ABSTRACT
The biosynthesis of silver nanoparticles (Ag-NPs) using the hydrosol extract of the dry stem bark of Acacia mearnsii as reducing and capping agents, and their antinociceptive properties are hereby reported. By varying the temperature and reaction time, the temporal evolution of the optical and morphological properties of the as-synthesized material was investigated. The NPs were characterized by UV–visible absorption spectroscopy, transmission electron microscopy (TEM), Fourier transform infrared spectroscopy (FTIR), scanning electron microscopy (SEM), energy-dispersive x-ray spectroscopy (EDS) and x-ray diffractometry (XRD) The optical analyses show that the position of the maximum surface plasmon resonance (SPR) peak is red-shifted as the reaction temperature decreased. The TEM micrographs show that the as-synthesized Ag-NPs are spherical while the X-ray diffraction shows that the material is highly crystalline with face-centered cubic structures. The anti-inflammatory efficacy, analyzed by the formalin model, indicates that the as-synthesized Ag-NPs are very effective, with an inhibition rate of about 76%.
GRAPHICAL ABSTRACT
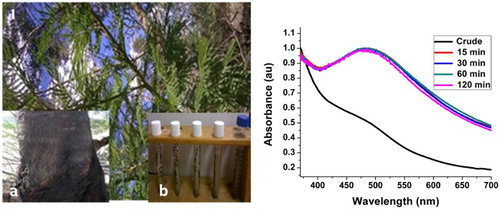
1. Introduction
Nanotechnology has become an interdisciplinary field of research that is growing rapidly with integration into other areas of science and technology (Citation1). In this technology, nanoparticles (NPs) are extremely important because of their exceptionally small size and therefore exceptionally large ratio of surface area to volume. This determines their mechanical, physical, chemical, electrical, optical, solubility and stability properties (Citation2,Citation3). Among the various metal NPs like copper, titanium, zinc, iron and gold, silver nanoparticles (Ag-NPs) have become desirable as a result of their outstanding surface plasmonic resonance (SPR) and their potent biological activities such as antimicrobial, antibacterial, anti-inflammatory, anticancer, antitumor and antimalarial activities (Citation4–9).
Several methods have been employed for synthesizing NPs; these include reverse phase micelles, chemical reduction, electrochemical, sono-electrochemical and laser ablation to mention a few. Among these methods, chemical reduction offers a simple method of synthesis which is achieved by reduction of the metal ion salt in solution. The method employs several organic reagents such as sodium borohydride (NaBH4), hexadecylamine (HDA), tri-n-octylphosphine (TOP), ethylene glycol, poly(vinyl pyrrolidine) (PVP) as the reaction solvent, reducing agent and capping agent (Citation10–14). However, these reagents/solvents are toxic, expensive, environmentally unfriendly and produce by-products that are toxic to both humans and the environment. To circumvent this problem, greener methods involving the use of plants, micro-organisms and marine organisms have been reported.
These methods have been proved to be effective, simple, inexpensive, environmental friendly and yield stable size-controlled Ag-NPs. The ability of plant and micro-organisms to function in phytomining and phytoremediation of heavy metals further enhances their potential for the biosynthesis of Ag-NPs (Citation15). It has been reported that certain magnetostatic bacteria can synthesize magnetic NPs (Citation16). Gardea-Torresdey et al. reported the reduction of metallic solution using alfalfa sprout. In this work, Au and Ag NPs between 6 and 10 nm with face-centered cubic (fcc) crystalline structure were produced (Citation17–18). In another development, Huang et al. successfully prepared triangular and spherical Ag-NPs from the biomass of Cinnamomum camphora at ambient temperature by controlling the amount of biomass used (Citation19). In addition to acting as reducing agent, the plant materials can also act as stabilizing agent. Bar et al. reported the synthesis of Ag-NPs using Jatropa curcas latex. The stability of the material was attributed to cyclic peptides present in the latex (Citation20). In another study, Velmurugan et al. reported the synthesis of 10–20 nm Ag-NPs from the root extract of Zingiber officinale. The as-synthesized Ag-NPs showed high antibacterial efficacy against Staphylococcus spp. and Listeria spp. The stability and high antibacterial property of the material was attributed to the heterocyclic moieties present in the alkaloids and flavonoids as the major constituents of Zingiber officinale root (Citation21).
