Abstract
Poor-solvating property of supercritical carbon dioxide (scCO2) has been a great challenge, which limits the use of CO2 as a common “green” solvent. The present report describes that by increasing molar volume (v) and lowering the melting temperature, which lowers cohesive energy density or solubility parameter (δ), it is possible to increase the solubility of metal-based catalysts in scCO2 without using costly fluorinated or tailor-made CO2-philic modifications. We have studied various chlorodistannoxanes (1) and Cu–β-diketonates (2) to support our views. The study of bio-diesel production and transesterification of hindered esters using 1 in scCO2 shows a 2–8-folds rate enhancement coupled with an easier catalyst and product separation than that in organic solvents. The methodology, which works at least within the range of Van der Waals sphere of interactions, can be useful to solubilizing the molecules in scCO2 and carries great opportunity in catalysis as well as in separation science.
Introduction
SupercriticalFootnote carbon dioxide (scCO2) among all supercritical fluids (SCFs) has gained tremendous attention in recent years primarily due to its environmentally benign nature and interesting physical properties Citation1–4. scCO2 is an inert, abundant, and non-toxic solvent and thus is considered to be one of the most environmentally benign solvents Citation5 Citation6. However, solubility of various components in scCO2 is extremely poor, which prevents the broad use of scCO2 as a “green solvent.” The major difficulty arises in solubilizing inorganic compounds in scCO2. Many of these polar inorganic materials, used as homogeneous catalysts, have very low solubility in non-polar solvents, such as scCO2, when compared to common polar organic solvents. In fact, low solubility of key inorganic catalysts in scCO2 severely limits their suitability for carrying out catalytic reactions to the extent where the reaction ceases to occur Citation3 Citation7. Due to such difficulties, high temperature and pressure are employed to obtain sufficient solubility of the catalyst in order to drive the reaction forward. However, the process is quite costly and safety becomes a prevalent issue for practical applications. Moreover, various chemical reactions are sensitive to temperature and may generate undesired side products.
Among many CO2-philes, fluorinated moieties or surfactants are mostly used as CO2-philic groups to enhance solubility in scCO2 Citation8. Besides fluorinated groups, silyl and alkyl groups are other type of moieties which are frequently used to enhance solubility in scCO2 Citation9. Even though such modified catalysts increase solubility in scCO2, particularly the synthesis of fluorine-modified catalysts dramatically increases operating expenses making this method economically undesirable. Beckman and co-workers Citation10 have reported the design of a polyether material of high solubility in scCO2 as a comparatively cheaper alternative. However, this type of tailor-made material is suitable for very specific applications. Jessop and colleagues Citation11 Citation12 have developed switchable solvent concept based on the reaction with CO2 to solubilize polar or ionic components in scCO2, but the use of this methodology also has a number of limitations as pointed out by the authors.
In recent years, tremendous efforts have been made toward homogenizing the metal-based components in CO2 by several ways Citation13–15. Extraction of metal ions, such as lanthanides, using scCO2 has been studied extensively. Metal ions were made soluble by chelating with acetylacetonate (acac) ligand Citation16. Ligand-like acac tends to neutralize the charge on the metal ions facilitating dissolution in scCO2 and thus easy extraction. Guironnet et al. Citation17 reported high polymerization yield of ethylene in scCO2 using a Ni phenoxyimine complex as catalyst. The success of the reaction was correlated to the high solubility of the neutral Ni catalyst in scCO2. There are several literature reports of moderate to highly soluble inorganic complexes or catalysts in scCO2, but to the best of our knowledge, almost no systematic justification or design criteria are available which can provide a sufficient explanation for the solubility of metal complexes in scCO2. Therefore, efforts to find or design catalysts, which are directly soluble in scCO2 without the aid of any CO2-philic groups are highly desirable, if the routine use of scCO2 as a green solvent is to be realized.
In order to develop scCO2 soluble catalysts, consideration should be given to the low polarity and other critical solvent properties of dense phase CO2. Molecules that have low melting points and additionally possess low intermolecular cohesive energy density (CED) should be suitable to have high solubility in scCO2. Molar volume (v), which is inversely related to CED, can be increased to achieve low CED and thus high solubility (Equation 1). Introducing big alkyl groups into the metal center or catalyst core may be one option to attain high v and thus low CED values. Fulfilling these solubility requirements, we have recently found that several, 1,3-dichloro-1,1,3,3-tetraalkyldistannoxanes (1, ) complexes and Cu–β-diketonates (2, ) complexes have solubility in liquid or scCO2 as a function of structure, covering the range from completely insoluble to highly soluble compounds.Our recent observation on the effect of lyophilic character of distannoxane complexes with varying reactivity Citation18 and readily available and widely studied diketone complexes of Cu(II) have attracted our interest for the present study in CO2 elaborately. Interestingly 1 is also known to be catalytically active for carrying several important organic transformations which include ring-opening polymerizations (ROP), polyurethane synthesis, polyester synthesis, and transesterification reactions, and so on Citation19–25. All of these reactions are traditionally carried out in conventional organic solvents that may pose environmental hazards.
