ABSTRACT
Although the acute effect of exercise on behavioral cognitive performance is well-documented in the exercise psychology field, a comprehensive evaluation on neuroelectric brain activity that determines healthy cognitive functioning following acute exercise is lacking. This systematic review included 39 studies examining acute exercise effects on P3 of event-related potential through its amplitude and latency, which reflect the amounts of attentional resources allocated to and the processing speed for categorizing a stimulus. Exercise has small effects on increasing amplitude and decreasing latency. The amplitude effect was moderated by age and the type, intensity, and duration of exercise, with a smaller effect being observed for individuals aged ≤18 and 19–35 than >60 years, for high-intensity than moderate-intensity exercise, for high-intensity interval training exercise than aerobic, resistance, and combined exercise, as well as for exercise lasting ≤10 and 11–20 than exercise lasting 21–30 min. The latency effect was moderated by exercise duration, with 11–20 min exercise showing a smaller effect than exercise lasting ≤10 min. These results demonstrated that acute exercise enhances allocation of attentional resources and processing speed needed to implement cognitive processes underlying goal-directed behavior. Further, these effects may be manipulated through targeting specific age groups and prescribing specific exercise parameters.
Introduction
Exercise has long been recognized as an important health behavior for mental health and well-being (Craft & Landers, Citation1998; Petruzzello et al., Citation1991; Rebar et al., Citation2015). A growing number of recent research centered in the field of exercise psychology has begun to show convergent evidence that long-term exercise engagement has positive influences on cognitive functions (Chen et al., Citation2020; Ludyga et al., Citation2020; Tomporowski & Pesce, Citation2019; Yu et al., Citation2021). These benefits have been extended to acute cognitive enhancements during the recovery period following an acute bout of exercise (Chang et al., Citation2019; Chang & Etnier, Citation2015). Meta-analyses have provided compelling evidence that acute exercise has a small-to-moderate positive effect on cognitive function across the lifespan (Chang et al., Citation2012; Lambourne & Tomporowski, Citation2010; Loprinzi et al., Citation2019; Ludyga et al., Citation2016; Moreau & Chou, Citation2019; Oberste et al., Citation2019; Sibley & Etnier, Citation2003; Wilke et al., Citation2019). Nonetheless, previous meta-analytic reviews primarily focused acute exercise effects on behavioral cognitive performance, with only one exception that included children’s neural functioning outcomes (Meijer et al., Citation2020), limiting our understanding of its effects on the underlying brain function that support healthy cognitive functioning across the lifespan.
The growing interest in human brain since the launch of several research initiatives around the world (Grillner et al., Citation2016), such as the Brain Research through Advancing Innovative Neurotechnologies (BRAIN) since 2013 in United States, the Human Brain Project (HBP) since 2015 in Europe Union, and the Brain Mapping by Integrated Neurotechnologies for Disease Studies (Brain/MINDS) since 2014 in Japan, have stimulated exercise psychologists’ effort to investigate the acute neurocognitive responses to exercise using a variety of neuroscience approaches in the past decades. Of these techniques, event-related potential (ERP) has attracted considerable attention because of its well-established associations with information processing underlying goal-directed human behaviors (Fabiani et al., Citation2007). Specifically, the acute exercise literature has focused on the simple, choice, and/or cued reaction time requiring attention and its underlying alerting and orienting networks (Petersen & Posner, Citation2012). Another major focus is executive function, which refers to an adaptive and flexible cognitive processes encompassing inhibition, updating, and shifting in support of goal-directed behavior (Miyake & Friedman, Citation2012). Given the increasing number of studies utilizing ERP to assess neuroelectric alteration associated with attention and executive function performance in response to exercise (Chang, Citation2016; Kao et al., Citation2020; Pedroso et al., Citation2017), the current study sought to provide the meta-analytic evidence to determine whether and how acute exercise influences P3, a neuroelectric marker of brain functioning needed to implement various cognitive processes.
The P3 event-related potential component
Derived from electroencephalography (EEG)Footnote1, ERP reflects the patterns of neuroelectric activation encompassing perceptual and cognitive processes in response to, or in preparation for, a stimulus or response (Fabiani et al., Citation2007). During the stimulus evaluationFootnote2, the P3 (or P300, P3b) is an endogenous and stimulus-locked positive-going deflection peaking approximately at or after 300 ms following the stimulus onset (Polich, Citation2007). Because P3 captures covert information processing at the neuroelectric levels and reflects brain function and development (Riggins & Scott, Citation2020; van Dinteren et al., Citation2014), it has been widely used to evaluate neurocognitive effects of exercise (Hillman et al., Citation2012).
P3 is theoretically explained by the context-updating theory (Donchin & Coles, Citation1988), which posits that when the current attributes of a stimulus differ from the mental representation stored in working memory, attentional-driven updating of this memory representation is processed concurrently with the elicitation of P3. Further, P3 might reflect the neuronal activities involved in context-updating in proportional to the attentional demands during which conscious and effortful responses toward the stimuli have to be made (Kok, Citation2001; Näätänen, Citation1990). These convergent evidences have led to the hypothesis that P3 is a neuroelectric manifestation of neural inhibition to task-irrelevant neuronal activity in facilitating the allocation of attentional resources and the evaluative process on task-relevant stimulus (Polich, Citation2007).
P3 measurement and its associated factors
P3 is quantified by its amplitude and latency. P3 amplitude (μV), which is the difference between the largest positive peak of the ERP within a window (i.e. 300–700 ms) and the mean pre-stimulus baseline, indexes the amount of attentional resource available toward stimulus evaluation and/or updating the memory representation (Polich, Citation2007). The P3 latency (ms), which is the time from stimulus onset to the P3 peak, indexes the timing of stimulus evaluation and cognitive processing efficiency (Verleger, Citation1997). Although these P3 indices modulate as a function of the type of P3-eliciting task and the within-task stimulus characteristics (Polich, Citation2007; Verleger, Citation2020), increased amplitude and decreased latency, with exceptions of P3 modulation during specific tasks that require priming, efficiency, and complex cognitive strategies (i.e. cue-related, dual-task) (Verleger, Citation2020), are generally related to superior cognitive performance (Brydges et al., Citation2014; Kok, Citation2001; Polich, Citation2007). Further, the P3 topographic analysis indicated increasing amplitude and decreasing latency from the anterior to the posterior brain regions, with maximum amplitude typically observed at the centroparietal region on the midline electrodes (Luck, Citation2005; Polich, Citation2007). Such a scalp distribution of P3 overlaps with the P3 generators centered at the cingulate cortex and temporoparietal areas (Kim, Citation2014; Polich, Citation2003; Soltani & Knight, Citation2000). Within these potential neural origins, locus coeruleus-norepinephrine (LC-NE) system is an arousal-regulating center that exerts modulatory effects on P3 in facilitating stimulus evaluation and decision-making processing of behaviorally relevant events (Nieuwenhuis et al., Citation2005). Indeed, arousal levels contribute to attentional activation and information processing (Kok, Citation1997; Nieuwenhuis et al., Citation2005), with higher arousal levels inducing larger P3 amplitude (Duncan et al., Citation2009; Polich, Citation2007). Recent research further identified the association of LC activation with P3 (Nieuwenhuis et al., Citation2005), with an intermediate LC activation level associating with the largest P3 amplitude (LoTemplio et al., Citation2021; Murphy et al., Citation2011).
P3 is also known for its developmental changes throughout the lifespan. P3 amplitude increases in early development until its peak during young adulthood, followed by gradual decreases with age (van Dinteren et al., Citation2014). Reversely, P3 latency decreases throughout childhood development and begins to increase at middle adulthood (van Dinteren et al., Citation2014). Further, P3 has been identified as a neuroelectric expression of sex difference (Hirayasu et al., Citation2000; Melynyte et al., Citation2017; Melynyte et al., Citation2018), individual difference related to cognitive disability (Pontifex et al., Citation2013), and aerobic fitness (Kao et al., Citation2020; Raine et al., Citation2018). Further, extensive research has established P3 as a neural marker relevant to psychological and behavioral outcomes including, but not limited to, personality (Cahill & Polich, Citation1992), motivation (Carrillo-de-la-Peña & Cadaveira, Citation2000), problem behaviors (Petersen et al., Citation2018), and academic achievement (Polich & Martin, Citation1992). The sensitivity of P3 as a neuroelectric marker to reflect information processing, arousal activation, and individual difference in neurocognitive development along with its relevance to a host of psycho-behavioral outcomes in the field of exercise psychology renders P3 measures useful for understanding brain functioning underlying cognitive performance in response to arousal-inducing interventions such as exercise.
Acute exercise and P3
Since Hillman’s first research examining P3 in response to acute exercise in comparison with a physically inactive condition (Hillman et al., Citation2003), many research groups replicated the acute increases in P3 amplitude and/or decreases in P3 latency following exercise (Chang et al., Citation2017; Hsieh et al., Citation2018; Pontifex et al., Citation2015; Tsai et al., Citation2016; Won et al., Citation2017). However, these patterns of P3 modulation were absent from some studies (Ligeza et al., Citation2018; Xie et al., Citation2020; Zhou & Qin, Citation2019) and reversed in the others (Kamijo et al., Citation2004; Kao et al., Citation2017). The most cited interpretation of these discrepancies has been the experimental designs, especially the methodological difference related to P3-eliciting tasks, exercise interventions, and target populations.
Task paradigm. Although P3 is a task-dependent neuroelectric activity that modulates as a function of cognitive domains as well as the frequency of the within-task stimulus (Polich, Citation2007; Verleger, Citation2020), exercise-induced modulations of P3 have been reported across different neurocognitive tasks presenting stimulus with various degrees of predictability (Aly & Kojima, Citation2020; Bae & Masaki, Citation2019; Chacko et al., Citation2020; Chang et al., Citation2017; Chu et al., Citation2017; Drollette et al., Citation2014; Hillman et al., Citation2009; Kao et al., Citation2018; Pontifex et al., Citation2021). In the acute exercise and P3 literature, these tasks are categorized into attentional and executive function domains (Etnier & Chang, Citation2009), including tasks requiring simple stimulus discrimination (Jain et al., Citation2014; Pontifex et al., Citation2015), cued-attentional anticipation (Chang et al., Citation2015; Tsai, Chen, et al., Citation2014) and executive processes of inhibitory control, working memory, and cognitive flexibility (Kao et al., Citation2020). Despite increased P3 amplitude and/or decreased P3 latency have been observed during various attention and executive function tasks following acute exercise in the literature (Kao et al., Citation2020), limited studies empirically investigated whether the modulation of P3 in response to acute exercise is similar or differential during tasks requiring different cognitive domains and presenting P3-eliciting stimulus at different probabilities.
Exercise characteristics. Exercise is a physical stressor to increase not only peripheral cardiovascular arousal but also neuronal activation (Critchley et al., Citation2000; McMorris et al., Citation2016). Because arousal level induced by exercise depends on its type, intensity, and duration (Brisswalter et al., Citation2002; Kenney et al., Citation2015; Kliszczewicz et al., Citation2016), manipulating these characteristics is expected to modulate arousal-mediated P3. Although aerobic exercise has been repeatedly shown to increase P3 amplitude (Kao et al., Citation2020), studies including different exercise modalities (i.e. coordination, resistance) showed similar increases in P3 amplitude (Lind et al., Citation2019; Ludyga et al., Citation2017; Tsai, Pan, et al., 2021; Tsai et al., Citation2018; Wen & Tsai, Citation2020; Wu et al., Citation2019; Xie et al., Citation2020). Some studies, however, found disproportionally larger (Ludyga et al., Citation2017) or selectively increased (Kao et al., Citation2017; Kao et al., Citation2018; O'Leary et al., Citation2011) P3 amplitude following aerobic compared to other exercise. These differential effects could also attribute to the intensity of exercise, as P3 measures differed following exercise at different intensities (Kamijo et al., Citation2004; Kamijo et al., Citation2007; Kamijo et al., Citation2009). Specifically, research has indicated an inverted-U (Kamijo et al., Citation2004; Kao et al., Citation2018) or inverted-J (Kamijo et al., Citation2007; Kao et al., Citation2017) shaped relationship between exercise intensity and P3 amplitude, suggesting that the largest P3 amplitude may be achieved at moderate arousal level through moderate-intensity exercise. Though these interrelationships between intensity, arousal, and P3 amplitude support the central role of arousal to the neurocognitive benefits of acute exercise, exercise-induced changes in P3 amplitude were not always intensity-dependent (Kamijo et al., Citation2009; Tsai, Wang, et al., Citation2014) and their relations with exercise duration have remained unexplored. Of the fewer studies examining the associations of P3 latency with exercise intensity, shorter latency was observed following high-intensity interval training (HIIT) than aerobic exercise (Kao et al., Citation2017; Kao et al., Citation2018). These seemingly selective acute changes in P3 following exercise prescribed with certain characteristics stem from only a small portion of the literature directly comparing multiple treatments, warranting a need to meta-analytically compare available effect sizes by different exercise parameters employed. Lastly, because the type of non-exercise control activity and the timing of exercise before P3 assessment influence the arousal levels to be contrasted with exercise interventions at a temporal proximity of P3 assessment, how these factors related to exercise characteristics affect exercise-induced modulation of P3 warrants further investigation (Kao et al., Citation2020).
Individual difference. Previous studies have shown that exercise increased P3 amplitude (Chu et al., Citation2017; Hsieh et al., Citation2018) and decreased P3 latency (Kamijo et al., Citation2009) regardless of age and the presence of attention deficit hyperactivity disorder (ADHD) (Ludyga et al., Citation2017; Pontifex et al., Citation2013). However, some studies showed increases in amplitude following exercise selectively in younger (Kamijo et al., Citation2009), more aerobically fit (Tsai et al., Citation2016; Tsai, Chen, et al., Citation2014), and low cognitively competent (Drollette et al., Citation2014) individuals than their older, less fit, and more cognitively competent counterparts. Further, various research groups have focused on modulations of P3 only in males following exercise (Chu et al., Citation2017; Du Rietz et al., Citation2019; Hsieh et al., Citation2018; Jain et al., Citation2014; Kamijo et al., Citation2009; Ligeza et al., Citation2018; Tsai et al., Citation2016; Won et al., Citation2017) because of the neuroanatomical and neurochemical difference underlying the generation of P3 between sexes (Melynyte et al., Citation2018). Taken together, although individual difference factors that may moderate acute exercise effects on P3 have been identified, the speculated moderation effect remains to be comprehensively determined.
