ± 69.5 s, RIPC, 292.3 ± 66.6 s, p = 0.048; LIPC, 291.6 ± 79.2 s, p = 0.042; p < 0.05). In contrast, the enhancements of RIPC and LIPC trials were similar (p = 1.000). Blood lactate concentrations were similar across the three intervention conditions (p > 0.05). Acute IPC improved MAOD and supramaximal intensity exercise capacity in 400-meter running athletes. The increased MAOD indicated greater anaerobic capacity, which can be the potential mediator for improvement in exhaustion time.
Highlights
Ischemic preconditioning may improve the exhaustion time of supramaximal intensity running in well-trained 400-meter middle distance athletes.
Acute IPC may be beneficial to anaerobic exercise capacity as the maximal accumulated oxygen deficit increases after IPC.
Acute IPC occlusion on the upper arms or thighs may improve anaerobic capacity.
KEYWORDS:
Introduction
Brief, intermittent occlusion of blood-flow restriction and reperfusion exposure, with repeated cycles, is commonly termed as Ischemic preconditioning (IPC) (Loukogeorgakis et al., Citation2005). IPC was first mentioned by Murry, Jennings, and Reimer (Citation1986). In a competitive scenario, small improvements are crucial for elite athletes. IPC has been shown to have ergogenic effects in aerobic and anaerobic exercises (Salvador et al., Citation2016). The IPC protocols are however diverse and the responses are inconsistent (O'Brien & Jacobs, Citation2021).
High-intensity track performance is strongly related to anaerobic capacity and energy supply depends on glycolysis to produce energy (Medbo et al., Citation1988). The rate of ATP production through anaerobic metabolism will determine power and exercise tolerance time (Lacour, Bouvat, & Barthelemy, Citation1990). It is known that ATP is formed through anaerobic pathways (the breakdown of phosphocreatine and glycolysis) when an oxygen deficit occurs (LI Yong-Ming, Citation2014). The maximal accumulated oxygen deficit (MAOD) is used as an effective method to quantify anaerobic capacity and evaluate anaerobic metabolism (Medbo et al., Citation1988). The MAOD method is considered a “gold standard” for anaerobic capacity measurement (Medbo et al., Citation1988; Noordhof, de Koning, & Foster, Citation2010), although its validity has been questioned (Noordhof et al., Citation2010). IPC was speculated to influence MAOD since research showed that IPC can enhance supramaximal intensity exercise performance and improved accumulated oxygen deficit (Cruz, de Aguiar, Turnes, Salvador, & Caputo, Citation2016; Paull & Van Guilder, Citation2019). However, the relationship between MAOD and IPC has rarely been tested.
Differences in dose, timing, location of the cuff across several IPC protocols have led to varying outcomes (Cocking, Jones, Cable, & Thijssen, Citation2019). Two IPC protocols: remote and local IPC, may lead to different effects (Barbosa et al., Citation2015; Cocking et al., Citation2018). Few studies have directly compared these two protocols. It therefore remained unclear whether the remote IPC was better than the local protocol in terms of performance improvement. We hypothesized that IPC could improve MAOD and prolong the exhaustion time during supramaximal intensity running. As such, we aimed to investigate the effect of IPC on the MAOD of 400 m athletes. Secondly, we also examined whether remote and local IPC could both improve anaerobic exercise performance and compared the effects of the two IPC interventions.
Materials and methods
Participants
A total of sixteen healthy 400-meter male runners volunteered to participate in this study. Four athletes had personal timing from the record within 49.6 s, and 12 athletes ran within 53.1 s. Their baseline characteristics are shown in . All athletes trained 3 times a week during this study and were familiar with wearing a portable gas metabolism analyzer for running tests. They had no history of disease and were not on medication. They were asked to refrain from alcohol, caffeine, and intensive training for at least 24 h before all testing (Tanner & Gore, Citation2013). Before signing the informed consent, the possible risks and benefits of the present study were informed. To avoid the placebo effect, participants were informed in advance that the IPC intervention was just an experimental procedure and would not produce any additional effects. In addition, athletes were blinded for their speed, exercise time, and other physiological data during the experiments. The study was approved by the Beijing Sport University Institutional Review Board.
Table 1. Baseline participant characteristics (n = 16).