Acacia mearnsii De Wild is a genus of the Mimosoideae family, commonly known as black wattle, blue passionflower, green wattle and tan wattle. It originates from the Australian continent, and has been spread to other continents, including America, Asia and Africa. The economic importance of Acacia mearnsii as a source of tannins led to its cultivation in South Africa (Citation22). The therapeutic uses of proanthocyanidins from Acacia mearnsii include anti-obesity, anti-diabetes (Citation23), inhibition of the enzymes α-amylase and lipase, and itching control (Citation24). Anti-inflammatory studies revealed that the extracts of Acacia mearnsii inhibit inflammatory mediators at some dose levels (Citation25–27). In addition, the phytochemical analysis of the plant shows a high content of tannins as reported by Kusano et al., who isolated some proanthocyanidin oligomers such as 5-deoxyflavan-3-ols, 4’-O-methylrobinetididol, 3’-O-β-D-glucopyranoside, syringic acid, butin, gallocatechin and catechin from the plant (Citation28). The essential oils from this plant are composed mainly of phytol and cis-verbeneol, with high anti-inflammatory activity (Citation29). In this report, we explore the synthesis of Ag-NPs using the hydrosol extract of the stem bark of Acacia mearnsii as both reducing and capping agents. The effect of the sequential evolution of the reaction temperature and time on the optical and morphological properties was investigated while the antinociceptive properties of the as-synthesized Ag-NPs were analyzed with the formalin model using Swiss mice.
2. Materials and methods
2.1. Plant material
The stem bark of Acacia mearnsii (; inset (stem bark)) was collected at the botanical garden of Walter Sisulu University, Mthatha, Eastern Cape, South Africa (31o36’08.35’S, 28o45’02.48’E). The plant was taxonomically identified by Mr. T. Dold, at the Selmar Schonland Herbarium at Rhodes University in Grahamstown (GRA), South Africa. A voucher number AOM001 was assigned and collected, and a voucher specimen deposited in the herbarium for future reference.
2.2. Chemicals and reagents
Silver nitrate (AgNO3) was purchased from Sigma-Aldrich and used as purchased. The plant extract served as the reducing and passivating agent.
2.3. Preparation of hydrosol extract of Acacia mearnsii (AM)
Three hundred grams of the dried stem bark of each sample were hydro-distilled in a Clevenger-type apparatus for 3 h at 100°C. Upon completion of the hydro-distillation, the plant residue in the flask was allowed to cool, followed by filtration using 250 µm mesh sizes. The extract was kept in an amber bottle, stored at 4°C and analyzed within one week, before any mucus could grow on it.
2.4. Synthesis of Acacia mearnsii dry stem extract capped silver nanopaerticles (AMDS)-Ag-NPs
An aliquot (50 mL) of the Acacia mearnsii extract was added to a three-necked flask, already equipped with a temperature sensor and placed in a jacketed heating mantle. 10 mL of a 0.1 mol/L AgNO3 aqueous solution was transferred into the flask containing the plant extract with constant stirring at room temperature, 40°C and 60°C. The reaction vessel was covered with an aluminum foil and placed in a dark cupboard to avoid auto-reduction of the AgNO3 because of its photosensitivity (Citation30). The appearance of a milky color after few minutes of reaction indicates formation of the Ag-NPs as shown in (inset). Aliquots were withdrawn at different time intervals to monitor the growth of the Ag-NPs.
2.5. Characterization
The UV–visible spectra were recorded using a UV 1650 PC-Shimadzu B UV–visible spectrophotometer (Shimadzu, Osaka, Japan). Transmission electron microscopy (TEM) analysis was carried out using a JEOL JEM 2100 (TEM) operated at 200 KV. Samples were mounted on a carbon-coated copper grid and then air dried. The crystallinity of the Ag-NPs was examined by a Bruker aXS D8 advanced diffractometer. The XRD patterns were recorded at a scan speed of 4°/min with Cu Kα radiation (λ = 1.5406 Å) operated at 40 KV and 40 mA. SEM analysis was performed using a Philips XL-30 instrument (Philips, Eindhoven), while EDXRF was carried out on a DX-700HS spectrometer (Shimadzu) The FTIR spectra were recorded over the range of 370–4000 cm−1 using an FTIR spectrometer equipped with the universal ATR sampling accessory. A small volume of the liquid sample was concentrated via air drying before analysis.