Purpose
While exercise has been known for its cognitive benefits, the current understanding of exercise-induced effects on brain function remains in its infancy. In response to the U.S. guideline’s call for determining the effects of acute exercise on brain function and potential moderators (Chang et al., Citation2019; Erickson et al., Citation2019; Physical Activity Guidelines Advisory Committee, Citation2018), a few earlier systematic reviews exist to synthesize the acute effect of on P3 (Chang, Citation2016; Kao et al., Citation2020; Meijer et al., Citation2020; Pedroso et al., Citation2017). Although one of these reviews on a variety of neurophysiological outcome measures demonstrated a small effect (d = 0.42) of acute physical activity effects on P3 amplitude (Meijer et al., Citation2020), this meta-analytic finding was obtained only from studies in 5–12 years old children and did not include potential moderators and P3 latency in the analysis. The present systematic review aimed to meta-analytically review studies using randomized or non-randomized controlled trial and within-subject crossover designs to evaluate the effects of acute exercise as compared with non-exercise control activities on P3 amplitude and latency as well as to conduct moderator analyses to test whether these effects were influenced by task paradigm, exercise characteristics, and individual difference. Identifying these moderators would inform the design of exercise programs that target brain functioning underlying domains of cognitive processes in different populations. This study is expected to make a significant and practically relevant contribution by (1) offering guidance on the use of exercise for altering brain function that supports cognitive performance and (2) providing neuroscience evidence to refine sport and exercise psychology theories on human arousal and performance in response to physiological stressors.
Materials and methods
Study eligibility criteria
The meta-analysis was prospectively registered in the International Prospective Register of Systematic Reviews (CRD42020148238) and conducted in accordance with the PRISMA statement (Moher et al., Citation2009). We included studies that examined the effects of acute exercise on P3/P3b/P300 in human samples published in English. The inclusion criteria were: (1) Participants: healthy and cognitive impaired participants with no age limitation; (2) Interventions: the studies included acute exercise intervention; ‘acute’ was defined as ‘performed on a single day’ and ‘exercise’ was defined as ‘a type of physical activity consisting of planned, structured, and repetitive bodily movement done to improve and/or maintain one or more components of physical fitness’ according to the American College of Sports Medicine (American College of Sports Medicine, Citation2014); (3) Study designs: intervention study using between-subject pretest-posttest and posttest only, within-subject crossover pretest-posttest and posttest only (Pontifex et al., Citation2019); (4) Control groups/conditions: studies included at least a non-exercise condition/group as a control; (5) Outcome measures: studies assessed P3/P3b/P300 by ERPs. We excluded conference abstracts, dissertations, theses, and articles from non–peer-reviewed journals. Additionally, case report and case series research designs were excluded.
Information sources, search strategies, and study selection
A systematic search of the literature was conducted in databases of PubMed, Scopus, and Web of Science from inception to November 24, 2021. The searches combined the terms of acute exercise with P3: (‘physical activity’ OR ‘physical activities’ OR ‘physical conditioning’ OR ‘resistance exercise’ OR ‘strength training’ OR ‘walking’ OR ‘exercise’ OR ‘acute exercise’ OR ‘a single bout’ OR ‘sport’ OR ‘aerobic activity’ OR ‘aerobic activities’ OR ‘cardiovascular activity’ OR ‘cardiovascular activities’ OR ‘endurance activity’ OR ‘endurance activities’ OR AND ‘event-related potential’ OR ‘ERP’ OR ‘electroencephalography’ OR ‘EEG’ OR ‘P3’ OR ‘P3b’ OR ‘P300’). Additionally, other sources, such as references from relevant review articles (Kao et al., Citation2020; Ludyga et al., Citation2020), were included as additional records. The search results were independently screened for eligibility and excluded articles with irreverent title or abstract by two reviewers (SCK, FTC). Full-text articles were received when the title or abstract met the inclusion criteria. When any disagreement occurred, the consensus was reached through consulting a third senior reviewer (YKC).
Data collection and extraction process
Data extraction was piloted on three eligible articles among three reviewers (SCK, FTC, ESD) to ensure consistency. The three reviewers then independently (with a 33% overlap on articles reviewed) extracted the P3 amplitude and latency outcomes along with the following information: (1) first author’s name and publication year; (2) experimental design; (3) characteristics of the study sample and experiment; (4) P3-eliciting task; and (5) characteristics of exercise interventions. The three reviewers met to compare extracted data and resolved disagreements through discussion.
Means and standard deviations (SDs) of P3 amplitude and latency values during P3-eliciting cognitive tasks were extracted from midline electrodes (i.e. Fz, FCz, Cz, CPz, Pz, POz, Oz) included in each study’s original analysis. For studies with multiple P3 outcomes from a single task, only the task condition with the highest demand (as determined by reviewers, Table S1) on the respective cognitive domain was extracted.
For each cognitive task condition of interest in each sample group in a given study, we first calculated the mean P3 amplitude value at each of the included midline electrodes averaged across interventions and testing time points (i.e. the averaged amplitude across exercise and control conditions during the pretest and posttest incongruent condition of a flanker task at Fz, Cz, and Pz electrode sites, respectively, for a within-subject study using pretest/posttest comparisons, a flanker task, and Fz, Cz, and Pz electrode sites in the original analysis). The purpose of this step was to allow the identification of the midline electrode that shows the largest P3 amplitude. Next, P3 amplitude and latency of that identified electrode were used to calculate effect sizes for the study. This data-driven approach was chosen to enable the examination on the actual P3 for each study sample. Two separate meta-analyses were performed to investigate acute exercise effects on P3 amplitude and latency. Studies (n = 10) that only reported P3 amplitude were not included in the analysis on P3 latency.
Studies that did not report necessary information were formed as a ‘Not Reported’ category in the moderator analysis. Experimental design was coded based on a recent review with the pre-experimental design being excluded (Pontifex et al., Citation2019), including randomized between-subjects pretest-posttest comparison, non-randomized between-subjects pretest-posttest comparison, randomized between-subjects posttest comparison, within-subjects crossover posttest comparison, and within-subjects crossover pretest-posttest comparison. Studies including more than one group were considered as between-subjects design studies only when at least one group was assigned to receive a control activity intervention in comparison with the exercise group(s).
Categorical variables were created based on (1) task paradigm, including cognitive domain (attention, inhibitory control, working memory, cognitive flexibility) and stimulus probability (<50%, 50%, >50% –<100%, 100%), (2) exercise characteristics, including type (aerobic, HIIT, resistance, coordination, and combined), intensity (low, moderate, and high according to reported objective [e.g. heart rate, one-repetition maximum] or subjective [e.g. rating of perceived exertion]) measuresFootnote3 (American College of Sports Medicine, Citation2014), duration (≤10, 11–20, 21–30 min), volume (≤100, 101–200, ≥201 metabolic equivalent [MET, 3.5 ml O2/kg/min] estimated by the intensity and duration of exercise adjusted for age) (American College of Sports Medicine, Citation2014), timing before P3 assessment (≤15, 15–30, ≥31 min), and control activity (baseline, disengagement, cognitive engagement, active), and (3) individual difference, including sex (male, female, both), age (≤18, 19–35, 36–60, >60), aerobic fitness (low [<34%], medium [34–66%], high [>66%])Footnote4, and cognitive ability (healthy, impaired). The range for variables that were converted into categories were specified a priori, with the exception of timing before P3 assessment and exercise volume which were determined based on distributions.
Risk of bias assessment
Three previously trained reviewers (SCK, FTC, ESD) independently assessed the quality of the studies selected. The initial agreement among reviewers’ risk of bias scores was strong (Fleiss Multirater Kappa = .850). Disagreements between reviewers were solved in a consensus meeting with the senior reviewer (YKC). Due to the unique experimental designs employed to evaluate acute exercise effects on cognition studies, the risk of bias was assessed using an adapted PEDro checklist (https://www.pedro.org.au/), including (1) random allocation; (2) concealed allocation; (3) baseline comparability; (4) blinded assessors; (5) between-group analysis; (6) outcome point estimates; (7) exercise description; and (8) P3 assessment description. A similar protocol was used in the issue of acute exercise and cognition (Loprinzi et al., Citation2019). Reviewers assigned 0 when the study did not meet a criterion (or not clear) and 1 when a criterion was met. Studies using within-subject designs were assigned 1 for random allocation, concealed allocation, baseline comparability, and between-group analysis. Publication bias was assessed using the Funnel plot and Egger’s regression.
Data analysis
Means and SDs of P3 amplitude and latency data were used to calculate effect sizes, accounting for specifics of the research design. Mean gain scores for each treatment, post-treatment SDs, and sample size were used to determine effect sizes in randomized and non-randomized between-subject pretest-posttest comparison studies or within-subjects crossover pretest-posttest comparison studies. Mean, SD, and sample size in each group were used to calculate effect sizes for the randomized between-subject posttest comparison studies. For the within-subjects crossover posttest comparison studies, effect sizes were calculated using means and SDs of difference scores between exercise and control conditions. When latency values were used as the outcome variable the effect size obtained from study data was multiplied by −1. Consequently, positive effect sizes indicate either increased P3 amplitude or decreased P3 latency in the treatment group/condition than in the control group/condition. The calculated effect sizes were transformed to the bias-corrected Hedges’ g.
The overall meta-analysis, subgroup analysis, and moderator analysis were conducted in the R software environment using the metafor package (Viechtbauer, Citation2010). To account for the dependency between effect sizes, a multilevel analysis was conducted (see Gucciardi et al., Citation2022 for review; Moreau & Chou, Citation2019) using a procedure that adds random effects at each level of possible dependency to reduce inflation while preserving valuable information provided by studies that reported multiple effect sizes (Viechtbauer, Citation2010). The multilevel analysis had two clustering variables (i.e. levels). First, we modeled dependency at the study-design level (i.e. within-subjects and between-groups designs). Second, we modeled dependency at the sample level (i.e. within-samples reporting results in multiple tests and/or samples with two or more comparison groups/conditions). For example, a group undergoing three sessions (i.e. control, low-intensity exercise, and high-intensity exercise) included dependency across all outcome scores given that the same control session score was compared with both the low-intensity and high-intensity session scores.
To assess heterogeneity, we conducted a visual inspection of the forest plot to appraise the variation in study-level effect sizes and the amount of overlap in the 95% confidence intervals. We used Cochran’s Q test to assess the extent to which all studies shared a common effect size (α = 0.10). Additionally, we considered the percentage of total variation in estimated effects due to between-study variation (I2); a value of 25% was considered to reflect low heterogeneity, 50% to reflect moderate heterogeneity, and 75% to reflect high heterogeneity (Higgins et al., Citation2003). To assess publication bias, we conducted a visual inspection of the distribution of the funnel plot of meta-analyzed studies and performed the Egger’s test (α = 0.05) of the intercept (Egger et al., Citation1997). The potential impact of any publication bias on the observed effect was addressed by the trim-and-fill approach (Duval & Tweedie, Citation2000).
Results
Characteristics of included studies
Our initial search retrieved 15,308 records from databases and 12 records from other sources. 6,749 records remained after de-duplication. Following the screening of title and abstract, 66 potentially eligible articles were identified. Of these studies, 20 were excluded after full-text assessments because of (1) pre-experimental and single-subject design designs (n = 13) (Dimitrova et al., Citation2017; Duzova et al., Citation2005; Gowsi et al., Citation2016; Grego et al., Citation2004; Kota et al., Citation2013; Kumar et al., Citation2010; Magnie et al., Citation2000; Nakamura et al., Citation1999; Popovich & Staines, Citation2015; Scanlon et al., Citation2017; Shibasaki et al., Citation2019; Tsai, Hsieh, et al., Citation2021; Yagi et al., Citation1999); (2) reanalysis of previously reported data (n = 1) (Drollette et al., Citation2014); (3) no P3 outcomes at midline electrodes (n = 3) (Stroth et al., Citation2009; Wang et al., Citation2016; Wu et al., Citation2021); (4) reporting P3 during exercise or in relation to fitness (n = 2) (Keye et al., Citation2021; Liebherr et al., Citation2021); and (5) using P3 to index appetite responses to food cues (n = 1) (Carbine et al., Citation2020). Forty-six remaining studies were reviewed and disagreements were solved in a meeting between reviewers and the senior expert (YKC). Because data from 7 studies were not available from the full-text and study authors after 3 attempts of contact (Bailey et al., Citation2021; Chu et al., Citation2015; Fearnbach et al., Citation2016; Fearnbach et al., Citation2017; Kumar et al., Citation2012; Swatridge et al., Citation2017; Winneke et al., Citation2019), 39 studies were included in the meta-analysis. The flow diagram of the study selection process following Preferred Reporting Items for Systematic Review and Meta-analysis (PRISMA) guidelines is shown in . Descriptive statistics of risk of bias measures were shown in and Fig. S1.
Table 1. Detailed risk of bias quality assessment of each criterion for all studies included (n = 39).
A summary of study details is shown in . Most included studies employed within-subject crossover posttest comparison design (n = 23), followed by within-subject crossover pretest-posttest comparison design (n = 7), randomized between-subject pretest-posttest comparison design (n = 6), non-randomized between-subject pretest-posttest comparison design (n = 2), and randomized between-subject posttest comparison design (n = 1). One study assessed P3 in multiple cognitive domains (Chang et al., Citation2015). In a study using two variations of GoNogo tasks, effect sizes obtained during a modified GoNogo task to elicit craving for methamphetamine in methamphetamine-dependent individuals were excluded (Wang et al., Citation2015). Twenty-five studies investigated one exercise intervention while 14 studies investigated two or more different interventions. Thirty-one studies included one sample group and eight studies included two developmentally different groups. Because some studies included multiple cognitive domains, exercise interventions, and samples, a total of k = 67 effect sizes were analyzed. Of the 67 effects, P3 amplitude was peaked at Cz (k = 20), CPz (k = 12), Pz (k = 31), POz (k = 2), and Oz (k = 2). and summarizes the overall and moderator analyses on amplitude and latency.