Experimental design
We designed a three-arm and assessor-blinded (assessors blinded during the statistical analysis) randomized, controlled cross-over study to compare the effects of RIPC and LIPC on MAOD . There was no intention to set up a sham trial, as participants were not blinded for the pressure and/or the cuff position in the LIPC or RIPC trials. Each athlete visited the laboratory for five different experimental exercise tests (including three experimental trials). All tests were performed on a laboratory treadmill (Stratos med, H/p/cosmos, Traunstein, Germany) with an inclination of 1% (Spencer & Gastin, Citation2001). First, athletes needed to complete an incremental maximal running test to determine the VO2max. A constant load test was used to determine the linear relationship between VO2 and exercise intensity (running speed). One week later, the participants performed the first supramaximal test to obtain the MAOD baseline values, which was considered the control trial without the IPC intervention. Seven days after the control trial, participants were randomized to complete the RIPC or LIPC trial (LIPC or RIPC intervention and additional supramaximal intensity test). After a 7-day washout to eliminate the delayed effect of IPC (Loukogeorgakis et al., Citation2005), participants were crossed to the other IPC intervention and performed the same procedure at the same time of the day. To minimize the sequence effect, randomization sequences were generated using a computer. Based on the sequence, participants were randomly assigned to a test order (RIPC-LIPC or LIPC-RIPC) to achieve counterbalance between groups. In addition, the allocation results were blinded to all athletes. The laboratory conditions were controlled at a temperature of 21.9 ± 2.4°C and relative humidity of 43 ± 8%. Body composition was measured using a digital dual-energy X-ray detector (iDxa, General Electric Company, Fairfield, United States). Respiratory variables were collected using a portable metabolic system (MetaMax 3B, Cortex Biophysic, Leipzig, Germany) during the incremental maximal running test, constant load test, and supramaximal test. Before testing, the portable system was calibrated in accordance with the manufacturer's instructions. Capillary blood samples were drawn from the fingertip before the test and at 1, 4, 7, and 10 min after the incremental maximal running test and the supramaximal test (Hanon, Lepretre, Bishop, & Thomas, Citation2010). Blood lactate concentration was assessed in whole blood using a portable lactate analyzer (Biosen S-line lab, EKF Diagnostic, Barleben, Germany).
Ischemic preconditioning
IPC was performed 30 min before the supramaximal tests, and the protocol involved alternating unilateral limb occlusion. Athletes were asked to lay supine to undergo blood flow restriction, using automated occlusion cuffs (arms: 41 cm×52 mm, legs: 72 cm×52 mm) (KAATSU Master, Sato Sports Plaza, Tokyo, Japan). IPC procedure consisted of 4 cycles of 5 min-occlusion induced by cuff inflation to 220 mmHg, followed by deflation and 5 min of reperfusion of blood flow. For the LIPC protocol, we placed the cuffs on the upper thighs (near the inguinal ligament). For RIPC on the arms, cuffs were placed on the proximal part of the upper arm to limit radial artery blood flow. To confirm the occlusion of blood flow, the near-infrared spectroscopy (PortaLite-Tragbares 3-Kanal NIRS-System, Neurolite AG, Belp, Switzerland) was used to observe whether there was a plateau in the NIRS-derived deoxy-hemoglobin signal.
Maximal incremental running test
The maximal incremental running test was performed on the laboratory treadmill (Stratos med, H/p/cosmos, Traunstein, Germany), with a warm-up period of 5 min at a speed of 8 km/h followed by stretching. The protocol began at 10 km/h for 2 min with an incline of 1% (Spencer & Gastin, Citation2001), and the speed was increased by 1 km/h every 2 min until exhaustion (Bosquet, Duchene, Delhors, Dupont, & Carter, Citation2008). The participant wore a portable gas metabolism analyzer during the running exercise. The following three criteria were used to determine whether the participant was exhausted (Peng, Citation2011): I. VO2 increase≤2.1 ml/kg/min in two or more consecutive 1-min; II. Blood lactate concentration≥8.0 mmol/L 1 min after the test; III. Respiratory exchange ratio≥1.10; IV. Heart rate≥110% HRmax(220-age).