Figure 1. 1,3-Dichloro-1,1,3,3-tetraalkyldistannoxane catalyst series (1) and Cu–β-diketonates (2) used for this study. Catalysts 1a–1b (21), 1c–1f Citation22, 1g Citation23, 1h–1i Citation24, 2b–2c Citation25, 2e–2f Citation25 were synthesized according to reported procedure. 2a and 2d were purchased from Aldrich Chemical Company, USA.
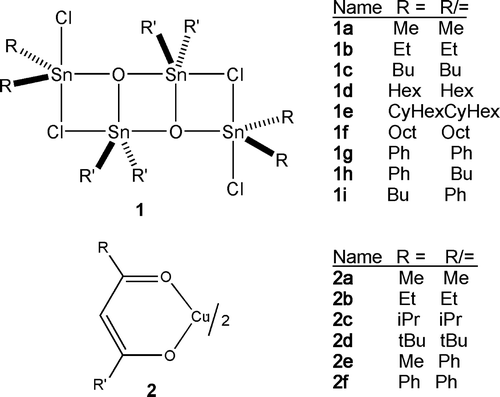
Taking advantage of the high solubility of 1 in scCO2, we have demonstrated that increasing solubility of metal-based catalyst in CO2 can be advantageous in chemical reactions in terms of rate acceleration, catalyst recycle, and product separation as shown in the methanolysis of triglycerides and transesterification of sterically hindered esters in scCO2 catalyzed by 1.
Results and discussion
Synthesis and stability studies of 1 and 2
Various tin and copper compounds used for this study are synthesized according to the literature reported procedures with readily available starting materials Citation21–25. The compounds are also oxygen and moisture stable, and thus can be handled without using any specialized equipment Citation26.
The stability and reactivity of 1 toward CO2 was checked at high pressure (100 bar) and temperature (110°C); we found that these molecules are inert to CO2 as indicated by the absence of any carbonyl peak in the IR spectra of CO2 treated 1 Citation27. Compounds derived from 1 after treatment with methanol under reflux contain bridging alkoxides, which are also inert toward CO2. This is in contrary to the case of many 1,3-diethoxydistannoxanes, which contain terminal alkoxy groups and react with CO2 Citation28.
Solubility, cohesive energy density (CED), and molar volume (v)
We have measured the solubility of catalysts 1 (50 bar, 25°C) and 2 (140 bar, 40°C) in CO2, and solubility (mol/l) of various metal-based catalysts are shown in . The solubility of the catalysts was also measured at various temperatures and pressures too (see supporting information). indicates that solubility follows a definite trend as the structure of the material is varied. The solubility of 1 reaches at about 0.06 mol/l at 50 bar CO2 pressure and at 25°C (A). δ and v, which were determined using methods of intrinsic viscosity measurement Citation29 and infinite dilution Citation30, respectively, along with melting points, are shown in . A reveals that with gradually decreasing melting point as ν of the catalysts is increased, δ of the catalysts decreases and subsequently increasing molar solubility of 1 in scCO2.
Figure 2. Variation of solubility with melting point, solubility parameter (δ) and molar volume (v) for catalysts 1 and 2. (A) The figure shows solubility (mol/L) ▴ of different cholorostannoxane catalysts (1) at 50 bar CO2 pressure and at ambient temperature, melting point (°C) ♦, δ (MPa1/2) ▾and ν (L/mol) ▪. (B) The figure shows solubility (mol/L) ▴ of different Cu–β-diketonates (2) at 140 bar CO2 pressure and at 40 °C, δ (MPa1/2) ▾ and ν (L/mol) ▪. L represents liter.
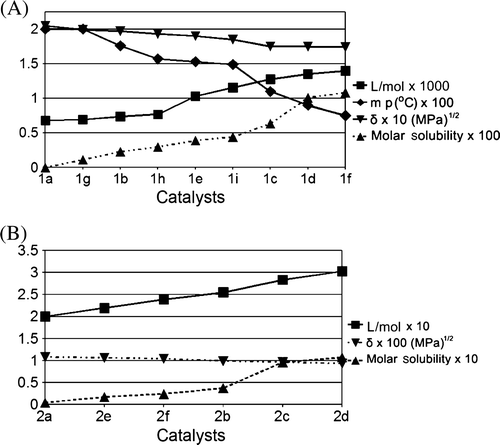
When searching for a highly scCO2 soluble inorganic catalyst (in the absence of any functionalization by known CO2-philes) the role of CED or δ can be considered as important governing factors. As noted by previous researchers, it is possible to enhance the solubility of polar compounds in non-polar solvents by introducing long alkyl chains or bulky groups to the compounds Citation31–33. In a first approximation, high solubility of any solute in scCO2 is linked to a low solute CED and hence the proximity of the solute CED to that of scCO2. The CED (Equation 1) is a function of polarizability (α) and v
Citation34
Citation35.δ, simply the square root of CED, is another parameter frequently used to explain solubility trends (Equation 2) Citation36. Viscosity correlates with the δ Citation28 and hence viscosity has been used to determine δ of 1 for the current study. A also shows the δ values obtained for different cholorostannoxane catalysts (1).
As indicated in A, solubility increases as we introduce longer chains to the cholorostannoxane's molecular structure. Obviously, the introduction of the long chains increases v significantly, resulting in both low-CED and δ values. A also illustrates that 1d, an n-hexyl substituted cholorostannoxane, has higher solubility compared to 1e, which is the cyclohexyl derivative of the catalyst. This is due to the fact that the replacement of hexyl groups with cyclohexyl groups in the catalyst decreases the v and subsequently increases the CED of the catalyst (A).