Table 2. Summary of the experimental studies examining the acute effect of exercise intervention on P3 (n = 39)
Table 3. Summary of overall and moderator analyses on P3 amplitude.
Table 4. Summary of overall and moderator analyses on P3 latency.
P3 amplitude
Overall Effect. The meta-analytic estimate of this effect was small-to-moderate (g = 0.315) (), with moderate heterogeneity (I2 = 50%). The visual inspection of the funnel plot () was suggestive of asymmetry at the bottom and the findings in Egger’s test was statistically significant (Z = 4.627, p < .001). The imputed overall effect size using the trim-and-fill method was smaller (g = 0.191, CI = 0.074–0.309), with larger heterogeneity (I2 = 75%) than the meta-analytic estimate, Q(78) = 241.3, p < .001.
Figure 2. Forest plot of amplitude effect sizes for all included studies. The sizes of the squares represent relative sample size. The diamond at the bottom represents the overall effect.
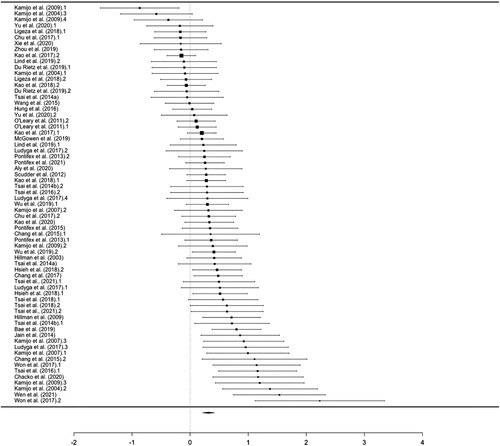
Figure 3. Funnel plot of amplitude effect sizes for studies in the meta-analysis. Each dot represents an individual effect size and is plotted as a function of standard error. The black dots represent effect sizes obtained from included studies. The white dots represent imputed effect sizes after adjusting for publication bias using the trim-and-fill method. The vertical line represents the random-effect models estimate.
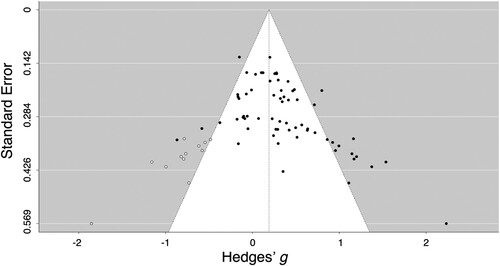
Moderator Analysis. The effect of exercise on P3 amplitude was not moderated by experimental design and task paradigm, and individual difference factors, except for age such that samples of individuals older than 60 years showed a larger effect than samples of individuals at or below 18 years (Mdiff = 0.537, p = .006) and between 19 and 35 years (Mdiff = 0.401, p = .013). No study-level effect size for the 36–60 years subgroup was available. Analysis on exercise type showed a significant moderation effect, with HIIT having a smaller effect than aerobic (Mdiff = 0.303, p = .007), resistance (Mdiff = 0.449, p = .028), and combined exercise (Mdiff = 0.445, p = .022). Exercise intensity was a significant moderator, with moderate-intensity exercise showing a significantly larger positive effect than high-intensity exercise (Mdiff = 0.317, p < .001) and a marginally larger positive effect than low-intensity (Mdiff = 0.243, p = .059). Exercise duration was a significant moderator, with exercise lasting 21–30 min showing a significantly larger positive effect than exercise lasting 11–20 min (Mdiff = 0.262, p = .020) and ≤ 10 min (Mdiff = 0.487, p = .009). Exercise volume, timing before P3 assessment, and control activity did not moderate effect sizes.
Analysis on risk of bias criteria that were not met by all studies showed no moderation effect for random allocation, Q(1) = 0.257, p = .612, concealed allocation, Q(1) = 2.143, p = .143, baseline comparability, Q(1) = 0.975, p = .324, and between-group analysis, Q(1) = 0.040, p = .842, except for P3 assessment description, Q(1) = 6.093, p = .013, with studies without describing their assessment of P3 amplitude appropriately showing a larger effect size than studies that met (Mdiff = 0.240). All studies met the outcome point estimates and exercise description criteria.
P3 latency
Overall Effect. The meta-analytic estimate of this effect was small (g = 0.146) (), with low-to-moderate heterogeneity (I2 = 44%). Both the visual inspection of the funnel plot () and the result of the Egger’s test (Z = 0.842, p = .400) did not provide evidence for publication bias.
Figure 4. Forest plot of latency effect sizes for included studies reporting P3 latency. The sizes of the squares represent relative sample size. The diamond at the bottom represents the overall effect.
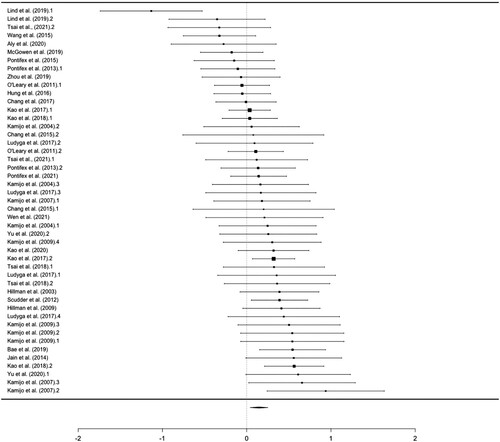
Figure 5. Funnel plot of latency effect sizes for studies in the meta-analysis. Each dot represents an individual effect size and is plotted as a function of standard error. The vertical line represents the random-effect models estimate.
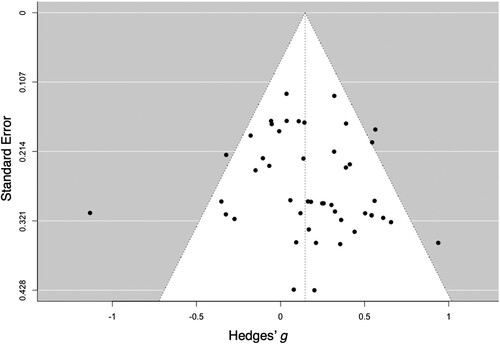
Moderator Analysis. Effect sizes were not moderated by experimental design, task paradigm, and individual difference factors. Exercise duration was a significant moderator, with exercise lasting ≤ 10 min showing a positive effect that was larger than that of exercise lasting 11–20 min (Mdiff = 0.306, p = .044). No moderation effects were found for exercise type, exercise intensity, exercise volume, timing before P3 assessment, and control activity.
Analysis on risk of bias criteria that were not met by all studies showed no moderation effect for baseline comparability, Q(1) < 0.001, p = .986, blinded assessors, Q(1) < 0.001, p = .988, and P3 assessment description, Q(1) = 0.074, p = .786, except for concealed allocation, Q(1) = 4.108, p = .043, with the summary effect for studies judged to be at higher risk of bias arising from inappropriate allocation being larger than studies judged to be at lower risk (Mdiff = 0.298). All studies met the random allocation, between-group analysis, outcome point estimates, and exercise description criteria.
Discussion
Novel to the existing exercise psychology literature, this study meta-analytically determined the acute exercise effects on P3. Specifically, amplitude and latency of P3 were investigated to index the amounts of attentional resources available for stimulus evaluation and the speed of stimulus processing, respectively. Although these P3 indices have been increasingly used to determine the temporal cognition-enhancing effect of exercise, the existing literature is characterized by methodological variations and mixed findings. Thus, experimental design, task paradigm, exercise characteristic, and individual difference were examined as moderators in the current meta-analysis. Study quality measures were examined and indicated a low risk of bias, except for two criteria related to P3 assessment, likely due to the lack of standardized ERP methodology in this emerging field until recent guidelines became available (Clayson et al., Citation2019; Clayson & Miller, Citation2017; Keil et al., Citation2014).
Overall effects
Our meta-analyses revealed a small positive effect on P3 amplitude (increased amplitude) and P3 latency (decreased latency) following an acute bout of exercise. These findings were consistent with recent reviews, suggesting a positive aftereffect of exercise on P3 (Kao et al., Citation2020), particularly the amplitude measure of P3 (Meijer et al., Citation2020). Such effects were thought to reflect exercise-induced cognitive gains because they correspond with enhanced cognitive performance following exercise (Kao et al., Citation2020) and have been theorized to reflect increased allocation of attentional resources to and enhanced speed for evaluating behaviorally-relevant stimulus (Polich, Citation2007). Further, the modulation of P3 is theorized as a neuroelectric manifestation of altered arousal levels resulting from LC-NE activation (Nieuwenhuis et al., Citation2005). The increased amplitude and decreased latency of P3 following exercise may indicate that exercise, as a stressor, activates the LC-NE system in facilitating neural inhibition needed to perform cognitive tasks.
Experimental design
Experimental design was not a significant moderator on effect sizes of P3 amplitude and latency in response to acute exercise, suggesting the adequacy of subsequent examination and interpretation of effect sizes collapsed across experimental designs. Examination on each subgroup indicated positive amplitude effects using randomized between-subject and within-subject crossover comparison designs as well as positive latency effects using the within-subject crossover comparison design including only posttest, suggesting the reliability of these designs for capturing exercise-induced changes in P3 (Pontifex et al., Citation2019). Alternatively, such selective effects may simply result from the popularity of these designs as they account for more than 75% of the total effect sizes.
Task paradigm
Although P3 modulates as the function of task characteristics (Polich, Citation2007; Verleger, Citation2020), exercise-induced changes in P3 measures were not moderated by the predominant cognitive domain involved during the P3-eliciting tasks nor the probability of the P3-elicting stimulus. These suggest that changes in P3 following exercise reflect a general enhancement of neural inhibition underlying attentional and executive function processes regardless of the stimulus probability. However, these results should be interpreted with caution because most effect sizes came from inhibitory control tasks and P3-eliciting stimulus presented at and below 50%. Additionally, positive effect sizes for amplitude were significant only for inhibitory control and cognitive flexibility tasks as well as stimulus probability at and below 50%, potentially because these tasks and probability manipulations required greater capacity to resist distracting/conflicting and less predictable stimuli, allowing more reliable benefits from enhanced post-exercise neural inhibition. Further, the current meta-analysis only extracted data from the most dominating task condition within each cognitive domain, hence could not determine the role of the within-domain cognitive demand to the exercise-induced effects on P3.
Exercise characteristics
Exercise Type. The type of exercise was a significant moderator for the acute exercise effects on the amplitude but not latency measure of P3. Aerobic, resistance, and combined exercises have positive amplitude effects, suggesting their particular benefits to neural inhibition in support of effective allocation of attentional resources to task-relevant stimulus (Polich, Citation2007). However, the amplitude effects were less than small and not reliably different from zero for coordination exercise and HIIT, with HIIT further showing a significantly smaller effect than aerobic, resistance, and combined exercise.
For coordination exercise, there were only two effect sizes obtained from children with and without attention-deficit hyperactivity disorder (ADHD), respectively. In the context of acute exercise without long-term adaptation, the complex movement involved in coordination exercise may exceed the limited availability of cognitive resources, and this cognitive overload may prevent cognitive enhancement following the exercise cessation, especially in children with ADHD (Ludyga et al., Citation2017). The variability in the implementation of coordination exercise may also undermine its effectiveness for cognitive enhancement because individual difference in coordinative skills and the actual dose of exercise received (i.e. some individuals performed prescribed moves with more mistakes and therefore spent less time completing the prescribed moves) (Ludyga et al., Citation2017).
HIIT is characterized by exercise bouts at high intensities, interspersed by lower-intensity activity (Laursen & Jenkins, Citation2002). The alternation of low- and high-intensity bouts has been related to differential cardiovascular and cerebral hemodynamic responses compared with continuous aerobic exercise (Lyall et al., Citation2019; Ogoh et al., Citation2021; Tallon et al., Citation2019; Weaver et al., Citation2020). Specifically, HIIT resulted in pronounced parasympathetic withdrawal and sympathetic involvement, leading to a slower return of parasympathetic activity, as evidenced by lower high-frequency heart rate variability (HF-HRV), during the recovery period following exercise (Mourot et al., Citation2004). This reduced level of HF-HRV may reflect overly activated LC (Napadow et al., Citation2008), which is related to decreased P3 amplitude (Murphy et al., Citation2011).
Although exercise type did not moderate latency effects, positive effects were found selectively for aerobic exercise and HIIT. Consistent with previous studies (Kao et al., Citation2017; Kao et al., Citation2018), HIIT showed a numerically larger effect on decreasing latency than aerobic exercise, suggesting that HIIT might have a greater effect on increasing arousal and a facilitating effect on information processing speed. However, it should be noted that HIIT protocols employed in all of the included studies were prescribed at high intensities (Kao et al., Citation2018; Kao et al., Citation2017; Ligeza et al., Citation2018; Tsai et al., Citation2021; Xie et al., Citation2020), hence the largest latency effect observed for HIIT was confounded with exercise intensity.
Exercise intensity. As a more direct manipulation of arousal, exercise intensity moderated exercise-induced changes in P3 amplitude. Moderate-intensity exercise showed a positive amplitude effect that was marginally larger than low-intensity exercise as well as significantly larger than high-intensity exercise. Further, both low- and high-intensity exercise did not result in significant changes, confirming the previously found inverted-U and/or inverted-J shaped relationship between exercise intensity and P3 amplitude (Kamijo et al., Citation2004; Kao et al., Citation2017; Kao et al., Citation2018) as well as between P3 amplitude and LC-mediated arousal (Murphy et al., Citation2011). This suggests the particular effectiveness of moderate-intensity exercise for enhancing neural inhibition to task-irrelevant processing while making attentional resources available for task-relevant processing. Although this moderation effect of exercise intensity was not observed for P3 latency, only high-intensity exercise showed a significant positive effect, suggesting a necessity of exercise performed at higher intensities to induce reliable enhancements in information processing speed.