Constant load test
Seven days after the maximal incremental running test, participants were allowed to rest for 5 min after arriving at the laboratory. Then, they were asked to wear a portable gas metabolism analyzer to measure resting VO2 in a sitting position. According to the VO2max data, athletes completed the 5-stage constant-load treadmill test (50%, 60%, 70%, 80%, and 90% VO2max), each stage lasted 10 min, and the treadmill inclination was set to 1%. Every stage followed a 4 min rest, the participant kept wearing a mask until the VO2 returned to the difference within 3 ml/min/kg of the VO2 of pre-exercise from the last stage, and then the participant was allowed to perform the next stage of constant load test (Gao et al., Citation2013). We took the last 2 min (8-10 min) VO2 average value of each load level and its corresponding load intensity (running speed) for linear mixing.
Supramaximal intensity test
Participants completed a specific warm-up at 10 km/h lasting for 10 min; the formal test then started after donning the test equipment. The participants were required to run to exhaustion (2-3 min) (Medbo et al., Citation1988) at 110% VO2max intensity for each of the Control, RIPC, and LIPC trials. The 110% VO2max intensity was calculated based on the intensity (running speed) when athletes reached their VO2max in the incremental maximal running test. The load intensity for each participant was the same in all three trials. Participants wore masks during the whole running test to collect respiratory gas exchange data and actual VO2 data.
Figure 1. Schematic representation of the experimental protocol of the present study. VO2max: incremental maximal running test to access the VO2max of participants. MAOD: maximal accumulated oxygen deficit. Con: Control trial. LIPC: local ischemic preconditioning. RIPC: remote ischemic preconditioning. RL: right leg. LL: left leg. RA: right arm. LA: left arm. The black boxes represent the unilateral IPC intervention, and the white boxes represent the reperfusion. All IPC interventions are first on right side and second on the left side.
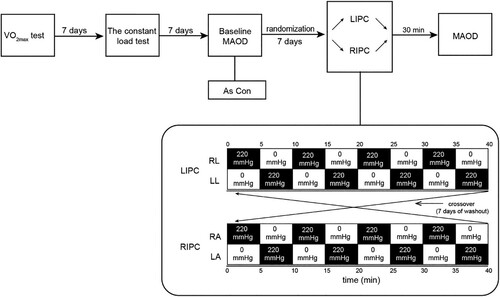
MAOD calculation
MAOD was determined by extrapolating the linear relationship between running speed and O2 uptake. The theoretical oxygen demand was extrapolated according to the relationship between linear running speed and oxygen uptake that was established using the 5-stage constant load test data. The accumulated demand VO2 was determined from the theoretical O2 uptake demand (constant during the whole exercise test) and the running time. The accumulated actual VO2 was determined in the same way. Accordingly, the MAOD of the supramaximal intensity test was obtained by calculating the difference between the estimated accumulated VO2 demand and the accumulated actual VO2. -B shows the kinetic fitting curve of oxygen uptake of participant A during the supramaximal intensity test and provides a schematic diagram of the assessment of MAOD in this study.
Figure 2. The linear relationship between oxygen uptake and velocity for participant A during the five-stage submaximal constant intensity test is presented in panel A. The kinetic fitting curve of oxygen uptake during the supramaximal intensity test for participant A is presented in panel B. The grey area represents the MAOD.
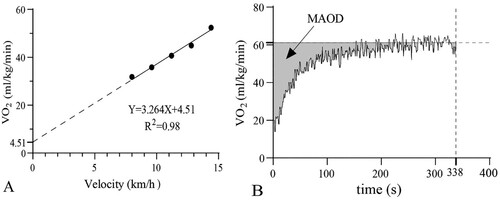
Statistical analysis
Data of the present study were statistically analyzed using SPSS statistical analysis software (version 21.0). GraphPad Prism 8 was used to fit the oxygen uptake-output power equation, and the results were presented as mean ± SD. Pearson correlation analysis was conducted to determine the relationship between the changes in MAOD, exhaustion time, and blood lactate concentration compared with Control. The Shapiro–Wilk test was used to check the normal distribution of data. Absolutes among the three trials were analyzed using ANOVA with repeated measures and the Bonferroni post hoc test method for pairwise comparison. The significance level was set at a p-value of <0.05.