Comparison of scCO2 solubility for mixed cholorostannoxane catalysts, such as 1h and 1i, reveals that the catalyst with terminal longer alkyl chains (butyl group, 1i) shows higher solubility due to higher v, and hence lower CED or δ values (A). It is also apparent from , that the melting point decreases with increasing v, reflecting potentially decreased solute–solute interactions. Thus, along with intermolecular interactions between cholorostannoxanes and CO2 (solute–solvent), v plays a key function in determining the catalyst solubility in scCO2. In other words within the same catalyst framework, it is possible to obtain enhanced solubility simply by increasing the ν where other interactions may also exist.
In order to strengthen the fact that above-mentioned findings are not only with 1, we have also determined molar solubility, δ and v of several Cu–β-diketonates (2) at 140 bar CO2 pressure and 40°C. The trends found in the case of 2 are very similar to 1 in terms of molar solubility, δ and ν (B), implying that the finding is applicable to solubilize metal-based catalysts in CO2.
Effect of molar volume in catalysis
Given the importance and versatility of cholorostannoxanes (1) as catalysts for industrially important organic transformations Citation18–20, we performed several catalytic reactions in scCO2 that are usually conducted in conventional organic solvents. Compound 1c, which is easy and inexpensive to synthesize was used to carry out catalytic reaction in scCO2.
The transesterification of triglycerides has recently received significant attention due to enhanced interest in bio-diesel production Citation37
Citation38. Current transesterification methods that use either acid or alkali catalysts Citation37–40, and enzymes Citation37
Citation38
Citation41 have exhibit notable shortcomings Citation37
Citation38. Catalyst recovery, product separation, saponification of products, and side reactions due to fatty acid and water contamination are some of problems commonly encountered using acid and alkali catalysts. Stability and cost are the major problems associated with use of enzymes to catalyze the transesterification reaction. The catalyst 1c was found to be highly efficient in carrying out transesterification of sunflower oil (SFO) in scCO2. At 100 bar CO2 pressure and 110°C temperature, 45 µmol of 1c can transesterify 4 g of SFO within 4 h by using 1 mL methanol (Equation 3) producing 1 g of glycerol, turn over frequency (TOF) 59.2 h−1. The same reaction performed in n-octane under similar conditions was found to be three times slower than that in CO2, producing only 0.6 g of glycerol in 10 h (), TOF 20 h−1. Progress of reaction was monitored by gas chromatography using DB 5 column.
Figure 3. Comparison of reaction rates using catalyst 1c. Transesterification reaction rates catalyzed by 1c in scCO2 and in organic solvents. ▾, Ethyl-2,2-dimethylacetoacetate transesterification with benzyl alcohol in toluene; ▴, triglyceride esterification with methanol in n-octane; •, ethyl-2,2-dimethylacetoacetate transesterification with benzyl alcohol in CO2; ▪, triglyceride transesterification with methanol in CO2.
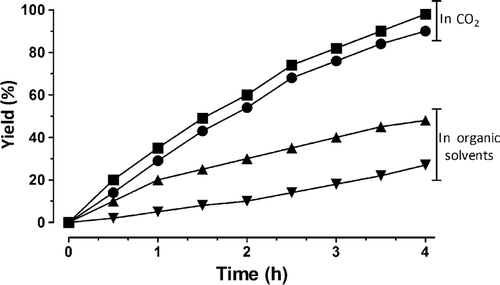
After completion of the reaction, the CO2 is vented and the solid catalyst conveniently settles at the bottom of the reaction chamber. The precipitated catalyst can be collected by filtration and recycled. Dilution of the glycerol rich phase with water further precipitates the remaining catalyst which was used further catalyzing the similar reactions and found to maintain almost identical catalytic activity. The catalyst can also be recovered after decanting of methyl esters after the reaction finishes, followed by extraction with hexane of the glycerol layer. Chemical analysis (supporting information) indicates that catalysts survive the reaction conditions and can be recycled without loss of activity. This is a major advantage of using chlorodistannoxanes in scCO2 over other distannoxanes as the latter form by-products under typical reaction conditions Citation42.
Non-enolizable esters, such as ethyl-2,2-dimethylacetoacetate (ED), are relatively difficult to esterify, but can be esterified with benzyl alcohol (Equation 4) in toluene in the presence of 1c at poor yield Citation22.
Compound 1c, when employed in CO2 (100 bar, 80°C), catalyzes the transesterification of ED by benzyl alcohol with 90% yield in 4 h. There is about 8-fold increase in reaction rate from the corresponding reaction in toluene at 80°C that reaches to completion at about 30 h (). The catalyst can be recovered easily and completely by hexane extraction following pressure reduction.