2.6. Analgesic and inflammation analysis
2.6.1. Methods for the formalin test
The method of Prabhu et al. was used for the formalin test with slight modification (Citation31). Four groups of Swiss mice (n = 6) were selected for the study. Group 1, the control group, was treated with 0.09% NaCl. Group 2, the positive control group, was treated with Aspirin (100 mg/kg). Group 3, the AMDS test group, was treated with the Acacia mearnsii dry stem extract (AMDS) (200 mg/kg). Group 4, the AMDS-Ag-NPs test group, was treated with the as-synthesized extract capped Ag-NPs (AMDS-Ag-NPs) (200 mg/kg). This is summarized in .
Table 1. Experimental method.
One hour (1 h) after treatment with the various test and control materials, animals were injected sub-plantally with 100 μL of 2.5% formalin solution diluted in saline. Swiss mice were used because of their high sensitivity to pain. The noniceptive response in Swiss mice was characterized by an intense period of biting and licking of the rat paw for 5 min after injection of diluted formalin to the hind paw. The biting and licking subsided for 15 min (rest period) and later commenced with periodic bites and licks of the hind paw for 20–30 min. Concurrent counts of bites and licks were recorded for the neurogenic phase (phase 1) and anti-inflammatory phases (phase 2) The percentage inhibition was then calculated using the following formula:(1) where T is the number of times treated mice licked/bit the injected paw; C is the number of times control mice licked/bit the treated paw.
2.6.2. Statistical analysis
Results were expressed as mean ± SEM. Statistical analyses were carried out using one-way analysis of variance (ANOVA) followed by Dunnett’s post hoc test, and values were considered significant at p < .05.
3. Results and discussion
3.1. Optical characterization
3.1.1. UV–visible spectroscopic analysis
In this reaction, the hydrosol extract of A. mearnsii acted as both reducing and stabilizing agents. Preliminary observation of color change from deep brown to milky color within 15 min of reaction confirmed the reduction of AgNO3 to Ag-NPs ((A) inset) The whole process is a redox reaction with silver nitrate acting as the oxidant and Ag-NPs are the reduction product, while the phytochemicals in the plant extracts act as the reducing and passivating agent. Based on our earlier reports (Citation29), this plant contains considerable amounts of monoterpenoids, sesquiterpenes and phytols. The OH of the phytol group reduced the Ag+ ions to Ag0 while the amide group of the monoterpenoids and sesquiterpene passivated and stabilized the NPs. The surface plasmon resonance (SPR) peak corresponding to the excitation of longitudinal plasmon vibrations of Ag-NPs was observed at 480 nm. (B) depicts the SPR spectra of the as-synthesized AM-AgNPs at a temperature of 60°C. The position of the SPR peak remains the same after 15 min and for the rest of the reaction time. The absence of any significant change in the SPR peak position and the shape of the spectra after 15 min is an indication that the particle size and size distribution remain the same throughout the reaction time. This shows that Acacia mearnsii extract reduction of AgNO3 to Ag-NPs is a very fast reaction. Since all the AgNO3 were reduced within 15 min, the reaction can be stopped after 15 min under this synthetic condition.
At 40°C reaction temperature ((A)), the position of the SPR peak is red-shifted to 490 nm with broadening compared to the Ag-NPs synthesized at 60°C. This indicates formation of larger particles with broad size distribution. However, the growth kinetics follow the same pattern as growth kinetics at 60°C, i.e. the position of the SPR peak and the shape of the spectrum remain the same after 15 min reaction time. The room temperature reaction did not show any distinctive SPR peak ((B)). The spectra show resemblance to that of the plant extract indicating that there was no reduction at this temperature. These results show that the use of Acacia mearnsii extracts as reducing and stabling agents is temperature dependent. The smaller particle size and narrow size distribution at 60°C can be attributed to the high concentration of phytochemicals released at this temperature which led to faster reduction and more effective passivation. Dai and Munper reported that Acacia mearnsii contains phytochemicals embedded in the cell cavity of the plant, which can be released at high temperatures (Citation1).