Results
Time to exhaustion
An improvement in running time was found after IPC (p < 0.05). Bonferroni’s posthoc comparison showed that the running time was 34.4 s (95CI%: 7.97–60.78) for LIPC and 35.1 s (95CI%: 7.54–62.58) for RIPC, longer than the baseline (p = 0.042 and p = 0.048, respectively). The exhaustion time was increased by 13.4% and 13.6% in LIPC and RIPC trial respectively. There was no difference in running time between LIPC and RIPC modes (p > 0.05).
MAOD
The linear relationship between oxygen uptake and velocity for participant A is shown in -A. The mean slope of the mixed regression curves for all participants was 3.3 ± 0.2, and R2 was 0.96 ± 0.26. The MAOD values were calculated using the linear equation and the measured variables. There was an enhancement in MAOD in RIPC and LIPC compared with the control (p < 0.05). After conducting the Bonferroni post hoc test, the MAOD for LIPC (57 ± 14 ml/kg/min) and RIPC (59 ± 16 ml/kg/min) were higher than the control (49 ± 9 ml/kg/min, p = 0.018 and p = 0.037, respectively). The LIPC and RIPC improved MAOD by 17.7% and 21.2% compared to control. No difference in MAOD was found between the LIPC and RIPC modes (p > 0.05).
Blood lactate
We collected the blood lactate at the 1st, 4th, 7th and 10th minutes respectively, and selected the highest value among them as the accumulated blood lactate concentrations. The concentration of control and ischemic preconditioning trials were similar in all tests (RIPC: 10.7 ± 3.9 mmol/L, LIPC: 12.9 ± 2.4 mmol/L, Control: 12.7 ± 3.7 mmol/L, p > 0.05).
Correlation of the change values of IPC compared with control
Pearson correlation analysis showed a significant relationship between the improvement of MAOD and an increase in exhaustion time after RIPC and LIPC intervention (TIMERIPC-Control and MAODRIPC-Control, r = 0.660, p = 0.005; TIMELIPC-Control and MAODLIPC-Control, r = 0.597, p = 0.015) (). In contrast, no correlations were observed between the changes in blood lactate and exhaustion times (TIMERIPC-Control and BLARIPC-Control, r = −0.101, p = 0.714, TIMELIPC-Control and BLALIPC-Control, r = −0.338, p = 0.201), and between the changes of blood lactate and MAOD (MAODRIPC-Control and BLARIPC-Control, r = 0.068, p = 0.802; MAODLIPC-Control and BLALIPC-Control, r = −0.050, p = 0.082) after IPC interventions.
Figure 3. The relationships between all participants’ changes of MAOD and exhaustion time after IPC trials. LIPC-Control: the change between LIPC and Control; RIPC-Control: the change between LIPC and Control; the fitting trend lines between MAOD and time from LIPC-Control and RIPC-Control are presented.
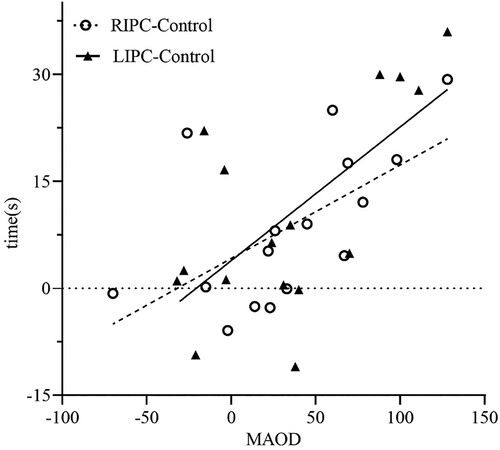
Discussion
It has been speculated that IPC is more likely to influence populations with excellent physical fitness level (Paull & Van Guilder, Citation2019). In the present study, the IPC intervention improved time-to-exhaustion and increased the MAOD in an athletic population. IPC to the limbs improved the anaerobic metabolic capacity during supramaximal intensity exercises.
The application of IPC on anaerobic metabolism is a source of debate (Salvador et al., Citation2016). The novel findings of our study are MAOD and the running time to exhaustion of athletes increased with IPC compared with the control trial. The increasing magnitude in accumulated oxygen deficit was similar with Cruz et al. (de Oliveira Cruz, de Aguiar, Turnes, Salvador, & Caputo, Citation2016).