Exercise Duration. Consistent with the positive association between longer exercise and higher arousal level (Brisswalter et al., Citation2002), effect sizes of amplitude were moderated by the duration of exercise, with exercise lasting 21–30 min showing a larger effect than exercise lasting 11–20 min and ≤10 min. Further, examination on subgroup effects showed a non-significant and less than small positive effect for exercise lasting ≤10 min, a small positive effect for exercise lasting 11–20 min, and a moderate positive effect for exercise lasting 21–30 min, suggesting a minimum of 11-min exercise to reliably induce neuroelectric alteration responsible for attention allocation, in a linear dose–response fashion. For P3 latency, effect sizes were also moderated by exercise duration, with exercise lasting ≤10 min showing larger positive effects than 11–20 min exercise. However, this moderation effect was likely confounded with exercise intensity because high-intensity exercise was usually prescribed with shorter durations. Indeed, the effect sizes for exercise ≤ 10 min were obtained from studies prescribing high-intensity exercise (Jain et al., Citation2014; Kao et al., Citation2017). Taken together, although exercise duration appears to be an important moderator for exercise-induced effects on amplitude and latency, limited effect sizes (k = 2 for both amplitude and latency) for exercise lasting ≤10 min were obtained, warranting future research on modulation of P3 following a short exercise bout.
Exercise Volume. Despite the moderation effects of each exercise characteristic, the total exercise volume estimated by both the intensity and duration of exercise did not moderate the acute exercise effects on P3 amplitude and latency. Interestingly, although the amplitude effect was significant for all total exercise volume subgroups, exercise volume ≥201 MET showed a numerically larger effect compared with ≤100 and 100–200 MET volumes. This seemly dose-dependent pattern was also found in the latency effect for exercise volume ≥201 MET, despite the latency effect was only significant for exercise with 100–200 MET volume. Given the limited effect sizes for exercise volume ≥201 MET (k = 5 for amplitude, k = 2 for latency) and the preliminary attempt to estimate total exercise volume across studies using different type of exercise, more research examining the acute effect of large-volume exercise on P3 is needed.
Other Exercise Characteristics. The timing before P3 assessment and control activity were examined as moderators because they not only determined the calculation of effect sizes but also influenced arousal levels during the P3 assessment and contrasted with exercise interventions. These moderators did not moderate the effect sizes for amplitude and latency, suggesting the general effects of exercise on attentional allocation and neural processing speed regardless of post-exercise arousal levels and control activities contrasted with exercise. It is worth noting that amplitude effect sizes within these moderators showed significant positive effects for all subgroups except for exercise ended ≥31 min before P3 assessment and active control. This pattern of results corroborates the arousal account for the acute exercise effects on P3 amplitude. That is, acute exercise effects on P3 amplitude may became negligible when the exercise-induced elevation in arousal levels at the P3 assessment and contrasted with the control activity are minimized. Alternatively, this may suggest the persistence of exercise-induced increase in P3 amplitude for up to 30-min during the recovery period following exercise cessation. However, these selective null effects were derived from limited effect sizes (k = 2 for exercise ended ≥31 min before P3 assessment, k = 1 for using active control) and require more research to confirm.
Individual difference
Although the effect size of P3 latency did not differ between age groups, age was identified as a moderator for the acute exercise effect on P3 amplitude, with studies of samples of adults older than 60 years showing a greater increase in P3 amplitude following exercise compared to those conducted in younger age groups. Given that older adults exhibit smaller P3 amplitude (van Dinteren et al., Citation2014) and the importance of LC for maintaining cognitive function in aging brain (Mather & Harley, Citation2016), the moderate-to-large effect size for P3 amplitude observed in the oldest subgroup suggest the ability of acute exercise to temporarily mitigate the age-related decline in LC-mediated neuroinhibition function. While P3 amplitude and latency develop to their peaks during early adulthood (van Dinteren et al., Citation2014), the 19–35 years subgroup that was less likely to have age-related deficit in LC still showed small positive effects for both amplitude and latency measures with rather narrow confidence intervals, suggesting that exercise has temporal benefits to neuroinhibition even when it is most developed. Such acute exercise effects were the smallest and did not reach significance in children and adolescents aged at and below 18 years old during development likely because this age group typically exhibits no signs of LC pathology (Mather & Harley, Citation2016). It should be noted that no studies examining adults aged between 36–60 years old, indicating the need to investigate the acute exercise effects on P3 during middle-age adulthood.
Although P3 can distinguish individual differences in cognitive ability (Ludyga et al., Citation2017; Pontifex et al., Citation2013), sex (Hirayasu et al., Citation2000), and aerobic fitness (Kao et al., Citation2020; Raine et al., Citation2018), the acute effects of exercise on amplitude and latency measures were not moderated by these individual difference factors, suggesting the effectiveness of exercise for temporally enhancing neuroinhibition in facilitating attention allocation and processing speed in these different populations. Despite this, some subgroup effects related to these individual difference factors are worth noting. First, although positive effects on amplitude and latency were observed without a sex-related moderation effect, effect sizes for latency only reached significance in studies including only male participants. Given that males show greater changes in P3 latency during maturation (Hirayasu et al., Citation2000), the neural mechanisms underlying P3 latency may have greater modifiability in males and therefore is more amenable to acute exercise.
Second, the general effects regardless of cognitive ability were inconsistent with the common speculation that cognitively impaired individuals would show greater acute benefits of exercise to P3 measures because these populations exhibit smaller P3 amplitude and longer P3 latency (Ludyga et al., Citation2017; Pontifex et al., Citation2013; van Dinteren et al., Citation2014) and the importance of LC for maintaining cognitive function in impaired brain (Bast et al., Citation2018; Mather & Harley, Citation2016). The subgroup effects further contradicted with this speculation as the significant amplitude and latency effects were only observed in cognitively healthy individuals, despite P3 amplitude and latency follow a superior developmental trajectory in cognitively healthy individuals (Ludyga et al., Citation2017; Pontifex et al., Citation2013). These findings suggest that an acute physical stressor may be a less reliable approach to alter P3 measures in cognitively impaired individuals, specifically those experiencing ADHD (Hung et al., Citation2016; Ludyga et al., Citation2017; Pontifex et al., Citation2013) and mild cognitive impairment (Tsai et al., Citation2018), potentially because of the greater variability in brain responses for these subgroups or simply their impaired ability to perform the P3-eliciting tasks. Alternatively, the lack of reliable changes in P3 measures in these groups may result from the lack of exercise prescriptions designed for cognitive enhancement in these populations.
Third, effect sizes for amplitude were not significant only in low-fit individuals, implying that reliable acute exercise effects on P3 amplitude may require certain levels of aerobic fitness potentially due to its role in cerebrovascular regulation (Guiney et al., Citation2015), brain activation (Wong et al., Citation2015), and brain network connectivity (Talukdar et al., Citation2018) that enable superior neurocognitive function in response to acute physical stressor. This speculation was not supported by the selective latency effect in both high-fit and low-fit individuals, likely due to the less consistent relationship between aerobic fitness and P3 latency (Kao et al., Citation2020). However, it should be noted that about 40% of effect sizes for P3 measures were obtained from studies without reporting aerobic fitness because of different methods used to determine exercise intensity. Future research including aerobic fitness measures is encouraged to better understand the role of fitness in acute exercise effects on P3.
Potential mechanisms for acute exercise effects on P3
Although several candidate mechanisms of the acute exercise effects on cognitive performance have been proposed (McMorris, Citation2021; McMorris et al., Citation2016; Pontifex et al., Citation2019), arousal and its underlying neurophysiological mechanisms are most suitable for explaining the acute exercise effects on P3 activation, particularly its amplitude measure (Nieuwenhuis et al., Citation2005). Deriving from the inverted-U shaped relationship between arousal and performance (Yerkes & Dodson, Citation1908), exercise is thought to serve as a physical stressor to increase arousal to a level that is optimal for performance, especially when comparing with a non-exercise sedentary control activity requiring minimum physical exertion and thus resulting in a sub-optimal arousal level for performance. Converging evidence from animal and human studies has further identified LC-NE system as the potential neurophysiological mechanism underlying the exercise-related changes in peripheral and central arousal (McMorris, Citation2021; McMorris et al., Citation2016). Given the essential role of LC-NE system to cerebral arousal that supports attention and executive function (Nieuwenhuis et al., Citation2005), it has been speculated that exercise stimulates the activation of LC and NE synthesis (McMorris et al., Citation2016), in turn the release of NE to exert modulatory effect on projected brain areas such as frontal and temporal brain regions (Nieuwenhuis et al., Citation2005), and finally the neurophysiological manifestation such as P3 activation associated with these regions (Polich, Citation2007).
Empirically testing these arousal-mechanisms in human is challenging because it requires invasive methods concurrently with collection of neurophysiological signals after exercise. To date, only one study investigated the acute exercise effects on P3 while concurrently monitoring pupillometry as an indirect measure of LC activation. The results did not show exercise-induced changes in tonic and phasic LC action nor their associations with increased P3 amplitude following exercise (McGowan et al., Citation2019). However, another study combining pupillometry and functional magnetic resonance imaging (fMRI) found that a short bout of interval handgrip exercise decreased tonic LC activation but increased phasic LC activation in response to a salient (i.e. less probable) target stimulus during an oddball task (Mather et al., Citation2020). The oddball paradigm is a classic paradigm that robustly elicits larger P3 amplitude in response to a behaviorally relevant target stimuli (Polich, Citation2007) and this target-elicited P3 amplitude was shown to be altered by a single bout of exercise (Pontifex et al., Citation2015). The similarity in the patterns of moderation in P3 and LC activation in response to the manipulation of stimulus probability as well as the acute exercise intervention suggest a potential association of LC-NE system with P3 in response to acute physical stressor. Given the discrepant findings derived from very limited research, there is a clear need to investigate the mechanistic role of LC-NE system in the acute exercise effect on P3, which may further help elucidate the neural mechanisms of exercise-induced changes in behavioral cognitive performance.
Manipulating exercise characteristics to target the LC-NE mechanism
Although the mechanistic role of LC-NE system in the acute exercise effects on P3 requires more research to establish, the LC-NE system is well-suitable for explaining the moderation effects of exercise characteristics on the amplitude measure of P3. Given that non-exercise studies have demonstrated an inverted-U relationship between P3 amplitude and tonic LC activation (LoTemplio et al., Citation2021; Murphy et al., Citation2011), the exercise-induced increases in P3 amplitude likely reflects a superior tonic brain functioning state characterized by optimal activation levels of the arousal system (Nieuwenhuis et al., Citation2005). This speculation corresponds with the inverted-U/inverted-J relationship between P3 amplitude and exercise intensity observed in the current meta-analysis. These findings suggest that exercise can be a vehicle with variations, if prescribed properly, to induce an adequate level of stress response and achieve the optimal activation level of the arousal system, leading to a superior brain functioning state that prepares effective neural inhibition in facilitating allocation of attentional resources to implement cognitive processes.
The moderation effect of exercise duration did not show an inverted-U shaped relationship between exercise duration and P3 amplitude, as longer exercise showed the largest amplitude effect. Similarly, larger volume exercise showed a moderate amplitude effect that was numerically larger than smaller exercise volumes, despite the lack of moderation effect of total exercise volume on P3 amplitude. Unlike exercise intensity that was defined using a relative manner standardized to individual maximum capacity (i.e. HRmax or 1RM), exercise duration and volume were defined by the absolute values based on the prevalent use in the existing literature. It is possible that the inverted-U relationship between duration/volume and P3 amplitude might show if long-duration or large-volume exercise (i.e. >60 min) were included in the moderation analysis. However, the duration and volume of exercise prescribed in the existing literature were likely intended to avoid prolonged exercise with large volumes for feasibility (i.e. difficult for participants to complete the already long cognitive and EEG testing and a long exercise) and practicality reasons (i.e. impractical to use a 60-min exercise bout in schools to improve students’ attention).
Examining the association of post-exercise arousal level with P3 amplitude may provide an alternative approach to manipulate the exercise-induced changes in P3 amplitude through targeting the LC-NE system. Given that exercise-induced increases in arousal, so does its underlying LC-NE activation, subsides gradually over time during the recovery period following exercise cessation, it is speculated that the LC-NE mediated P3 amplitude would change as the function of the time delay between exercise cessation and P3 assessment. Although our meta-analysis did not support this speculation, as the exercise timing before P3 assessment was not a significant moderator, the amplitude effects were significant only when exercise was completed within 30-min before the P3 assessment. In contrast, the amplitude effect assessed after more than a 30-min delay following exercise became non-significant likely because the exercise-induced increases in arousal and its underlying LC-NE activation had begun to normalize. However, it should be noted that 8 of the effect sizes were obtained from studies without reporting the timing of P3 assessment in relative to exercise cessation. Systematically manipulating exercise timing before P3 assessment and exercise characteristics such as intensity, duration, and volume that modulate arousal and its underlying LC-NE system is needed to not only identify the best exercise prescription for transiently altering P3 amplitude but also indirectly test LC-NE system as the mechanism of acute exercise effects on P3 amplitude.
Although the current meta-analysis also showed a positive (i.e. decreased latency) P3 latency effect following exercise, this effect was quantitatively smaller than that of amplitude and was only moderated by the duration of exercise. The overall patters of subgroup effects within each exercise-related moderator also did not indicate a pattern of inverted-U relationship between exercise characteristic and P3 latency. This appears consistent with the evidence that alteration in P3 latency was not a good neuroelectric manifestation of LC-NE activation (Nieuwenhuis et al., Citation2005) and thus less responsive to acute physical stressor induced by exercise (Kao et al., Citation2020). Alternatively, methodological considerations related to latency quantification (Nieuwenhuis et al., Citation2005) and exclusion of latency from reporting may bias the current findings in latency effects. Before more empirical evidence becomes available, the current meta-analysis indicated that P3 latency may be a less sensitive index to reflect the modulation of LC-NE mediated cerebral arousal in response to manipulation of exercise characteristics.