3.1.2. Fourier transform infrared spectroscopy
To determine the probable bio-reducing functional groups liable for the reduction and passivation of the Ag-NPs’ surface under this synthetic method, FTIR analysis was performed. shows the spectra of the crude material and that of the as-synthesized AM-Ag-NPs. Characteristic changes in the position, emergence of new peaks and change in the peak intensities are some confirmatory parameters for the biomolecules responsible for the reduction and successful capping of the as-synthesized Ag-NPs. The changes and assignments of the peaks are outlined in . Representative functional groups of some biomolecules such as proteins, sesquiterpenes, phytols, flavonoids, alkaloids and terpenoids were observed from the crude extract. The free O–H of alcohols and phenols, C=O of the amide group of proteins, C–N of the amines, alkaloids, N–O of nitro-containing terpenoids, –OCH3 of flavonols and halogen peaks were identified. In the as-synthesized AM-Ag-NPs, the broadness of the peak increases and the peaks assigned to the free O-H stretching vibration shifted from 3715.8 to 3855.5 cm−1. In addition, the C=O of the amide which appeared at 1619.3 cm−1 in the crude extract was shifted to 1608.4 cm−1. Furthermore, the peaks at 1452.7, 1320.1, 1204.0 and 1154.9 cm−1 assigned to the C=C of aromatics, C–N of amides, C–N of amines and C–O of ester stretching vibrations in the AM extract shifted to 1440.06, 1396.95, 1278.89, 1192.51, respectively. The red shifting of the amide, amine and ester C–O stretching vibration in comparison with the crude extract indicates the involvement of the NH and the proton of the C–O containing compounds in the reduction of Ag ion to Ag-NPs and its subsequent capping. However, the blue shifting of the C=C aromatic bond indicates the interaction between the aromatic group of the flavonols of the extract with the Ag-NPs’ surface. Other peaks at 1030.1 and 523.1 cm−1 are attributed to the presence of ether linkage and bending vibration found in both the extract and the as-synthesized Ag-NPs. This further confirmed the reduction and capping of the Ag-NPs by the plant extract. The previously observed peak at 2124.6 cm−1, earlier attributed to the C°C triple bond stretching vibrations in the AM extract, disappeared with the emergence of a new peak at 2856.7 cm−1 assigned to the C–H stretching of alkyl groups. Thus, the bio-reduction and stabilization of the as-synthesized Ag-NPs could be attributed to the presence of nerolidol, phytol, flavonols, verbenone and monoterpenoid functional groups present in the plant extract and the synergistic effect of other functional groups earlier highlighted. Earlier reports had indicated the ability of the protein moiety of amines, amides and peptides, to bind to nanoparticle surfaces, thereby acting as capping agents preventing aggregation (Citation32–34).
Table 2. FTIR analysis of the crude extract and the as-synthesized AM-AgNPs.
3.2. Morphological studies
3.2.1. SEM and EDS studies
The morphology of the as-synthesized Ag-NPs investigated using scanning electron microscopy (SEM) is shown in . The micrographs show that the surface of the crude extracts is coarse while that of the as-synthesized Ag-NPs shows little follicles ((B)) The formation of Ag-NPs and their purity was further confirmed using EDS () The EDS spectrum of the crude extract ((A)) shows C and O as the major peaks with traces of Cl, N and S. However, the Ag-peak is conspicuously absent. On the other hand, the EDS spectrum of the as-synthesized Ag-NPs ((B)) shows the Ag peak as the major peak with traces of Cl, C, O, N and S. The weight percentage of the Ag, Cl, C, O, N and S are 75.4%, 11.1%, 0.9%, 12.5%, 1.0% and 0.1%, respectively. This further confirms the formation and the purity of the as-synthesized Ag-NPs.
3.2.2. XRD and TEM analyses
The crystallinity of the as-synthesized Ag-NPs was confirmed using XRD analysis. (A) shows the typical XRD pattern of the Ag-NPs synthesized at 40 and 60°C. The XRD pattern shows diffraction peaks at 2θ values of 38.63, 46.54, 64.97, 77.42 and 81.99, respectively, corresponding to the (111), (200), (220), (311) and (222) crystallographic planes of the face-centered cubic crystalline structures of metallic Ag (JCPDS no 04-0783). The broad nature of the XRD peaks is attributed to the nano-crystalline nature of the Ag-NPs. The unassigned peaks may result from the bio crystalline particles of the plant extract (Citation1). The particle sizes as calculated using the Debye–Sherrer’s formula (Citation44) are 20.77 and 24.91 nm for Ag-NPs synthesized at 60°C and 40°C, respectively. These results are in alignment with the optical analyses. The typical TEM micrograph of the as-synthesized AM-Ag-NPs at 60°C ((B)) shows that the material is spherical in shape while the presence of clear singular reciprocal points in the fast Fourier transform (FFT) patterns ((C)) further confirmed the high crystallinity of the as-synthesized material indicated by the XRD analysis. The particle diameter, as determined from the TEM images using the size distribution curve ((D)), is in the range 5–60 nm with an average particle size of 19.95 ± 7.76 nm, in alignment with calculated values and optical data.