A strong correlation between MAODIPC-Control and TIMEIPC-Control in our study was also found. From the regression point of view, there is a trend of linear relationship between the two from . It was speculated that the larger MAOD mediated improved anaerobic endurance capacity. Our data demonstrated that the application of IPC was ergogenic to anaerobic capacity in supramaximal intensity running, and the improved MAOD may be the mediator of prolonged exhaustion time.
The Wingate Anaerobic Test (WAnT) is a popular method to assess anaerobic exercise performance. Prior studies have adopted WAnT test methods for measurement, and the average and peak powers were enhanced after IPC intervention (Kraus et al., Citation2015; Lindsay et al., Citation2017). However, the peak and mean powers measured from WAnT are not reliable indicators to assess anaerobic capacity but are good predictors compared with MAOD (Minahan, Chia, & Inbar, Citation2007). Moreover, studies have shown that power does not translate to capacity (Minahan et al., Citation2007). Therefore, we applied the MAOD test to assess anaerobic capacity.
Alternatively, our research shows that IPC does not affect blood lactate accumulation during exercise. This result was in agreement with Kraus et al. (Citation2015) and Clevidence, Mowery, and Kushnick (Citation2012) Moreover, the changes in blood lactate are not always consistent with performance enhancement. Conclusions from a classic IPC study reported a decrease in blood lactate concentrations after IPC but the running performance was improved (Bailey et al., Citation2012). An explanation is an indirect decline in lactate production due to reduced ATP depletion caused by IPC (Murry et al., Citation1986), which was termed an “energy sparing effect” by Pang et al. (Citation1995).
No correlation was found between the changes of MAOD and blood lactate after IPC, which might account for the limitation of the traditional MAOD method that cannot estimate the energy supply ratio from ATP-CP and glycolysis system (Bertuzzi, Kiss, Damasceno, Oliveira, & Lima-Silva, Citation2015). Furthermore, blood lactate can fluctuate in concentration, as it can act as the product or the substrate from different pathways. Thus it should not be used as the sole indicator to quantify anaerobic capacity (Gastin, Citation1994).
LIPC was mostly applied in the previous studies that induced directly on the exercising limbs (Barbosa et al., Citation2015). Meanwhile, RIPC applied to the arms has also been shown to be beneficial in leg-dominant exercises (e.g. running, cycling) (Paull & Van Guilder, Citation2019). Theoretically, MAOD consists of the anaerobic alactic and lactate components. Since no increase in blood lactate was observed after acute IPC in our study, we suspected the energy supply from the alactic system may be enhanced. It has previously been reported that LIPC acting directly on local limbs can trigger relevant receptor-mediated responses: like the increase of nitric oxide (NO) and adenosine to augment vasodilatory response (Yellon & Downey, Citation2003), which may improve muscle blood flow during high-intensity exercise. In addition, RIPC is known to produce an ergogenic effect by activating both neural (Khaliulin et al., Citation2019) (moderating autonomic nervous system (ANS)) and humoral system (Heinen et al., Citation2011) (like mediating protein kinase C (PKC) and mitogen-activated protein kinases (MAPK) pathways) that spread to target exercising limbs (Griffin, Hughes, Gissane, & Patterson, Citation2019) (arms to legs). RIPC can also promote ATP-sparing (Pang et al., Citation1995) in skeletal muscles and decreases blood PH (Heinen et al., Citation2011), and may prolong the exhaustion time during supramaximal intensity tests. Although differ in mechanisms between RIPC and LIPC, our observations concur with previous research such that anaerobic exercise performance was enhanced after LIPC and RIPC (Cocking et al., Citation2019; Griffin et al., Citation2019). The cuff locations may therefore not influence the effect of IPC (O'Brien & Jacobs, Citation2021), although more mechanistic experiments are warranted.
IPC interventions may lead to a placebo effect on exercise performance, though studies have shown that the IPC intervention can surpass placebo effects (Carvalho, Concon, Meloni, De Souza, & Barroso, Citation2020). Sham-control trials were added to eliminate possible placebo effects (Cheung, Slysz, & Burr, Citation2019) but they were not effective enough to prevent placebo effects, leading to a trend to remove placebo-control trials from many studies (including ours) (O'Brien & Jacobs, Citation2021). Therefore, future studies are needed to minimize placebo and nocebo effects.