Applications for acute exercise effects on P3
With the need to better understand the relationship between physical activity and cognitive health (Physical Activity Guidelines Advisory Committee, Citation2018), P3 has been increasingly used to determine the neurocognitive benefits of acute exercise (Kao et al., Citation2020) because of its association with a host of health and developmental outcomes (Polich, Citation2007). This meta-analysis corroborated the potential utility of P3 to evaluate acute exercise effects on brain functioning and demonstrated the manipulability of such neurocognitive effects through a simple behavioral approach with variation in exercise parameters, informing the potential of exercise as an effective, inexpensive, and healthy strategy for temporarily altering brain function that is important for cognitive performance. Given that increased P3 amplitude and/or decreased P3 latency have frequently been observed along with enhanced cognitive performance following acute bouts of exercise (Kao et al., Citation2020), the current findings also provide evidence to guide the development toward optimal exercise intervention to maximize exercise-induced neurocognitive benefits. For instance, the inverted-U relationship between P3 amplitude and exercise intensity suggests that exercise-induced changes in brain function in facilitating attention allocation can be maximized by prescribing exercise at a moderate-intensity. Such a brain functioning state can also be achieved by engaging a single bout of exercise using aerobic, resistance, and combined forms as well as lasting >20 min. Additionally, acute exercise effects on P3 latency can be maximized by prescribing exercise lasting ≤10 min. Although the short-duration exercise is practically attractive for its time-efficiency for temporally enhancing the speed of information processing, it should be noted that the larger latency effect associated with exercise lasting ≤10 min was confounded with a higher exercise intensity. Thus, a high intensity may be needed for short exercise bout to maximize the latency effect.
To expand the potential application of acute exercise for enhancing brain function, there are several future directions to be considered. First, as repeatedly iterated, the current literature is lacking investigation into long-duration exercise with large volume, limiting the ability to depict the speculated dose–response relationship more accurately between acute exercise and P3. Relatedly, although the literature has generally assumed that the acute exercise benefits to cognition lasts about 1 hour following exercise cessation, the persistence of the exercise-induced effects on P3 remains to be empirically tested. Future research systematically comparing exercise protocols prescribed with different durations/volumes and placed at different timings in relative to P3 assessments is needed to inform the best practice in exercise-based intervention for transiently enhancing subsequent task-related brain functioning.
Second, although our analysis did not reveal any moderation effect related to individual difference factors, exercise appeared to have less reliable effects on P3 measures in developing and cognitively impaired individuals. A recent review has proposed an interceptive model, which contends that exercise-induced activation of the LC-NE system also interacts with psychological factors such as motivation, perceived effort costs and perceived availability of resources that could vary across individuals (McMorris, Citation2021). Future research accommodating for developing and cognitively impaired individuals while considering the psychological outcomes is needed to better understand the potential of exercise-based strategy for not only maximizing brain functioning in typically developing individuals but also counteracting neurocognitive deficits.
Third, although the current meta-analysis provided evidence that exercise is an effective approach for transiently enhancing brain function as manifested by increased amplitude and decreased latency of P3, whether the effectiveness of such an exercise-based strategy is comparable with other alternative options remains largely unknown. To the best of our understanding, no evidence exists directly comparing the acute effects of exercise with alternative interventions on P3. Given that arousal and its underlying LC-NE system play the potentially mechanistic role in the exercise-induced changes in P3, approaches that alter arousal levels may be of particular interest for effectiveness comparison. For example, brain stimulation through transcranial direct current stimulation (tDCS) has been shown to transiently affect P3 (Nikolin et al., Citation2018). Biobehavioral approaches such as neurofeedback (Deiber et al., Citation2021) and mindfulness training (Norris et al., Citation2018; Wolff & Beste, Citation2020) have also been shown to have acute effects on P3. Empirically comparing different arousal-altering interventions can further elucidate the unique contribution of exercise-induced physical exertion resulting from muscle contraction and energy expenditure to P3 modulations. This research line will provide evidence to inform the decision-making process of adapting an exercise-based intervention and translating it to the real-life settings.
Lastly, research linking exercise-induced changes in P3 with behavioral cognitive performance is needed. Increased P3 amplitude and decreased latency have been repeatedly found to correlate with better behavioral cognitive performance (Polich, Citation2007) as well as a host of psychological (Cahill & Polich, Citation1992), developmental (Polich & Martin, Citation1992), and health outcomes (Petersen et al., Citation2018). Similar positive associations have been found in the context of acute exercise and P3, with studies showing increased P3 amplitude and/or decreased P3 latency also showing improved behavioral performance during the P3-eliciting tasks (Kao et al., Citation2020; Kao et al., Citation2022; McGowan et al., Citation2019). However, some other studies reported no correlation between P3 measures and cognitive performance (Tsai et al., Citation2018) following acute exercise and the literature to date has limited understanding of the associations between exercise-induced changes in P3 and task performance at the individual study and subject levels. Further, task paradigms employed to elicit P3 could also affect the relationship of the amplitude and latency measures of P3 with task performance (Verleger, Citation2020). Future research should be encouraged to report these correlations to allow quantitative synthesis on the association between P3 and behavioral cognitive performance in response to exercise.
Strengths and limitations
The current meta-analysis provided a quantitative evaluation of the overall exercise-induced effects on P3 and potential moderators. A multilevel analysis was employed to handle the dependency of effect sizes obtained from studies having multiple effects sizes (Cheung, Citation2019; Gucciardi et al., Citation2022). Further, a data-driven approach was used to extract the largest centro-parietally-centered P3 observed at the midline electrodes at an individual study level from each of the included studies to ensure the current findings were less likely to be confounded with the frontally-centered P3a component. Despite these strengths, limitations inherent to the quality of analyzed studies should be considered. First, there was evidence of potential publication bias for the exercise-induced effects on P3 amplitude, likely resulting from a few small studies having large effects (i.e. Won et al., Citation2017) and contributing to overestimated pooled effect size. Similar to previous meta-analyses on behavioral cognitive outcomes following exercise (Chang et al., Citation2012; Ludyga et al., Citation2016; Moreau & Chou, Citation2019), heterogeneity among studies was moderate for P3 amplitude. This may also reflect methodological variabilities in P3 assessments, as only one study used blinded assessors (Pontifex et al., Citation2021) and half studies reported sufficient information about EEG data reduction, which moderated amplitude effect sizes, suggesting the need for improvements following technical guidelines (Keil et al., Citation2014). Particularly relevant is the reliability of P3 measures because of the popular use of repeated measures needed to evaluate the difference between exercise conditions, time points, and/or the condition by time interactions. In addition to reporting information about test-retest reliability and signal-to-noise ratio (i.e. the minimum accepted trials to construct grand average for extracting P3 amplitude and latency), future research should be encouraged to include sufficiently powered sample sizes to account for data loss due to unacceptable outcome reliability (Bailey et al., Citation2021). Although no publication bias for P3 latency effect was identified and the heterogeneity among studies for P3 latency was low-to-moderate, the effect size for P3 latency was larger for studies using between-subject design without a concealed allocation. Thus, knowledge of future assignment to intervention available to experimenters should be minimized in future studies using between-subject designs to adequately evaluate acute exercise effects on P3 latency. Second, moderator analyses were performed regardless of the number of effect sizes available for each subgroup to best represent the literature. Consequently, some subgroups had limited effect sizes, resulting in low power, less accurate estimates, and the inability to explore interactions between moderators. Relatedly, although the moderator analyses were conducted based on the common practice in categorizing exercise characteristics, these characteristics were oftentimes confounded with each other (e.g. intensity negatively correlates with duration). The current study attempted to address this limitation by converting these characteristics to generate an estimated measure of total exercise volume. However, future research systematically manipulating a single exercise characteristic to isolate its effect on P3 is still needed to best understand the influence of each exercise characteristic on exercise-induced effects on P3. Lastly, the current study focused on the exercise-induced modulations of P3 as outcome measures without considering the concurrent behavioral performance during the P3-eliciting tasks, limiting the interpretability of exercise-induced changes in P3 measures. Systematic research on the relationship between exercise-induced changes in P3 and its functional significance to behavioral and clinical outcomes is warranted.
Conclusion
The advance in human brain science in the past few decades has stimulated the utilization of neuroelectric measures such as ERP to evaluate the consequence of physical activity engagement in the field of exercise psychology. The findings in the current study showed that an acute bout of exercise affects P3, a marker of functional neural inhibition underlying attentional and executive function processes, by increasing its amplitude and decreasing its latency, suggesting increased attentional resources allocation and information processing speed. Further, the amplitude effect may be enlarged through manipulating the type, intensity, and duration of exercise as well as when targeting older adults, whereas the latency effect depends on the duration of exercise. Given the increasing interest in the feasibility and maximization of exercise-based strategy for cognitive enhancement, the current findings offered a novel evidence from meta-analytic perspective that acute exercise can alter neuroelectric activities in support of cognitive functions that are essential for goal-directed behavior in many real-life settings.
Acknowledgements
We thank all authors of the included studies for providing data.
Disclosure statement
No potential conflict of interest was reported by the author(s).
Data availability statement
The data that support the findings of this study are available upon request from the corresponding author [YKC].
Additional information
Funding
Notes
1 Although other neuroscience tools such as magnetoencephalography (MEG) can also be used to study brain activity that is similar to P3-ERP, MEG requires large facility, is costly, and has rarely been used in the field of sport and exercise psychology. This study intended to focus on EEG-derived P3-ERP because EEG has become increasingly accessible for research and clinical uses in the field of sport and exercise psychology. It is also the authors’ understanding that the existing literature on acute exercise and cognition primarily used EEG to measure P3-ERP.
2 P3 is not the only ERP component reflecting cognitive processes during the stimulus evaluation. For example, P1 and N2 are other earlier components that index perceptual attention and conflict monitoring. Variation of P3 component such as P3a that can be elicited by an infrequent distractor during the 3-stimulus oddball paradigm also exists. In contrast with P3 or P3b, P3a peaks earlier at frontally-centered electrodes and indexes attentional orienting. Despite these different ERP components exist, they have been less studied in relation to acute exercise and findings from the limited studies are mixed.
3 Percent of maximum heart rate reported or converted based on exercise and maximum (measured or estimated) heart rate were the first source to determine intensity. When heart rate measures showed disagreement with self-reported measures (e.g., moderate-intensity based on a mean RPE = 12 but high-intensity based on an average of 80% heart rate maximum), a higher intensity was always used. When a range of intensity (e.g., 65–75% heart rate maximum) was reported, the mean of the range was used to reflect the intensity (e.g., 70% heart rate maximum). For resistance exercise, intensity was determined based on percent of one-repetition maximum.
4 Aerobic fitness was measured by actual and estimated maximum oxygen consumptions (ml/kg/min), which were in turn converted into percentile scores using a sex- and age-adjusted norm (Shvartz & Reibold, Citation1990).
References
- Aly, M., & Kojima, H. (2020). Acute moderate-intensity exercise generally enhances neural resources related to perceptual and cognitive processes: A randomized controlled ERP study. Mental Health and Physical Activity, 19, 100363. https://doi.org/10.1016/j.mhpa.2020.100363
- American College of Sports Medicine. (2014). ACSM’s guidelines of exercise testing and prescription (9th ed.). Lippincott Williams & Wilkins.
- Bae, S., & Masaki, H. (2019). Effects of acute aerobic exercise on cognitive flexibility required during task-switching paradigm. Frontiers in Human Neuroscience, 13, 260. https://doi.org/10.3389/fnhum.2019.00260
- Bailey, B. W., Muir, A. M., Bartholomew, C. L., Christensen, W. F., Carbine, K. A., Marsh, H., LaCouture, H., McCutcheon, C., & Larson, M. (2021). The impact of exercise intensity on neurophysiological indices of food-related inhibitory control and cognitive control: A randomized crossover event-related potential (ERP) study. NeuroImage, 237, 118162. https://doi.org/10.1016/j.neuroimage.2021.118162
- Bast, N., Poustka, L., & Freitag, C. (2018). The locus coeruleus-norepinephrine system as pacemaker of attention – a developmental mechanism of derailed attentional function in autism spectrum disorder. European Journal of Neuroscience, 47(2), 115–125. https://doi.org/10.1111/ejn.13795
- Brisswalter, J., Collardeau, M., & René, A. (2002). Effects of acute physical exercise characteristics on cognitive performance. Sports Medicine, 32(9), 555–566. https://doi.org/10.2165/00007256-200232090-00002
- Brydges, C. R., Fox, A. M., Reid, C. L., & Anderson, M. (2014). Predictive validity of the N2 and P3 ERP components to executive functioning in children: A latent-variable analysis. Frontiers in Human Neuroscience, 8, 80. https://doi.org/10.3389/fnhum.2014.00080
- Cahill, J., & Polich, J. (1992). P300, probability, and introverted/extroverted personality types. Biological Psychology, 33(1), 23–35. https://doi.org/10.1016/0301-0511(92)90003-D
- Carbine, K., Anderson, J., Larson, M., LeCheminant, J., & Bailey, B. (2020). The relationship between exercise intensity and neurophysiological responses to food stimuli in women: A randomized crossover event-related potential (ERP) study. International Journal of Psychophysiology, 158, 349–361. https://doi.org/10.1016/j.ijpsycho.2020.10.011
- Carrillo-de-la-Peña, M., & Cadaveira, F. (2000). The effect of motivational instructions on P300 amplitude. Neurophysiologie Clinique/Clinical Neurophysiology, 30(4), 232–239. https://doi.org/10.1016/S0987-7053(00)00220-3
- Chacko, S. C., Quinzi, F., De Fano, A., Bianco, V., Mussini, E., Berchicci, M., Perri, R. L., & Di Russo, F. (2020). A single bout of vigorous-intensity aerobic exercise affects reactive, but not proactive cognitive brain functions. International Journal of Psychophysiology, 147, 233–243. https://doi.org/10.1016/j.ijpsycho.2019.12.003
- Chang, Y. K. (2016). Chapter 5 – acute exercise and event-related potential: Current status and future prospects. In T. McMorris (Ed.), Exercise-cognition interaction (pp. 105–130). Academic Press.