We have recovered and afterward reused catalyst in both the above-mentioned transesterification reactions. Results indicate (supporting information) that even after five times of catalyst recycle it retains catalyst activity to almost 100%. Thus, designing or identifying catalysts which are inert to CO2, it is possible to obtain not only the benefit of enhanced reaction rate, but also the advantage of separating and recycling the catalysts.
shows the yields (%) of reaction products of triglyceride and ED transesterifications using various chlorostannoxanes (1). Supporting our views, yield increases with the increasing molar volume or deceasing CED or δ values and decreasing melting points of 1. All these are responsible for high solubility of 1 in scCO2 and thus enhanced activity. However, it is worthwhile to mention that actual solubility of the catalysts in the reaction medium may be higher than that in neat CO2 due to the presence of other polar components, but the trend must remain same. Insoluble catalysts like 1g and 1b show least reactivities whereas highly soluble catalysts like 1c and 1f shows highest activities for transesterification reactions. Noticeable shift in activity from 1e to 1i was obtained when cyclohexyl group was replaced by peripheral butyl groups, which ultimately increase the molar volume and thus increased solubility of the catalyst.
Figure 4. Trends in catalytic activity (% yield) of various chlorostannoxanes (1) for sun flower oil methanolysis and ethyl-2,2-dimethylacetoacetate transesterification with benzyl alcohol in CO2, respectively. The results reflect higher solubility of the catalysts with increasing molar volume.
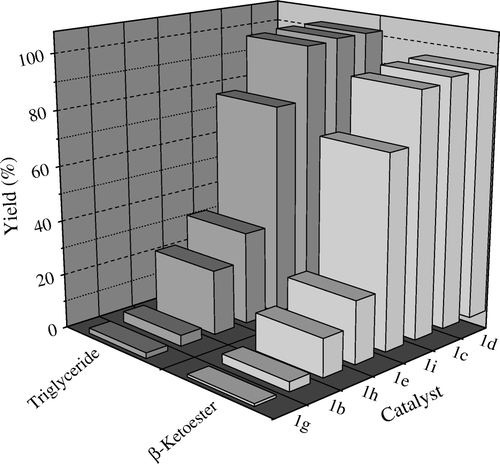
TOF (supporting information) of two types of transesterification reactions show similar trend as that of solubility and molar volume. Using 1f TOFs of 59.77 and 260, respectively, were obtained for triglyceride methanolysis and transesterification of ED. On the other hand TOF of 1g for the same reactions were found to be 1.2 and 2.8, respectively. As revealed by the different slopes in the plot (Figure S6, supporting information), TOFs are different for triglyceride methanolysis and transesterification of ED. This may be due to the difference in reaction rates of two types of transesterification reactions.
The products obtained during the transesterifcation reactions have been tested by inductively coupled plasma-optical emission spectroscopy (ICP-OES) Citation43, (limit of detection for Sn, 1 ppm) and ion chromatography (limit of detection for Cl−, 1 ppb). Results show no presence of Sn and Cl− by following the above-mentioned analytical techniques indicating no decomposition of the catalysts. Moreover the purity of the product obtained by gas chromatography, after completion of reaction, is >98%. Thus, the product can be directly obtained without further purification.
Practical implication of such highly CO2 soluble catalysts may be found, as described above, in high reaction rates and easy separations Citation44 Citation45 of product and catalysts. When transesterification reaction rates are concerned, presumably, CO2 addition to the mixture of methanol and triglyceride increases the mutual solubility of the reactants, and lowers the viscosity of the reaction medium Citation44–46, which may act to enhance the rate of the reaction. As only 0.03% (w/v) glycerol is soluble in CO2 Citation47, glycerol formed during reaction readily separates from the reaction mixture, driving the equilibrium forward. In the conventional process SFO, octane, and methanol form a biphasic medium leading to a much slower reaction.
Experimental
Methods
Chemicals were purchased from Aldrich, USA, and used without further purifications unless otherwise mentioned. Solvents were purified by using standard purification procedures before using in any reaction. Refined SFO was purchased from Agro Tech Foods Ltd., India. Chloride was estimated by using ion chromatography (Metrohm) method (limit of detection for chloride <0.01 ppb). Tin was determined by ICP-OES method (Perkin Elmer, Optima 4300 DV) within the limit of 1 ppm. All the high-pressure reactions and solubility measurement were carried out in 100 mL Parr reactor connected with a 4842 controller. Reaction products were analyzed by gas chromatography (Shimadzu, GC 2010) using a DB-5 column (J&W Scientific). 1H NMR spectra were obtained in a 300 MHz Varian FT spectrometer using deuteretated solvent as the lock. The spectra were collected at 25°C and chemical shifts (δ, ppm) were referenced to residual solvent peak (CDCl3 δ, 1H, 7.26 ppm). Electrospray ionization mass spectra (ESI-MS) were recorded by using a Micromass Q-TOF mass spectrometer. Infrared (IR) spectra were recorded by using a Perkin Elmer, Spectrum 100 instrument. The elemental analyses (C, H) were carried out with a Perkin-Elmer 240C elemental analyzer (accuracy ±0.3%).
Preparation of catalysts
R2SnO and R2SnCl2 (R = Hexyl, Cyclohexyl, Octyl, and Decyl) were prepared following previously published literature procedures.
Preparation of catalyst 1
1a, 1b, 1c, 1d, 1e and 1f
These chlorostannoxanes were synthesized according to Otera et al.'s Citation22 published procedure. A representative synthetic procedure is given for 1,3-dichlorotetra-n-butyldistannoxane (1c).