To date, the potential biochemical and physiological mediators of improved performance after IPC remain unclear. It was found that energy utilization could be improved by enhancing excitation-contraction coupling (Crisafulli et al., Citation2011); a higher activation of skeletal muscle has also been found in electromyographic (EMG) (Cruz, de Aguiar, Turnes, Pereira, & Caputo, Citation2015). Another study hypothesized that IPC could strengthen the anti-fatigue ability of the body by shifting the threshold level of the central nervous system (CNS) that inhibits body activity and improves the recruitment of motor units of skeletal muscles (Crisafulli et al., Citation2011), thus prolonging the exhaustive exercise performance. Therefore, based on the results of supramaximal intensity tests, we speculated that IPC could delay fatigue time and maintain the use of energy-rich phosphate compounds during exercise.
Limitations
We acknowledge several limitations in the present study. Firstly, no sham trial was included in our study to avoid possible placebo effects of IPC. Although IPC has been thought to have a placebo effect (Marocolo, da Mota, Pelegrini, & Appell Coriolano, Citation2015), it is hard to blind the involved participants to the inflating pressure or cuff position. Moreover, we ensured athletes had no prior knowledge of the mechanism of IPC and did not inform them that IPC could induce a positive/negative effect. Another possible limitation is that the equipment for IPC was used initially for KAATSU training, which suggests that the cuff width could be inappropriate (52 mm). We used NIRS to ensure the occlusion and postulate that a smaller cuff width may lead to discomfort with no significant negative effect. Finally, this study only included male athletes. The sex-related difference has been reported in the previous studies (Gibson, White, Neish, & Murray, Citation2013). It has been reported that females may hinder the ergogenic effect of IPC for greater reliance on oxidative metabolism compared to males (Paradis-Deschenes, Joanisse, & Billaut, Citation2016). To better investigate the ergogenic effect of IPC and avoid the possible confounding factor relating to sex, we recruited males first, which could limit the extrapolation of results to female athletes. In our future study, females and males will be included to compare the potential differing effects from IPC.
Conclusions
In conclusion, IPC in the limbs improved supramaximal time to exhaustion and MAOD in 400-meter athletes. RIPC and LIPC are both beneficial to anaerobic capacity and exhibited a similar yield in enhancing performances. Most importantly, IPC has the potential to improve anaerobic endurance capacity in well-trained athletes.
Disclosure statement
No potential conflict of interest was reported by the author(s).
Additional information
Funding
References
- Bailey, T. G., Jones, H., Gregson, W., Atkinson, G., Cable, N. T., & Thijssen, D. H. J. (2012). Effect of ischemic preconditioning on lactate accumulation and running performance. Medicine & Science in Sports & Exercise, 44(11), 2084–2089.
- Barbosa, T. C., Machado, A. C., Braz, I. D., Fernandes, I. A., Vianna, L. C., Nobrega, A. C. L., & Silva, B. M. (2015). Remote ischemic preconditioning delays fatigue development during handgrip exercise. Scandinavian Journal of Medicine & Science in Sports, 25(3), 356–364.
- Bertuzzi, R., Kiss, M. A. P. D. M., Damasceno, M., Oliveira, R. S. F., & Lima-Silva, A. E. (2015). Association between anaerobic components of the maximal accumulated oxygen deficit and 30-second Wingate test. Brazilian Journal of Medical and Biological Research, 48(3), 261–266.
- Bosquet, L., Duchene, A., Delhors, P. R., Dupont, G., & Carter, H. (2008). A comparison of methods to determine maximal accumulated oxygen deficit in running. Journal of Sports Sciences, 26(6), 663–670.
- Carvalho, L., Concon, V., Meloni, M., De Souza, E. O., & Barroso, R. (2020). Effects of resistance training combined with ischemic preconditioning on muscle size and strength in resistance-trained individuals. The Journal of Sports Medicine and Physical Fitness, 60(11), 1431–1436.
- Cheung, C. P., Slysz, J. T., & Burr, J. F. (2019). Ischemic Preconditioning: Improved Cycling Performance Despite Nocebo Expectation. International Journal of Sports Physiologica Perform, 15(3), 1–7.