- Chang, Y. K., Alderman, B. L., Chu, C. H., Wang, C. C., Song, T. F., & Chen, F. T. (2017). Acute exercise has a general facilitative effect on cognitive function: A combined ERP temporal dynamics and BDNF study. Psychophysiology, 54(2), 289–300. https://doi.org/10.1111/psyp.12784
- Chang, Y.-K., Erickson, K. I., Stamatakis, E., & Hung, T.-M. (2019). How the 2018 US physical activity guidelines are a call to promote and better understand acute physical activity for cognitive function gains. Sports Medicine, 49, 1625–1627. https://doi.org/10.1007/s40279-019-01190-x
- Chang, Y. K., & Etnier, J. L. (2015). Acute exercise and cognitive function: Emerging research issues. Journal of Sport and Health Science, 4(1), 1–3. https://doi.org/10.1016/j.jshs.2014.12.001
- Chang, Y. K., Labban, J. D., Gapin, J. I., & Etnier, J. L. (2012). The effects of acute exercise on cognitive performance: A meta-analysis. Brain Research, 1453, 87–101. https://doi.org/10.1016/j.brainres.2012.02.068
- Chang, Y.-K., Pesce, C., Chiang, Y.-T., Kuo, C.-Y., & Fong, D.-Y. (2015). Antecedent acute cycling exercise affects attention control: An ERP study using attention network test. Frontiers in Human Neuroscience, 9, 156. https://doi.org/10.3389/fnhum.2015.00156
- Chen, F. T., Etnier, J. L., Chan, K. H., Chiu, P. K., Hung, T. M., & Chang, Y. K. (2020). Effects of exercise training interventions on executive function in older adults: A systematic review and meta-analysis. Sports Medicine, 50(8), 1451–1467. https://doi.org/10.1007/s40279-020-01292-x
- Cheung, M. (2019). A guide to conducting a meta-analysis with non-independent effect sizes. Neuropsychology Review, 29(4), 387–396. https://doi.org/10.1007/s11065-019-09415-6
- Chu, C. H., Alderman, B. L., Wei, G. X., & Chang, Y. K. (2015). Effects of acute aerobic exercise on motor response inhibition: An ERP study using the stop-signal task. Journal of Sport and Health Science, 4(1), 73–81. https://doi.org/10.1016/j.jshs.2014.12.002
- Chu, C.-H., Kramer, A. F., Song, T.-F., Wu, C.-H., Hung, T.-M., & Chang, Y.-K. (2017). Acute exercise and neurocognitive development in preadolescents and young adults: An ERP study. Neural Plasticity, 2017, 1–13. https://doi.org/10.1155/2017/2631909
- Clayson, P., & Miller, G. (2017). Psychometric considerations in the measurement of event-related brain potentials: Guidelines for measurement and reporting. International Journal of Psychophysiology, 111, 57–67. https://doi.org/10.1016/j.ijpsycho.2016.09.005
- Clayson, P. E., Carbine, K. A., Baldwin, S. A., & Larson, M. J. (2019). Methodological reporting behavior, sample sizes, and statistical power in studies of event-related potentials: Barriers to reproducibility and replicability. Psychophysiology, 56(11), e13437. https://doi.org/10.1111/psyp.13437
- Craft, L., & Landers, D. (1998). The effect of exercise on clinical depression and depression resulting from mental illness: A meta-analysis. Journal of Sport and Exercise Psychology, 20(4), 339–357. https://doi.org/10.1123/jsep.20.4.339
- Critchley, H., Corfield, D., Chandler, M., Mathias, C., & Dolan, R. (2000). Cerebral correlates of autonomic cardiovascular arousal: A functional neuroimaging investigation in humans. The Journal of Physiology, 523(1), 259–270. https://doi.org/10.1111/j.1469-7793.2000.t01-1-00259.x
- Deiber, M.-P., Ammann, C., Hasler, R., Colin, J., Perroud, N., & Ros, T. (2021). Electrophysiological correlates of improved executive function following EEG neurofeedback in adult attention deficit hyperactivity disorder. Clinical Neurophysiology, 132(8), 1937–1946. https://doi.org/10.1016/j.clinph.2021.05.017
- Dimitrova, J., Hogan, M., Khader, P., O’Hora, D., Kilmartin, L., Walsh, J. C., Roche, R., & Anderson-Hanley, C. (2017). Comparing the effects of an acute bout of physical exercise with an acute bout of interactive mental and physical exercise on electrophysiology and executive functioning in younger and older adults. Aging Clinical and Experimental Research, 29(5), 959–967. https://doi.org/10.1007/s40520-016-0683-6
- Donchin, E., & Coles, M. G. H. (1988). Is the P300 component a manifestation of context updating? Behavioral and Brain Sciences, 11(3), 357–374. https://doi.org/10.1017/S0140525X00058027
- Drollette, E. S., Scudder, M. R., Raine, L. B., Moore, R. D., Saliba, B. J., Pontifex, M. B., & Hillman, C. H. (2014). Acute exercise facilitates brain function and cognition in children who need it most: An ERP study of individual differences in inhibitory control capacity. Developmental Cognitive Neuroscience, 7, 53–64. https://doi.org/10.1016/j.dcn.2013.11.001
- Duncan, C. C., Barry, R. J., Connolly, J. F., Fischer, C., Michie, P. T., Naatanen, R., Polich, J., Reinvang, I., & Van Petten, C. (2009). Event-related potentials in clinical research: Guidelines for eliciting, recording, and quantifying mismatch negativity, P300, and N400. Clinical Neurophysiology, 120(11), 1883–1908. https://doi.org/10.1016/j.clinph.2009.07.045
- Du Rietz, E., Barker, A., Michelini, G., Rommel, A. S., Vainieri, I., Asherson, P., & Kuntsi, J. (2019). Beneficial effects of acute high-intensity exercise on electrophysiological indices of attention processes in young adult men. Behavioural Brain Research, 359, 474–484. https://doi.org/10.1016/j.bbr.2018.11.024
- Duval, S., & Tweedie, R. (2000). Trim and fill: A simple funnel-plot-based method of testing and adjusting for publication bias in meta-analysis. Biometrics, 56(2), 455–463. https://doi.org/10.1111/j.0006-341X.2000.00455.x
- Duzova, H., Özişik, H. I., Polat, A., Emre, M. H., & Gullu, E. (2005). Correlations between event-related potential components and nitric oxide in maximal anaerobic exercise among sportsmen trained at various levels. International Journal of Neuroscience, 115(10), 1353–1373. https://doi.org/10.1080/00207450590956387
- Egger, M., Smith, G., Schneider, M., & Minder, C. (1997). Bias in meta-analysis detected by a simple, graphical test. BMJ, 315(7109), 629–634. https://doi.org/10.1136/bmj.315.7109.629
- Erickson, K., Hillman, C., Stillman, C., Ballard, R., Bloodgood, B., Conroy, D., Macko, R., Marquez, D. D. X., Petruzzello, S. J., & Powell, K. (2019). Physical activity, cognition, and brain outcomes: A review of the 2018 physical activity guidelines. Medicine & Science in Sports & Exercise, 51(6), 1242–1251. https://doi.org/10.1249/MSS.0000000000001936
- Etnier, J. L., & Chang, Y. K. (2009). The effect of physical activity on executive function: A brief commentary on definitions, measurement issues, and the current state of the literature. Journal of Sport and Exercise Psychology, 31(4), 469–483. https://doi.org/10.1123/jsep.31.4.469
- Fabiani, M., Gratton, G., & Federmeier, K. (2007). Event-related brain potentials: Methods, theory, and applications. In J. T. Cacioppo, L. G. Tassinary, & G. G. Berntson (Eds.), Handbook of psychophysiology (3rd ed., pp. 85–119). Cambridge University Press. https://doi.org/10.1017/CBO9780511546396.004
- Fearnbach, S., Silvert, L., Keller, K., Genin, P. M., Morio, B., Pereira, B., Duclos, M., Boirie, Y., & Thivel, D. (2016). Reduced neural response to food cues following exercise is accompanied by decreased energy intake in obese adolescents. International Journal of Obesity, 40(1), 77–83. https://doi.org/10.1038/ijo.2015.215
- Fearnbach, S., Silvert, L., Pereira, B., Boirie, Y., Duclos, M., Keller, K., & Thivel, D. (2017). Reduced neural responses to food cues might contribute to the anorexigenic effect of acute exercise observed in obese but not lean adolescents. Nutrition Research, 44, 76–84. https://doi.org/10.1016/j.nutres.2017.06.006
- Gowsi, K., Jr., Sharma, V. K., Pal, G. K., & Aaramban, P. (2016). Effects of moderate and high-intensity exercise on P300 latency and reaction time in athletes and nonathletes – an interim analysis. Biomedicine, 36(4), 96–101.
- Grego, F., Vallier, J.-M., Collardeau, M., Bermon, S., Ferrari, P., Candito, M., Bayer, P., Magnié, M.-N., & Brisswalter, J. (2004). Effects of long duration exercise on cognitive function, blood glucose, and counterregulatory hormones in Male cyclists. Neuroscience Letters, 364(2), 76–80. https://doi.org/10.1016/j.neulet.2004.03.085
- Grillner, S., Ip, N., Koch, C., Koroshetz, W., Okano, H., Polachek, M., Poo, M.-M., & Sejnowski, T. (2016). Worldwide initiatives to advance brain research. Nature Neuroscience, 19(9), 1118–1122. https://doi.org/10.1038/nn.4371.
- Gucciardi, D. F., Lines, R. L., & Ntoumanis, N. (2022). Handling effect size dependency in meta-analysis. International Review of Sport and Exercise Psychology, 15(1), 152–178. https://doi.org/10.1080/1750984X.2021.1946835
- Guiney, H., Lucas, S. J., Cotter, J. D., & Machado, L. (2015). Evidence cerebral blood-flow regulation mediates exercise–cognition links in healthy young adults. Neuropsychology, 29(1), 1–9. https://doi.org/10.1037/neu0000124
- Higgins, J., Thompson, S., Deeks, J., & Altman, D. (2003). Measuring inconsistency in meta-analyses. BMJ, 327(7414), 557–560. https://doi.org/10.1136/bmj.327.7414.557
- Hillman, C. H., Kamijo, K., & Pontifex, M. B. (2012). The relation of ERP indices of exercise to brain health and cognition. In H. Boecker, C. Hillman, L. Scheef, and H. Strüder (Eds.), Functional neuroimaging in exercise and sport sciences (pp. 419–446). Springer. https://doi.org/10.1007/978-1-4614-3293-7_18
- Hillman, C. H., Pontifex, M. B., Raine, L. B., Castelli, D. M., Hall, E. E., & Kramer, A. F. (2009). The effect of acute treadmill walking on cognitive control and academic achievement in preadolescent children. Neuroscience, 159(3), 1044–1054. https://doi.org/10.1016/j.neuroscience.2009.01.057
- Hillman, C. H., Snook, E. M., & Jerome, G. J. (2003). Acute cardiovascular exercise and executive control function. International Journal of Psychophysiology, 48(3), 307–314. https://doi.org/10.1016/S0167-8760(03)00080-1
- Hirayasu, Y., Samura, M., Ohta, H., & Ogura, C. (2000). Sex effects on rate of change of P300 latency with age. Clinical Neurophysiology, 111(2), 187–194. https://doi.org/10.1016/S1388-2457(99)00233-3
- Hsieh, S. S., Huang, C. J., Chang, Y. K., & Hung, T. M. (2018). Acute exercise facilitates the N450 inhibition marker and P3 attention marker during Stroop test in young and older adults. Journal of Clinical Medicine, 7(11), 391–409. https://doi.org/10.3390/jcm7110391.
- Hung, C. L., Huang, C. J., Tsai, Y. J., Chang, Y. K., & Hung, T. M. (2016). Neuroelectric and behavioral effects of acute exercise on task switching in children with attention-deficit/hyperactivity disorder. Frontiers in Psychology, 7, 1589. https://doi.org/10.3389/fpsyg.2016.01589.
- Jain, P., Jain, P., Jain, A. K., & Babbar, R. (2014). Influence of affective changes on behavioral and cognitive performances after acute bout of exhaustive exercise. Journal of Psychophysiology, 28(1), 1–10. https://doi.org/10.1027/0269-8803/a000105
- Kamijo, K., Hayashi, Y., Sakai, T., Yahiro, T., Tanaka, K., & Nishihira, Y. (2009). Acute effects of aerobic exercise on cognitive function in older adults. The Journals of Gerontology Series B: Psychological Sciences and Social Sciences, 64B(3), 356–363. https://doi.org/10.1093/geronb/gbp030
- Kamijo, K., Nishihira, Y., Hatta, A., Kaneda, T., Wasaka, T., Kida, T., & Kuroiwa, K. (2004). Differential influences of exercise intensity on information processing in the central nervous system. European Journal of Applied Physiology, 92(3), 305–311. https://doi.org/10.1007/s00421-004-1097-2
- Kamijo, K., Nishihira, Y., Higashiura, T., & Kuroiwa, K. (2007). The interactive effect of exercise intensity and task difficulty on human cognitive processing. International Journal of Psychophysiology, 65(2), 114–121. https://doi.org/10.1016/j.ijpsycho.2007.04.001
- Kao, S.-C., Baumgartner, N., Nagy, C., Fu, H.-L., Yang, C.-T., & Wang, C.-H. (2022). Acute effects of aerobic exercise on conflict suppression, response inhibition, and processing efficiency underlying inhibitory control processes: AnERPandSFTstudy. Psychophysiology, 59, https://doi.org/10.1111/psyp.14032
- Kao, S.-C., Cadenas-Sanchez, C., Shigeta, T. T., Walk, A. M., Chang, Y.-K., Pontifex, M. B., & Hillman, C. H. (2020). A systematic review of physical activity and cardiorespiratory fitness on P3b. Psychophysiology, 57(7), e13425. https://doi.org/10.1111/psyp.13425
- Kao, S. C., Drollette, E. S., Ritondale, J. P., Khan, N., & Hillman, C. H. (2018). The acute effects of high-intensity interval training and moderate-intensity continuous exercise on declarative memory and inhibitory control. Psychology of Sport and Exercise, 38, 90–99. https://doi.org/10.1016/j.psychsport.2018.05.011
- Kao, S. C., Westfall, D. R., Soneson, J., Gurd, B., & Hillman, C. H. (2017). Comparison of the acute effects of high-intensity interval training and continuous aerobic walking on inhibitory control. Psychophysiology, 54(9), 1335–1345. https://doi.org/10.1111/psyp.12889
- Keil, A., Debener, S., Gratton, G., Junghöfer, M., Kappenman, E., Luck, S. J., Luu, P., Miller, G. A., & Yee, C. (2014). Committee report: Publication guidelines and recommendations for studies using electroencephalography and magnetoencephalography. Psychophysiology, 51(1), 1–21. https://doi.org/10.1111/psyp.12147
- Kenney, L., Wilmore, J., & Costill, D. (2015). Physiology of sport and exercise. Human Kinetics.