A mixture of Bu2SnO (1.0 g, 4.0 mmol) and Bu2SnCl2 (1.22 g, 4.0 mmol) in toluene (50 mL) was refluxed for several hours. After 10 h reflux, the reaction mixture became a clear solution. The reaction was further refluxed for another 7 h. The hot mixture was filtered and concentrated in vacuum to get 1,3-dichlorotetra-n-butyldistannoxane (1c). Pure product was obtained by recrystallizing from toluene. Yield 95%. Elemental analysis, 1H NMR and ESI-MS data are given in Table S1. Figure S1 shows the ESI-MS of 1c.
1g
This was synthesized according to Harrison and Molloy's Citation23 published procedure. Ph2SnCl2 (1.0 g, 2.9 mmol) and 2-aminobenzthiazole (436 mg, 2.9 mmol) in 25 mL acetone were stirred for 3 h while a white precipitate appeared during the reaction. Solution was filtered to remove any insolubles and evaporated to dryness to yield a solid white product. Yield 25%.
1h and 1i
These catalysts were synthesized according to Lu et al.'s Citation24 published procedure. Ph2SnO (1.0 g, 3.46 mmol) and Bu2SnCl2 (1.05 g, 3.45 mmol) were dissolved in 25 mL acetone in a 100 mL round-bottomed flask and refluxed for 5 h while stirring the reaction mixture. Reaction mixture was then cooled to room temperature and filtered to remove any insolubles. A solid product was obtained after concentrating the reaction mixture under vacuum. Pure crystalline 1h was obtained after crystallizing the solid from hexane. Yield 75%.
1i
Synthesis of 1i was performed similarly. Bu2SnO (0.720 g, 2.90 mmol) and Ph2SnCl2 (1.0 g, 2.908 mmol) were dissolved in 25 mL acetone in a 100 mL round-bottomed flask and refluxed for 3 h while stirring the reaction mixture. Most of the solid dissolved within 3 h of the reaction. After filtration, reaction mixture was cooled at room temperature and concentrated to obtain solid product. Pure needle-shaped crystals of 1i were obtained after crystallizing the solid from hexane. Yield 90%.
Preparation of catalyst 2
2a and 2d were purchased from Aldrich and used as they were received. Synthesis of 2b, 2c, 2e, and 2f were performed according to Fernelius and Bryant's Citation25 published procedures by using various β-diketones. Complexes were characterized by elemental (C, H) analysis, mass spectrometer and 1H NMR technique.
Analytical data for all the catalysts are given in supporting information.
Measurement of cohesive energy density (CED) or solubility parameter (δ)
CED or δ of catalysts was determined according to Lee and Lee's Citation29 published procedure. Solutions of different concentrations ranging form 0% to 10% (w/v) in various non-reactive and reasonably less volatile solvents were prepared in 25 mL volumetric flasks. Using standard procedure, an Ubbelhode viscometer was loaded with the solution and efflux time was measured for each solution. The measurement was repeated for five times for each solution. If T and T 0 are the efflux times of a solution with solute concentrations C and 0, respectively, for a particular solvent, a straight line is obtained when concentration is plotted against [(T–T 0)/T 0 C]. The specific viscosity, ηsp, of the solution was obtained form the intercept when the graph is extrapolated. ηsp is used to determine intrinsic viscosity, η. Once η is measured for various solvents, a graph is plotted with η versus solubility parameter δ. The maximum obtained from plot (Figure S5, supporting information) provides solubility parameter of solute or catalyst.
Measurement of molar volume (v)
This was performed according to Ruelle et al. Citation30 published procedure. Hexane was used as solvent for 1 whereas for 2 toluene was used. For example, 100 mg of 1c was dissolved in 100 mL hexane and the solution was sonicated for 10 min to dissolve completely. Solutions with five different concentrations (10–80%, w/v) of 1c were prepared by dilution. The stability of the solutions was checked by leaving the solutions for a period of 48 h at 25°C and no precipitate was observed. In a typical measurement, partial molar volumes of 1c at infinite dilution, was determined by using DMA-58 vibrating tube density meter (Anton Paar, A-8054 Graz, Austria) at 25°C. The plot of specific volume or reciprocal density (V
S) of a series of diluted solutions with respect to mass fraction (m
1c), following Equation 5 provides the intercept which represents partial specific volume of the solvent (hexane),
:
On the other hand, the partial specific volume of 1c,
, is obtained from the sum of both the intercept and the slope of the curve. The final v of 1c was obtained using Equation 6:
Estimation of Cl− and Sn in the catalysts
A representative method for the estimation of% of Cl− and Sn of catalyst 1c is described here. All other catalysts were run similarly in order to obtain their% of Cl− and Sn Citation43.
1c (110 mg, 0.1 mmol) was digested with equivalent amount of concentrated HNO3 (69%) in a porcelain crucible for 5 h. The resultant digested mass was then evaporated to dryness. The solid obtained after evaporation was dissolved in 10 mL of water and acidified with dilute HNO3 (6.9%) until solution turned clear. After filtering the solution, the filtrate was transferred into a 100 mL volumetric flask and diluted with water. The solution was then analyzed to estimate Cl− by using a Metrohm column in ion chromatography method. Same solution was used to determine Sn content by ICP-OES.