- Clevidence, M. W., Mowery, R. E., & Kushnick, M. R. (2012). The effects of ischemic preconditioning on aerobic and anaerobic variables associated with submaximal cycling performance. European Journal of Applied Physiology, 112(10), 3649–3654.
- Cocking, S., Cable, N. T., Wilson, M. G., Green, D. J., Thijssen, D. H. J., & Jones, H. (2018). Conduit Artery Diameter During Exercise Is Enhanced After Local, but Not Remote, Ischemic Preconditioning. Frontiers in Physiology, 9.
- Cocking, S., Jones, H., Cable, N. T., & Thijssen, D. H. (2019). Chapter 19 - Enhancing Sports Performance Through Ischemic Preconditioning: Moderating Factors and Potential Mechanisms. In Rattan SIS & Kyriazis M (Eds.), Science of Hormesis in Health and Longevity (pp. 213–222). London: Academic Press.
- Crisafulli, A., Tangianu, F., Tocco, F., Concu, A., Mameli, O., Mulliri, G., & Caria, M. A. (2011). Ischemic preconditioning of the muscle improves maximal exercise performance but not maximal oxygen uptake in humans. Journal of applied physiology (Bethesda, Md. : 1985), 111(2), 530–536.
- Cruz, R. S. D. O., de Aguiar, R. A., Turnes, T., Pereira, K. L., & Caputo, F. (2015). Effects of ischemic preconditioning on maximal constant-load cycling performance. Journal of applied physiology (Bethesda, Md. : 1985), 119(9), 961–967.
- Cruz, R. S. S. O., de Aguiar, R. A., Turnes, T., Salvador, A. F., & Caputo, F. (2016). Effects of ischemic preconditioning on short-duration cycling performance. Applied Physiology, Nutrition, and Metabolism, 41(8), 825–831.
- de Oliveira Cruz, R. S., de Aguiar, R. A., Turnes, T., Salvador, A. F., & Caputo, F. (2016). Effects of ischemic preconditioning on short-duration cycling performance. Applied Physiology Nutrition and Metabolism, 41(8), 825–831.
- Gao, W., Gu, D., Lin, J., et al. (2013). Design of Simplified Test Scheme for Maximum Accumulated Oxygen Deficit and Its Validity Demonstration. China Sport Sciences, 33(02), 69–79+91.
- Gastin, P. B. (1994). Quantification of anaerobic capacity. Scandinavian Journal of Medicine & Science in Sports, 4, 91–112.
- Gibson, N., White, J., Neish, M., & Murray, A. (2013). Effect of ischemic preconditioning on land-based sprinting in team-sport athletes. International Journal of Sports Physiology and Performance, 8(6), 671–676.
- Griffin, P. J., Hughes, L., Gissane, C., & Patterson, S. D. (2019). Effects of local versus remote ischemic preconditioning on repeated sprint running performance. The Journal of Sports Medicine and Physical Fitness, 59(2), 187–194.
- Hanon, C., Lepretre, P.-M., Bishop, D., & Thomas, C. (2010). Oxygen uptake and blood metabolic responses to a 400-m run. European Journal of Applied Physiology, 109(2), 233–240.
- Heinen, N. M., Pütz, V. E., Görgens, J. I., Huhn, R., Grüber, Y., Barthuber, C., … Bauer, I. (2011). Cardioprotection by remote ischemic preconditioning exhibits a signaling pattern different from local ischemic preconditioning. Shock, 36(1), 45–53.
- Khaliulin, I., Fleishman, A. N., Shumeiko, N. I., Korablina, T., Petrovskiy, S. A., Ascione, R., & Suleiman, M.-S. (2019). Neuro-autonomic changes induced by remote ischemic preconditioning (RIPC) in healthy young adults: Implications for stress. Neurobiology of Stress, 11, 100189.
- Kraus, A., Pasha, E., Machin, D. R., Alkatan, M., Kloner, R. A., & Tanaka, H. (2015). Bilateral Upper Limb Remote Ischemic Preconditioning Improves Anaerobic Power. Open Sports Medicine, 9(4), 1–6.