- Keye, S., Walk, A., Cannavale, C., Iwinski, S., McLoughlin, G., Steinberg, L., & Khan, N. (2021). Six-minute walking test performance relates to neurocognitive abilities in preschoolers. Journal of Clinical Medicine, 10(4), 584. https://doi.org/10.3390/jcm10040584
- Kim, H. (2014). Involvement of the dorsal and ventral attention networks in oddball stimulus processing: A meta-analysis. Human Brain Mapping, 35(5), 2265–2284. https://doi.org/10.1002/hbm.22326
- Kliszczewicz, B., Esco, M., Quindry, J., Blessing, D., Oliver, G., Taylor, K., & Price, B. (2016). Autonomic responses to an acute bout of high-intensity body weight resistance exercise vs. treadmill running. Journal of Strength and Conditioning Research, 30(4), 1050–1058. https://doi.org/10.1519/JSC.0000000000001173
- Kok, A. (1997). Event-related-potential (ERP) reflections of mental resource̊s: A review and synthesis. Biological Psychology, 45(1-3), 19–56. https://doi.org/10.1016/S0301-0511(96)05221-0
- Kok, A. (2001). On the utility of P3 amplitude as a measure of processing capacity. Psychophysiology, 38(3), 557–577. https://doi.org/10.1017/S0048577201990559
- Kota, S., Kelsey, K., Rigoni, J., & Molfese, D. (2013). Feasibility of using event-related potentials as a sideline measure of neurocognitive dysfunction during sporting events. Neuroreport, 24(8), 437–439. https://doi.org/10.1097/WNR.0b013e3283616512
- Kumar, N., Singh, M., Sood, S., Roy, P. S., & Behera, J. K (2012). Effect of acute moderate exercise on cognitive P300 in persons having sedentary lifestyles. International Journal of Applied and Basic Medical Research, 2(1), 67–69. https://doi.org/10.4103/2229-516x.96813
- Kumar, N., Sood, S., Singh, M., Beena, & Sakshi. (2010). Effect of acute moderate exercise on cognitive event-related potentials n100, p200, n200, and interpeak latencies. Indian Journal of Psychological Medicine, 32(2), 131–135. https://doi.org/10.4103/0253-7176.78511
- Lambourne, K., & Tomporowski, P. (2010). The effect of exercise-induced arousal on cognitive task performance: A meta-regression analysis. Brain Research, 1341, 12–24. https://doi.org/10.1016/j.brainres.2010.03.091
- Laursen, P., & Jenkins, D. (2002). The scientific basis for high-intensity interval training. Sports Medicine, 32(1), 53–73. https://doi.org/10.2165/00007256-200232010-00003
- Liebherr, M., Corcoran, A., Alday, P., Coussens, S., Bellan, V., Howlett, C., & Bornkessel-Schlesewsky, I. (2021). Plasma Hsp90 levels in patients with systemic sclerosis and relation to lung and skin involvement: A cross-sectional and longitudinal study. Scientific Reports, 11(1), 1–13. https://doi.org/10.1038/s41598-020-79139-8
- Ligeza, T. S., Maciejczyk, M., Kalamala, P., Szygula, Z., & Wyczesany, M. (2018). Moderate-intensity exercise boosts the N2 neural inhibition marker: A randomized and counterbalanced ERP study with precisely controlled exercise intensity. Biological Psychology, 135, 170–179. https://doi.org/10.1016/j.biopsycho.2018.04.003
- Lind, R. R., Beck, M. M., Wikman, J., Malarski, K., Krustrup, P., Lundbye-Jensen, J., & Geertsen, S. S. (2019). Acute high-intensity football games can improve children's inhibitory control and neurophysiological measures of attention. Scandinavian Journal of Medicine & Science in Sports, 29(10), 1546–1562. https://doi.org/10.1111/sms.13485
- Loprinzi, P. D., Blough, J., Crawford, L., Ryu, S., Zou, L., & Li, H. (2019). The temporal effects of acute exercise on episodic memory function: Systematic review with meta-analysis. Brain Sciences, 9(4), 87. https://doi.org/10.3390/brainsci9040087
- LoTemplio, S., Silcox, J., Federmeier, K., & Payne, B. (2021). Inter-and intra-individual coupling between pupillary, electrophysiological, and behavioral responses in a visual oddball task. Psychophysiology, 58(4), e13758. https://doi.org/10.1111/psyp.13758.
- Luck, S. J. (2005). An introduction to the event-related potential technique. MIT Press.
- Ludyga, S., Brand, S., Gerber, M., Weber, P., Brotzmann, M., Habibifar, F., & Puhse, U. (2017). An event-related potential investigation of the acute effects of aerobic and coordinative exercise on inhibitory control in children with ADHD. Developmental Cognitive Neuroscience, 28, 21–28. https://doi.org/10.1016/j.dcn.2017.10.007
- Ludyga, S., Gerber, M., Brand, S., Holsboer-Trachsler, E., & Pühse, U. (2016). Acute effects of moderate aerobic exercise on specific aspects of executive function in different age and fitness groups: A meta-analysis. Psychophysiology, 53(11), 1611–1626. https://doi.org/10.1111/psyp.12736
- Ludyga, S., Gerber, M., Pühse, U., Looser, V., & Kamijo, K. (2020). Systematic review and meta-analysis investigating moderators of long-term effects of exercise on cognition in healthy individuals. Nature Human Behaviour, 4(6), 603–612. https://doi.org/10.1038/s41562-020-0851-8.
- Lyall, G., Davies, M., Ferguson, C., Porter, K., & Birch, K. (2019). In-exercise vascular shear rate during acute continuous and interval exercise: Impact on endothelial function and miR-21. Journal of Applied Physiology, 127(6), 1754–1762. https://doi.org/10.1152/japplphysiol.00156.2019
- Magnie, M. N., Bermon, S., Martin, F., Madany-Lounis, M., Suisse, G., Muhammad, W., & Dolisi, C. (2000). P300, N400, aerobic fitness, and maximal aerobic exercise. Psychophysiology, 37(3), 369–377. https://doi.org/10.1111/1469-8986.3730369
- Mather, M., & Harley, C. W. (2016). The locus coeruleus: Essential for maintaining cognitive function and the aging brain. Trends in Cognitive Sciences, 20(3), 214–226. https://doi.org/10.1016/j.tics.2016.01.001
- Mather, M., Huang, R., Clewett, D., Nielsen, S. E., Velasco, R., Tu, K., Han, S., & Kennedy, B. L. (2020). Isometric exercise facilitates attention to salient events in women via the noradrenergic system. NeuroImage, 210, 116560. https://doi.org/10.1016/j.neuroimage.2020.116560
- McGowan, A. L., Chandler, M. C., Brascamp, J. W., & Pontifex, M. B. (2019). Pupillometric indices of locus-coeruleus activation are not modulated following single bouts of exercise. International Journal of Psychophysiology, 140, 41–52. https://doi.org/10.1016/j.ijpsycho.2019.04.004
- McMorris, T. (2021). The acute exercise-cognition interaction: From the catecholamines hypothesis to an interoception model. International Journal of Psychophysiology, 170, 75–88. https://doi.org/10.1016/j.ijpsycho.2021.10.005
- McMorris, T., Turner, A., Hale, B. J., & Sproule, J. (2016). Beyond the catecholamines hypothesis for an acute exercise-cognition interaction: A neurochemical perspective. In T. McMorris (Ed.), Exercise-cognition interaction: Neuroscience perspectives (pp. 65–103). https://doi.org/10.1016/B978-0-12-800778-5.00004-9.
- Meijer, A., Königs, M., Vermeulen, G., Visscher, C., Bosker, R., Hartman, E., & Oosterlaan, J. (2020). The effects of physical activity on brain structure and neurophysiological functioning in children: A systematic review and meta-analysis. Developmental Cognitive Neuroscience, 45, 100828. https://doi.org/10.1016/j.dcn.2020.100828.
- Melynyte, S., Ruksenas, O., & Griskova-Bulanova, I. (2017). Sex differences in equiprobable auditory Go/NoGo task: Effects on N2 and P3. Experimental Brain Research, 235(5), 1565–1574. https://doi.org/10.1007/s00221-017-4911-x
- Melynyte, S., Wang, G., & Griskova-Bulanova, I. (2018). Gender effects on auditory P300: A systematic review. International Journal of Psychophysiology, 133, 55–65. https://doi.org/10.1016/j.ijpsycho.2018.08.009
- Miyake, A., & Friedman, N. (2012). The nature and organization of individual differences in executive functions. Current Directions in Psychological Science, 21(1), 8–14. https://doi.org/10.1177/0963721411429458
- Moher, D., Liberati, A., Tetzlaff, J., Altman, D. G., & PRISMA Group. (2009). Preferred reporting items for systematic reviews and meta-analyses: The PRISMA Statement. Annals of Internal Medicine, 151(4), 264–269. https://doi.org/10.7326/0003-4819-151-4-200908180-00135
- Moreau, D., & Chou, E. (2019). The acute effect of high-intensity exercise on executive function: A meta-analysis. Perspectives on Psychological Science, 14(5), 734–764. https://doi.org/10.1177/1745691619850568
- Mourot, L., Bouhaddi, M., Tordi, N., Rouillon, J., & Regnard, J. (2004). Short-and long-term effects of a single bout of exercise on heart rate variability: Comparison between constant and interval training exercises. European Journal of Applied Physiology, 92(4), 508–517. https://doi.org/10.1007/s00421-004-1119-0.
- Murphy, P., Robertson, I., Balsters, J., & O'connell, R. (2011). Pupillometry and P3 index the locus coeruleus–noradrenergic arousal function in humans. Psychophysiology, 48(11), 1532–1543. https://doi.org/10.1111/j.1469-8986.2011.01226.x
- Näätänen, R. (1990). The role of attention in auditory information processing as revealed by event-related potentials and other brain measures of cognitive function. Behavioral and Brain Sciences, 13(2), 201–233. https://doi.org/10.1017/S0140525X00078407
- Nakamura, Y., Nishimoto, K., Akamatu, M., Takahashi, M., & Maruyama, A. (1999). The effect of jogging on P300 event related potentials. Electromyography and Clinical Neurophysiology, 39(2), 71–74.
- Napadow, V., Dhond, R., Conti, G., Makris, N., Brown, E., & Barbieri, R. (2008). Brain correlates of autonomic modulation: Combining heart rate variability with fMRI. NeuroImage, 42(1), 169–177. https://doi.org/10.1016/j.neuroimage.2008.04.238
- Nieuwenhuis, S., Aston-Jones, G., & Cohen, J. D. (2005). Decision making, the P3, and the locus coeruleus-norepinephrine system. Psychological Bulletin, 131(4), 510–532. https://doi.org/10.1037/0033-2909.131.4.510
- Nikolin, S., Martin, D., Loo, C., & Boonstra, T. (2018). Effects of TDCS dosage on working memory in healthy participants. Brain Stimulation, 11(3), 518–527. https://doi.org/10.1016/j.brs.2018.01.003
- Norris, C. J., Creem, D., Hendler, R., & Kober, H. (2018). Brief mindfulness meditation improves attention in novices: Evidence from ERPs and moderation by neuroticism. Frontiers in Human Neuroscience, 12, 315. https://doi.org/10.3389/fnhum.2018.00315
- Oberste, M., Javelle, F., Sharma, S., Joisten, N., Walzik, D., Bloch, W., & Zimmer, P. (2019). Effects and moderators of acute aerobic exercise on subsequent interference control: A systematic review and meta-analysis. Frontiers in Psychology, 10, 2616. https://doi.org/10.3389/fpsyg.2019.02616
- Ogoh, S., Washio, T., Suzuki, K., Iemitsu, M., Hashimoto, T., Iwamoto, E., & Bailey, D. (2021). Greater increase in internal carotid artery shear rate during aerobic interval compared to continuous exercise in healthy adult men. Physiological Reports, 9(2), e14705. https://doi.org/10.14814/phy2.14705
- O'Leary, K. C., Pontifex, M. B., Scudder, M. R., Brown, M. L., & Hillman, C. H. (2011). The effects of single bouts of aerobic exercise, exergaming, and videogame play on cognitive control. Clinical Neurophysiology, 122(8), 1518–1525. https://doi.org/10.1016/j.clinph.2011.01.049
- Pedroso, R., Fraga, F., Ayán, C., Cancela Carral, J., Scarpari, L., & Santos-Galduróz, R. (2017). Effects of physical activity on the P300 component in elderly people: A systematic review. Psychogeriatrics, 17(6), 479–487. https://doi.org/10.1111/psyg.12242
- Petersen, I., Hoyniak, C., Bates, J., Staples, A., & Molfese, D. (2018). A longitudinal, within-person investigation of the association between the P3 ERP component and externalizing behavior problems in young children. Journal of Child Psychology and Psychiatry, 59(10), 1044–1051. https://doi.org/10.1111/jcpp.12975
- Petersen, S. E., & Posner, M. I. (2012). The attention system of the human brain: 20 years after. Annual Review of Neuroscience, 35(1), 73–89. https://doi.org/10.1146/annurev-neuro-062111-150525
- Petruzzello, S., Landers, D., Hatfield, B., Kubitz, K., & Salazar, W. (1991). A meta-analysis on the anxiety-reducing effects of acute and chronic exercise. Sports Medicine, 11(3), 143–182. https://doi.org/10.2165/00007256-199111030-00002
- Physical Activity Guidelines Advisory Committee. (2018). 2018 physical activity guidelines advisory committee scientific report. https://health.gov/paguidelines/second-edition/report/.
- Polich, J. (2003). Detection of change: Event-related potential and fMRI findings. Springer.