Solubility of catalysts in carbon dioxide (CO2)
Solubility of catalysts was measured by a modified high pressure Parr reactor apparatus. The apparatus was designed according to Alessi et al. Citation31 published procedure. A schematic representation of the apparatus is shown in (Figure S2, supporting information). CO2 gas was connected through a Haskel gas booster (AG 62) to the reactor, which is also connected to inline filters (F1, F2, 0.5 µm), valves (V1, V2, V3), and reservoir (R), respectively, as shown in Figure S2. A known amount of solute or catalyst was placed in a 100 mL Parr reactor jacketed with a heating mantle. CO2 was then introduced via the gas booster, and the pressure and temperature of the reactor were recorded. The system was allowed to attain equilibrium for 5 min while keeping the valves closed. CO2 gas was then vented to the atmosphere slowly. The solids deposited in the reservoir, inline filters, and valves (if any) were collected carefully, and weighed to determine the solubility in 100 mL of liquid CO2. The validity of the method was checked by measuring the solubility of salicylic acid in CO2 and also by measuring the solubility using a Thar Equipment Co. phase analyzer apparatus equipped with variable volume view cell Citation48. The results indicate that the solubility measurements using all the methods are in excellent agreements. The experimental results of these validity tests are given in the supplementary section (all of the Supplementary information can be found by clicking on the Supplementary Content tab at http://dx.doi.org/10.1080/17518253.2010.487840). The solubility of catalysts, such as 1c and 1e, were measured at various temperatures and pressures.
Validity of the method of solubility measurement
The validity of the method was checked by measuring the solubility of salicylic acid in CO2 at 40°C and also by measuring the solubility of salicylic acid in CO2 using a Thar Equipment Co. phase analyzer apparatus equipped with variable volume view cell. The results as shown in Figure S3 indicate that the solubility measurements using all the methods are in excellent agreements under various CO2 pressures.
Moreover solubility data of salicylic acid obtained by us is in very much agreement with the literature values (Table S3) Citation49.
Solubility of catalysts 2 were determined similarly and catalysts 2 were also found to be stable under similar reaction conditions.
Stability of catalyst in carbon dioxide (CO2)
1c (100 mg, 0.09 mmol) was taken in the reactor and CO2 was introduced at 100 bar of pressure. The temperature of the reactor was raised to 120°C. The mixture was stirred for 6 h and then the solid obtained after depressurizing the reaction vessel was checked by IR spectroscopy. Absence of any carbonyl peak in the IR spectrum indicates no CO2 incorporation into the catalyst framework. This clearly indicates the robust nature of the catalyst 1 to CO2. On the other hand diethoxytetrabutyldistannoxane [(OEt)Bu2SnOBu2Sn(OEt)]2 when treated with CO2 under high pressure, carbonate formation was observed as indicated by IR spectrum. Generally, stannoxanes with terminal alkoxy groups are susceptible to reaction with CO2.
Transesterification of sunflower oil (SFO)
SFO (4 g), methanol (1 mL), and 1c (50 mg, 0.0452 mmol) were added to a 100 mL Parr reactor containing a magnetic stir bar. Reactor was pressurized with CO2 at 55 bar and temperature was maintained at 110°C. The reaction mixture was stirred for 4 h while keeping the mixture at pressure and temperature. After 4 h, the pressure was released and the reactor cooled to room temperature. The reaction mixture was centrifuged for 10 min at 1000 rpm whereupon the solid catalyst was found to settle at the bottom of the reactor and then the catalyst was collected by filtration for reuse. The liquid portion of the reaction mixture separated into two layers, which were collected using a separatory funnel. The bottom layer (0.8 g) was found to be 98.7% glycerol as confirmed by GC analysis using a DB-5 column and also comparing with pure glycerol sample. The top layer was found to be methyl esters (3.15 g), the desired reaction product. Dilution of the glycerol with water resulted in further precipitate formation of dissolved catalyst. The recovered catalyst solids were combined and their chemical analysis indicated no chemical changes. Using the recovered catalyst, the reaction was performed again and results indicate identical activity of the catalyst.
For comparison, SFO (4 g), methanol (1 mL), and 1c (50 mg, 0.0452 mmol) were mixed with 25 mL n-octane in a round-bottomed flask fitted with a condenser. The mixture was refluxed for 10 h. The hexane was removed under vacuum and the products analyzed. The result showed formation of 3.12 gm methyl esters and only 0.6 g glycerol.
Same procedure was followed for other catalysts as well. Progress of reaction was followed by gas chromatography, comparing with authentic sample of glycerol. Activity of the catalyst was measured with respect to the amount of glycerol produced.
Transesterification of ethyl-2,2-dimethylacetoacetate (ED)
ED (790 mg, 5 mmol), benzyl alcohol (5.482 g, 50.69 mmol), and 1c (49.74 mg, 0.045 mmol) were taken in 100 mL Parr reactor with a stir bar placed inside. Then the reactor was pressurized with CO2 at 100 bar and temperature was raised to 80 °C. Reaction was carried out for 4 h and then reactor was cooled to room temperature and depressurized. The reaction mixture was concentrated under vacuum in order to remove any excess benzyl alcohol. The residue was purified by a short silica column with hexane and ethyl acetate (95:5) to give benzyl-2,2-dimethylethylacetotate (BD) as pure product. Yield 90%. 1H NMR (CDCl3, ppm), 1.28 (s, 6H), 1.83 (s, 3H), 4.95 (s, 2H), 7.13 (broad, s, 5H); ESI-MS: m/z, 220; Analytically calculated for C13H16O3, C, 70.89, H 7.32, Found: C, 70.85; H 7.25.