- Lacour, J. R., Bouvat, E., & Barthelemy, J. C. (1990). Post-competition blood lactate concentrations as indicators of anaerobic energy expenditure during 400-m and 800-m races. European Journal of Applied Physiology and Occupational Physiology, 61(3-4), 172–176.
- Lindsay, A., Petersen, C., Blackwell, G., Ferguson, H., Parker, G., Steyn, N., & Gieseg, S. P. (2017). The effect of 1 week of repeated ischaemic leg preconditioning on simulated Keirin cycling performance: a randomised trial. BMJ Open Sport & Exercise Medicine, 3(1), e000229.
- LI Yong-Ming, M. C. (2014). Energy Contribution in Competitive Sports—Error in need of correction. China Sport Science, 34, 93–97.
- Loukogeorgakis, S. P., Panagiotidou, A. T., Broadhead, M. W., Donald, A., Deanfield, J. E., & MacAllister, R. J. (2005). Remote ischemic preconditioning provides early and late protection against endothelial ischemia-reperfusion injury in humans: role of the autonomic nervous system. Journal of the American College of Cardiology, 46(3), 450–456.
- Marocolo, M., da Mota, G., Pelegrini, V., & Appell Coriolano, H.-J. (2015). Are the Beneficial Effects of Ischemic Preconditioning on Performance Partly a Placebo Effect? International Journal of Sports Medicine, 36(10), 822–825.
- Medbo, J. I., Mohn, A. C., Tabata, I., Bahr, R., Vaage, O., & Sejersted, O. M. (1988). Anaerobic capacity determined by maximal accumulated O2 deficit. Journal of applied physiology (Bethesda, Md. : 1985), 64(1), 50–60.
- Minahan, C., Chia, M., & Inbar, O. (2007). Does power indicate capacity? 30-s Wingate anaerobic test vs. maximal accumulated O2 deficit. International Journal of Sports Medicine, 28(10), 836–843.
- Murry, C. E., Jennings, R. B., & Reimer, K. A. (1986). Preconditioning with ischemia: a delay of lethal cell injury in ischemic myocardium. Circulation, 74(5), 1124–1136.
- Noordhof, D. A., de Koning, J. J., & Foster, C. (2010). The maximal accumulated oxygen deficit method: a valid and reliable measure of anaerobic capacity? Sports Medicine, 40(4), 285–302.
- O'Brien, L., & Jacobs, I. (2021). Methodological Variations Contributing to Heterogenous Ergogenic Responses to Ischemic Preconditioning. Frontiers in Physiology, 12, 656980.
- Pang, C. Y., Yang, R. Z., Zhong, A., Xu, N., Boyd, B., & Forrest, C. R. (1995). Acute ischaemic preconditioning protects against skeletal muscle infarction in the pig. Cardiovascular Research, 29(6), 782–788.
- Paradis-Deschenes, P., Joanisse, D. R., & Billaut, F. (2016). Sex-Specific Impact of Ischemic Preconditioning on Tissue Oxygenation and Maximal Concentric Force. Frontiers in Physiology, 7, 674.
- Paull, E. J., & Van Guilder, G. P. (2019). Remote ischemic preconditioning increases accumulated oxygen deficit in middle-distance runners. Journal of applied physiology (Bethesda, Md. : 1985), 126(5), 1193–1203.
- Peng, L. (2011). Doubtful Maximum Oxygen Uptake-test Methods and Criteria. China Sport Sciences, 31(07), 85–91.
- Salvador, A. F., De Aguiar, R. A., Lisbôa, F. D., Pereira, K. L., Cruz, R. S. d. O., & Caputo, F. (2016). Ischemic Preconditioning and Exercise Performance: A Systematic Review and Meta-Analysis. International Journal of Sports Physiology and Performance, 11(1), 4–14.
- Spencer, M. R., & Gastin, P. B. (2001). Energy system contribution during 200- to 1500-m running in highly trained athletes. Medicine and Science in Sports and Exercise, 33(1), 157–162.
- Tanner, R., & Gore, C. (2013). Physiological Tests for Elite Athletes. (2nd Ed). Champaign,IL: Human Kinetics.
- Yellon, D. M., & Downey, J. M. (2003). Preconditioning the myocardium: from cellular physiology to clinical cardiology. Physiological Reviews, 83(4), 1113–1151.