- Polich, J. (2007). Updating P300: An integrative theory of P3a and P3b. Clinical Neurophysiology, 118(10), 2128–2148. https://doi.org/10.1016/j.clinph.2007.04.019
- Polich, J., & Martin, S. (1992). P300, cognitive capability, and personality: A correlational study of university undergraduates. Personality and Individual Differences, 13(5), 533–543. https://doi.org/10.1016/0191-8869(92)90194-T
- Pontifex, M. B., McGowan, A., Chandler, M., Gwizdala, K., Parks, A. C., Fenn, K., & Kamijo, K. (2019). A primer on investigating the after effects of acute bouts of physical activity on cognition. Psychology of Sport and Exercise, 40, 1–22. https://doi.org/10.1016/j.psychsport.2018.08.015
- Pontifex, M. B., Parks, A. C., Henning, D. A., & Kamijo, K. (2015). Single bouts of exercise selectively sustain attentional processes. Psychophysiology, 52(5), 618–625. https://doi.org/10.1111/psyp.12395
- Pontifex, M. B., Parks, A. C., Paoli, A. G. D., Schroder, H. S., & Moser, J. S. (2021). The effect of acute exercise for reducing cognitive alterations associated with individuals high in anxiety. International Journal of Psychophysiology, 167, 47–56. https://doi.org/10.1016/j.ijpsycho.2021.06.008.
- Pontifex, M. B., Saliba, B. J., Raine, L. B., Picchietti, D. L., & Hillman, C. H. (2013). Exercise improves behavioral, neurocognitive, and scholastic performance in children with attention-deficit/hyperactivity disorder. The Journal of Pediatrics, 162(3), 543–551. https://doi.org/10.1016/j.jpeds.2012.08.036
- Popovich, C., & Staines, W. R. (2015). Acute aerobic exercise enhances attentional modulation of somatosensory event-related potentials during a tactile discrimination task. Behavioural Brain Research, 281, 267–275. https://doi.org/10.1016/j.bbr.2014.12.045
- Raine, L. B., Drollette, E. S., Kao, S.-C., Westfall, D., Chaddock-Heyman, L., Kramer, A. F., Khan, N., & Hillman, C. (2018). The associations between adiposity, cognitive function, and achievement in children. Medicine & Science in Sports & Exercise, 50(9), 1868–1874. https://doi.org/10.1249/MSS.0000000000001650
- Raine, L. B., Kao, S.-C., Pindus, D., Westfall, D. R., Shigeta, T. T., Logan, N., Cadenas-Sanchez, C., Li, J., Drollette, E. S., Pontifex, M., Khan, N. A., Kramer, A. F., & Hillman, C. H. (2018). A large-scale reanalysis of childhood fitness and inhibitory control. Journal of Cognitive Enhancement, 2(2), 170–192. https://doi.org/10.1007/s41465-018-0070-7
- Rebar, A., Stanton, R., Geard, D., Short, C., Duncan, M., & Vandelanotte, C. (2015). A meta-meta-analysis of the effect of physical activity on depression and anxiety in non-clinical adult populations. Health Psychology Review, 9(3), 366–378. https://doi.org/10.1080/17437199.2015.1022901
- Riggins, T., & Scott, L. S. (2020). P300 development from infancy to adolescence. Psychophysiology, 57(7), e13346. https://doi.org/10.1111/psyp.13346
- Scanlon, J. E. M., Sieben, A. J., Holyk, K. R., & Mathewson, K. E. (2017). Your brain on bikes: P3, MMN/N2b, and baseline noise while pedaling a stationary bike. Psychophysiology, 54(6), 927–937. https://doi.org/10.1111/psyp.12850
- Scudder, M. R., Drollette, E. S., Pontifex, M. B., & Hillman, C. H. (2012). Neuroelectric indices of goal maintenance following a single bout of physical activity. Biological Psychology, 89(2), 528–531.
- Shibasaki, M., Namba, M., Kamijo, Y. I., Ito, T., Kakigi, R., & Nakata, H. (2019). Effects of repetitive exercise and thermal stress on human cognitive processing. Physiological Reports, 7(4), e14003. https://doi.org/10.14814/phy2.14003
- Shvartz, E., & Reibold, R. (1990). Aerobic fitness norms for males and females aged 6 to 75 years: A review. Aviation, Space, and Environmental Medicine, 61(1), 3–11.
- Sibley, B. A., & Etnier, J. L. (2003). The relationship between physical activity and cognition in children: A meta-analysis. Pediatric Exercise Science, 15(3), 243–256. https://doi.org/10.1123/pes.15.3.243
- Soltani, M., & Knight, R. (2000). Neural origins of the P300. Critical Reviews™ in Neurobiology, 14, 3–4.
- Stroth, S., Kubesch, S., Dieterle, K., Ruchsow, M., Heim, R., & Kiefer, M. (2009). Physical fitness, but not acute exercise modulates event-related potential indices for executive control in healthy adolescents. Brain Research, 1269, 114–124. https://doi.org/10.1016/j.brainres.2009.02.073
- Swatridge, K., Regan, K., Staines, W. R., Roy, E., & Middleton, L. E. (2017). The acute effects of aerobic exercise on cognitive control among people with chronic stroke. Journal of Stroke and Cerebrovascular Diseases, 26(12), 2742–2748. https://doi.org/10.1016/j.jstrokecerebrovasdis.2017.06.050
- Tallon, C., Simair, R., Koziol, A., Ainslie, P., & McManus, A. (2019). Intracranial vascular responses to high-intensity interval exercise and moderate-intensity steady-state exercise in children. Pediatric Exercise Science, 31(3), 290–295. https://doi.org/10.1123/pes.2018-0234
- Talukdar, T., Nikolaidis, A., Zwilling, C. E., Paul, E. J., Hillman, C. H., Cohen, N. J., Kramer, A. F., & Barbey, A. K. (2018). Aerobic fitness explains individual differences in the functional brain connectome of healthy young adults. Cerebral Cortex, 28(10), 3600–3609. https://doi.org/10.1093/cercor/bhx232
- Tomporowski, P., & Pesce, C. (2019). Exercise, sports, and performance arts benefit cognition via a common process. Psychological Bulletin, 145, 929–951. https://doi.org/10.1037/bul0000200
- Tsai, C.-L., Chen, F.-C., Pan, C.-Y., Wang, C.-H., Huang, T.-H., & Chen, T.-C. (2014). Impact of acute aerobic exercise and cardiorespiratory fitness on visuospatial attention performance and serum BDNF levels. Psychoneuroendocrinology, 41, 121–131. https://doi.org/10.1016/j.psyneuen.2013.12.014
- Tsai, C.-L., Pan, C.-Y., Chen, F.-C., Wang, C.-H., & Chou, F.-Y. (2016). Effects of acute aerobic exercise on a task-switching protocol and brain-derived neurotrophic factor concentrations in young adults with different levels of cardiorespiratory fitness. Experimental Physiology, 101(7), 836–850. https://doi.org/10.1113/EP085682
- Tsai, C.-L., Pan, C.-Y., Tseng, Y.-T., Chen, F.-C., Chang, Y.-C., & Wang, T.-C. (2021). Acute effects of high-intensity interval training and moderate-intensity continuous exercise on BDNF and irisin levels and neurocognitive performance in late middle-aged and older adults. Behavioural Brain Research, 413, 113472. https://doi.org/10.1016/j.bbr.2021.113472
- Tsai, C. L., Ukropec, J., Ukropcová, B., & Pai, M. C. (2018). An acute bout of aerobic or strength exercise specifically modifies circulating exerkine levels and neurocognitive functions in elderly individuals with mild cognitive impairment. NeuroImage: Clinical, 17, 272–284. https://doi.org/10.1016/j.nicl.2017.10.028
- Tsai, C.-L., Wang, C.-H., Pan, C.-Y., Chen, F.-C., Huang, T.-H., & Chou, F.-Y. (2014). Executive function and endocrinological responses to acute resistance exercise. Frontiers in Behavioral Neuroscience, 8(AUG), https://doi.org/10.3389/fnbeh.2014.00262
- Tsai, Y. J., Hsieh, S. S., Huang, C. J., & Hung, T. M. (2021). Dose-response effects of acute aerobic exercise intensity on inhibitory control in children with attention deficit/hyperactivity disorder. Frontiers in Human Neuroscience, 15, 316. https://doi.org/10.3389/fnhum.2021.617596.
- van Dinteren, R., Arns, M., Jongsma, M. L. A., & Kessels, R. P. C. (2014). P300 development across the lifespan: A systematic review and meta-analysis. PLoS ONE, 9(2), e87347. https://doi.org/10.1371/journal.pone.0087347
- Verleger, R. (1997). On the utility of P3 latency as an index of mental chronometry. Psychophysiology, 34(2), 131–156. https://doi.org/10.1111/j.1469-8986.1997.tb02125.x
- Verleger, R. (2020). Effects of relevance and response frequency on P3b amplitudes: Review of findings and comparison of hypotheses about the process reflected by P3b. Psychophysiology, 57(7), e13542. https://doi.org/10.1111/psyp.13542
- Viechtbauer, W. (2010). Conducting meta-analyses in R with the metafor package. Journal of Statistical Software, 36(3), 1–48. https://doi.org/10.18637/jss.v036.i03
- Wang, D., Zhou, C., & Chang, Y. K. (2015). Acute exercise ameliorates craving and inhibitory deficits in methamphetamine: An ERP study. Physiology & Behavior, 147, 38–46. https://doi.org/10.1016/j.physbeh.2015.04.008
- Wang, D., Zhou, C., Zhao, M., Wu, X., & Chang, Y. K. (2016). Dose–response relationships between exercise intensity, cravings, and inhibitory control in methamphetamine dependence: An ERPs study. Drug and Alcohol Dependence, 161, 331–339. https://doi.org/10.1016/j.drugalcdep.2016.02.023
- Weaver, S., Skinner, B., Furlong, R., Lucas, R., Cable, T., Rendeiro, C., … Lucas, S. (2020). Cerebral hemodynamic and neurotrophic factor responses are dependent on the type of exercise. Frontiers in Physiology, 11, 609935. https://doi.org/10.3389/fphys.2020.609935.
- Wen, H.-J., & Tsai, C.-L. (2020). Effects of acute aerobic exercise combined with resistance exercise on neurocognitive performance in obese women. Brain Sciences, 10(11), 767. https://doi.org/10.3390/brainsci10110767
- Wilke, J., Giesche, F., Klier, K., Vogt, L., Herrmann, E., & Banzer, W. (2019). Acute effects of resistance exercise on cognitive function in healthy adults: A systematic review with multilevel meta-analysis. Sports Medicine, 49(6), 905–916. https://doi.org/10.1007/s40279-019-01085-x
- Winneke, A., Hübner, L., Godde, B., & Voelcker-Rehage, C. (2019). Moderate cardiovascular exercise speeds up neural markers of stimulus evaluation during attentional control processes. Journal of Clinical Medicine, 8(9), 1348. https://doi.org/10.3390/jcm8091348
- Wolff, N., & Beste, C. (2020). Short-term smartphone app–based focused attention meditation diminishes cognitive flexibility. Journal of Cognitive Neuroscience, 32(8), 1484–1496. https://doi.org/10.1162/jocn_a_01564
- Won, J., Wu, S., Ji, H., Smith, J. C., & Park, J. (2017). Executive function and the P300 after treadmill exercise and futsal in college soccer players. Sports, 5(4), https://doi.org/10.3390/sports5040073
- Wong, C. N., Chaddock-Heyman, L., Voss, M. W., Burzynska, A. Z., Basak, C., Erickson, K. I., R. S. Prakash, A. N. Szabo-Reed, S. M. Phillips, T. Wojcicki, M. L. Mailey, E. McAuley, & Kramer, A. F. (2015). Brain activation during dual-task processing is associated with cardiorespiratory fitness and performance in older adults. Frontiers in Aging Neuroscience, 7, 154. https://doi.org/10.3389/fnagi.2015.00154
- Wu, C. H., Karageorghis, C. I., Wang, C. C., Chu, C. H., Kao, S. C., Hung, T. M., & Chang, Y. K. (2019). Effects of acute aerobic and resistance exercise on executive function: An ERP study. Journal of Science and Medicine in Sport, 22(12), 1367–1372. https://doi.org/10.1016/j.jsams.2019.07.009
- Wu, S., Ji, H., Won, J., Liu, X., & Park, J. J. (2021). Effects of acute visual stimulation exercise on attention processes: An ERP study. International Journal of Environmental Research and Public Health, 18(3), 1107. https://doi.org/10.3390/ijerph18031107
- Xie, C., Alderman, B. L., Meng, F., Ai, J., Chang, Y. K., & Li, A. (2020). Acute high-intensity interval exercise improves inhibitory control among young adult males with obesity. Frontiers in Psychology, 11, 1291. https://doi.org/10.3389/fpsyg.2020.01291
- Yagi, Y., Coburn, K. L., Estes, K. M., & Arruda, J. E. (1999). Effects of aerobic exercise and gender on visual and auditory P300, reaction time, and accuracy. European Journal of Applied Physiology and Occupational Physiology, 80(5), 402–408. https://doi.org/10.1007/s004210050611
- Yerkes, R., & Dodson, J. (1908). The relation of strength of stimulus to rapidity of habit-formation.
- Yu, C. L., Hsieh, S. S., Chueh, T. Y., Huang, C. J., Hillman, C. H., & Hung, T. M. (2020). The effects of acute aerobic exercise on inhibitory control and resting state heart rate variability in children with ADHD. Scientific Reports, 10(1), 1–15.
- Yu, Q., Herold, F., Becker, B., KluGah-Brown, B., Zhang, Y., Perrey, S., Veronese, N., Müller, N. G., Kramer, A. F., & Zou, L. (2021). Cognitive benefits of exercise interventions: An fMRI activation likelihood estimation meta-analysis. Brain Structure and Function, 226(3), 601–619. https://doi.org/10.1007/s00429-021-02247-2
- Zhou, F., & Qin, C. (2019). Acute moderate-intensity exercise generally enhances attentional resources related to perceptual processing. Frontiers in Psychology, 10, 2547. https://doi.org/10.3389/fpsyg.2019.02547