For comparison, ED (790 mg, 5 mmol), benzyl alcohol (5.482 g, 50.69 mmol) and 1c (49.74 mg, 0.045 mmol) were mixed with 25 mL toluene in a round-bottomed flask fitted with a condenser. The mixture was heated at 80°C for several hours. The yield of the reaction was checked from time to time and it took almost 30 h to complete the reaction.
Similarly other catalysts were used according to this procedure and yields in these cases were measured with respect to product formation by gas chromatography. Formation of product was compared with authentic sample synthesized and characterized previously.
Catalyst recovery
Catalyst was generally recovered for each reaction by extracting with hexane from the reaction mixture. Addition of hexane in reaction mixture separates out the product at the bottom of the reaction mixture, which is easily removed. Evaporation of hexane layer gives back the catalyst (98% recovery). Recovered catalyst was tested by 1H NMR, ESI-MS, and IR spectrophotometer techniques. The recovered catalyst was found to be identical to the starting catalyst. Absence of any carbonyl peak in the IR Citation50 shows no reaction of CO2 with the catalyst.
Catalyst recyclability
The recovered catalyst was tested in five cycles for both the reactions: (a) transesterification of SFO with methanol and (b) transesterification of ED with benzyl alcohol. Catalyst was physically separated and tested for each cycle. The results of catalyst recycling (supporting information) indicate that there is no depletion of catalyst reactivity even after five times of recycle for performing both the transesterification reactions.
Conclusion
In conclusion, this work demonstrates the possibility of designing inorganic catalysts that exhibit significant solubility in scCO2, without the need to functionalize the catalyst ligands with conventional CO2-philic groups. By changing v, one can drop the CED and δ values close enough to those of CO2 to induce relatively high solubility of components. The methodology would not only benefit the homogeneous catalysis but also in the separation science. Advantages of using CO2 as a green solvent include replacement of conventional hazardous solvents, enhanced reaction rates, high product yields, ease of separation of products, and convenient catalyst recovery.
Supplementary Data
Download Zip (117.4 KB)Acknowledgements
AG likes to thank UALR faculty start up grant and Department of Energy (grant number DE-FG36-06GO86072) for financial assistance to complete the work. AG would also like to thank Arkansas State Technology Authority for a summer scholarship (09-EPSCoR-0072) grant for financial support. PM would like to thank Dr. Pijus Kanti Roy for some critical references and valuable discussions.
Notes
Supporting information: Detail experimental data, figures and spectra that include analytical data of catalysts, solubility at different temperature and pressure, comparison of solubility of salicylic acid, recyclability test, mass spectrum of 1c, apparatus for solubility measurement, validity of solubility method, inertness test of catalyst to CO2, a representative plot for measurement of δ and plot of turn over frequency of different catalysts are given in supporting information.
References
- Munshi , P. ; Main , D.A. ; Linehan , J.C. ; Tai , C.C. ; Jessop , P.G. J. Am. Chem. Soc . 2002 , 124 , 7663 7671 .
- Munshi , P. ; Beckman , E.J. Ind. Eng. Chem. Res . 2009 , 48 , 1059 1062 .
- Darr , J.A. ; Poliakoffs , M. Chem. Rev . 1999 , 99 , 495 541 .
- Munshi , P. ; Bhaduri , S. Curr. Sci . 2009 , 97 , 63 72 .
- Beckman , E.J. J. Supercrit. Fluids 2004 , 28 , 121 191 .
- Leitner , W. ; Jessop , P.G . Chemical Synthesis Using Supercritical Fluids ; Wiley-VCH , Verleg , 1999 .
- Cowey , C.M. ; Bartle , K.D. ; Burford , M.D. ; Clifford , A.A. ; Zhu , S. J. Chem. Eng. Data 1995 , 40 , 1217 1221 .
- DeSimone , J.M. ; Guan , Z. ; Elsbernd , C.S. Science 1992 , 257 , 945 947 .
- Hoefling , T.A. ; Newman , D.A. ; Enic , R.M. ; Beckman , E.J. J. Supercrit. Fluids 1993 , 6 , 165 171 .
- Sarbu , T. ; Styranec , T. ; Beckman , E.J. Nature 2000 , 405 , 165 168 .
- Jessop P.G. Heldebrant D.J. Li X. Eckert C.A. Liotta C.L. Nature 2005 436 1102
- Liu , Y. , Jessop , P.G. , Cunningham , M. , Eckert , C.A. and Liotta , C.L. 2006 . Science , 313 : 958 – 960 .
- Wegner A. Leitner W. Chem. Commun 1999 1583 1584
- Leitner , W. Pure Appl. Chem . 2004 , 76 , 635 644 .
- Ablan , C.D. ; Sheppard , D. ; Beckman , E.J. ; Olmstead , M.M. ; Jessop , P.G. Green Chem . 2005 , 7 , 590 594 .
- Yajdi , A.V. ; Beckman , E.J. Ind. Eng. Chem. Res . 1997 , 36 , 2368 2374 .
- Guironnet , D. ; Schnetmann , I.G. ; Mecking , S. Macromolecules 2009 . doi: DOI: 10,1021/ma901397q .
- Patel , Y. ; George , J. ; Pillai , S.M. ; Munshi , P. Green Chem . 2009 , 11 , 1056 1060 .
- Davies A.G. Organotin Chemistry , 2nd ed Weinheim : Wiley-VCH , 2004 .
- Omae , I. Organotin Chemistry ; Elsevier : New York , 1989 .
- Ingham , R.K. ; Rosenberg , S.D. ; Gilmn , H. Chem. Rev . 1960 , 60 , 459 539 .
- Otera , J. ; Danoh , N. ; Nozaki , H. J. Org. Chem . 1991 , 56 , 5307 5311 .
- Harrison , P.G. ; Molloy , K. J. Organomet. Chem . 1978 , 152 , 63 72 .
- Lu , Y. ; Weng , L.H. ; Lan , Z.L. ; Li , J. ; Xie , Q.L. Chin. Chem. Lett . 2002 , 13 , 185 188 .
- Fernelius , W.C. ; Bryant B.E. Inorganic Syntheses ; New York : McGraw-Hill , 1957 ; 105 113 .
- Wada , M. ; Nishino , M. ; Okawara , R. J. Organomet. Chem . 1965 , 3 , 70 75 .
- Tkatchenko , D.B. ; Chambrey , S. ; Keiski , R. ; Ligabue , R. ; Plasseraud , L. ; Richard , P. ; Turunen , H. Catal. Today 2006 , 115 , 80 86 .
- Ballivet-Tkatchenko , D. ; Ligabue , R.A. ; Plasseraud , L. Braz. J. Chem. Eng . 2006 , 23 , 111 116 .
- Lee , S.H. ; Lee , S.B. Chem. Commun . 2005 , 3469 3471 .
- Ruelle , P. ; Farina-Cuendet , A. ; Kesselring , U.W. J. Am. Chem. Soc . 1996 , 118 , 1777 1784 .
- Alessi , P. ; Cortesi , A. ; Kikic , I. ; Foster , N.R. ; Macnaughton , S.J. ; Colombo , I. Ind. Eng. Chem. Res . 1996 , 35 , 4718 4726 .
- Aschenbrenner , O. ; Kemper , S. ; Dahmen , N. ; Schaber , K. ; Dinjus , E. J. Supercrit. Fluids 2007 , 41 , 179 186 .
- Lagalante , A.F. ; Hansen , B. ; Bruno , T.J. Inorg. Chem . 1995 , 34 , 5781 5785 .
- Barton , A.F.M. Chem. Rev . 1975 , 75 , 731 754 .
- Harrison , K. ; Goveas , J. ; Johnston , K.P. ; O'Rear , E.A. III . Langmuir 1994 , 10 , 3536 3541 .
- Hildebrand , J.H. J. Am. Chem. Soc . 1919 , 41 , 1067 1080 .
- Fukuda , H. ; Kondo , A. ; Noda , H. J. Biosci. Bioeng . 2001 , 92 , 405 416 .
- Narasimharao , K. ; Lee , A. ; Wilson , K. J. Biobased Mater. Bioenergy 2007 , 1 , 19 30 .
- Freedman , B. ; Butterfield , R.O. ; Pryde , E.H. J. Am. Oil Chem. Soc . 1986 , 63 , 1375 1380 .
- Noureddini , H. ; Zhu , D. J. Am. Oil Chem. Soc . 1997 , 74 , 1457 1463 .
- Shimada , Y. ; Watanabe , Y. ; Samukawa , T. ; Sugihara , A. ; Noda , H. ; Fukuda , H. ; Tominaga , Y. J. Am. Oil Chem. Soc . 1999 , 76 , 789 793 .
- Jousseaume , B. ; Laporte , C. ; Rascle , M-C. ; Toupance , T. Chem. Commun . 2003 , 1428 1429 .
- George , J. ; Patel , Y. ; Pillai , S.M. ; Munshi , P. J. Mol. Catal. A 2009 , 304 , 1 7
- Eckert , C.A. ; Knutson , B.L. ; Debenedetti , P.G. Nature 1996 , 383 , 313 318 .
- Jessop , P.G. ; Subramaniam , B. Chem. Rev . 2007 , 107 , 2666 2694 .
- Song , J. ; Hou , M. ; Jiang , T. ; Han , B. ; Li , X. ; Liu , G. ; Yang , G. J. Phys. Chem. A 2007 , 111 , 12007 12010 .
- Dandge , D.K. ; Heller , J.P. ; Wilson , K.V. Ind. Eng. Chem. Prod. Res. Dev . 1985 , 24 , 162 166 .
- Macnaughton , S.J. ; Kikic , I. ; Rovedo , G. ; Foster , N.R. ; Alessi , P. J. Chem. Eng. Data 1995 , 40 , 593 597 .
- Gurdial , G.S. ; Foster , N.R. Ind. Eng. Chem. Res . 1991 , 30 , 575 580 .
- Tkatchenko , D.B. ; Chambrey , S. ; Keiski , R. ; Ligabue , R. ; Plasseraud , L. ; Richard , L.P. ; Turunen , H. Catal. Today 2006 , 115 , 80 86 .