3.3. Antinociceptive and inflammation activity
The formalin-induced test is a pain model used to analyze both the analgesic and anti-inflammatory properties simultaneously. Formalin induces pain of moderate intensity characterized by irritation, tissue damage and edema formation due to the release of inflammatory mediators (Citation45). Typically, formalin-induced pain involves two phases. The initial phase is linked to the stimulation of nociceptors and principal afferent fibers by the formalin, triggering the liberation of bradykinin and tachykinins which occurs for 5 min (Citation46). The latter phase, which lasts for 20–30 min after injection, is accompanied by the discharge of inflammation facilitators such as prostaglandins, cytokines, histamine, nitric oxide (NO) and serotonin. In addition, these phases are inhibited by different classes of drugs. Opioid drugs inhibit the early phase and non-steroidal, anti-inflammatory drugs (NSAIDs) and opioid drugs inhibit the latter phase. shows the antinociceptive effect of oral treatment with AMDS-Ag-NPs on formalin-induced pain. In this model, a lower number of licks is an indication of high inhibition. As expected, aspirin used as the standard drug significantly inhibited the first phase (83%) and second phase (73%) of the test which is expected for a non-steroidal drug. The crude sample (AMDS) and the AMDS-Ag-NPs exhibited high activity at the inflammatory phase with 70% and 76% inhibition, respectively, at a dose level of 200 mg/kg. Ag-NPs had been shown to possess very high wound healing and anti-inflammation effects due to their high rate of absorption into cell membranes and ability to modulate cytokine production (Citation47–49). The activity of the as-synthesized Ag-NPs was significant to an order of p > .01 at both phases and the result shows that the NPs possess better activity than the crude plant at the second phase only. In addition, the anti-inflammatory activity of the as-synthesized Ag-NPs at a dosage of 200 mg/kg is almost similar to 100 mg/kg dose level of the standard drug (Aspirin) with a significance level of p < .01. This indicates that the as-synthesized AMDS-Ag-NPs can be used as a drug to modulate various inflammatory mediators.
Table 3. Antinociceptive effect of oral treatment with AMDS-AgNPs on formalin-induced pain.
4. Conclusion
Ag-NPs were successfully synthesized via bio-reduction of silver nitrate solutions using the stem bark hydrosol of Acacia mearnsii. The plant extract acted as both reducing and capping agents while the optimization of the synthetic parameters shows 60°C as the optimum temperature for the synthesis. Furthermore, the reduction reaction using the Acacia mearnsii extract is very fast, and can be completed within 15 min. The morphological analyses show that the as-synthesized AM-Ag-NPs are spherical in shape with face-centered cubic (fcc) crystalline structure while the FTIR analyses showed that the hydroxyl, alkyne, carboxyl and amide groups of the monoterpenoids, sesquiterpenes and phytols present in the Acacia mearnsii extract were liable for the bio-reduction of the silver ions to NPs and the consequent passivation of the surface. The anti-inflammatory efficacy, analyzed by the formalin model, reveals that the as-synthesized Ag-NPs were more effective than the crude extract at the second phase (inflammation phase) with an inhibition that is close to that of the standard drug, Aspirin. This investigation shows that Ag-NPs synthesized from the stem bark of Acacia mearnsii can be used as a drug to modulate various inflammatory mediators such as cytokines, prostaglandins, NO, histamine and serotonin.
Acknowledgement
The authors are grateful to Govan Mbeki Research office, University of Fort Hare, Directorate of Research, Walter Sisulu University.
Disclosure statement
No potential conflict of interest was reported by the authors and University of Johannesburg.
Notes on contributors
Dr. Avoseh Opeyemi Nudewhenu earned his PhD degree in Organic/Natural product chemistry from University of Fort Hare, South Africa, where he worked on Isolation, Characterisation of Terpenoids and Biosynthesis of Silver Nanoparticles of Acacia Mearnsii (De Wild) and Acacia Karroo (Hayne) and on their bioassays with Prof. O.O. Oyedeji. He is currently a Lecturer II in Lagos State University, Lagos-Nigeria. His research is centred on Natural Products, Semi-synthesis and Material chemistry.
Opeoluwa O Oyedeji is an associate professor of organic chemistry and currently Head of Department of Chemistry at University of Fort Hare. His research areas of interest include isolation of bioactive compounds and semi-synthesis for enhanced activity. He graduated from University of KwaZulu Natal, South Africa with a PhD degree in 2010.
Olukayode Aremu is a dynamic, highly ambitious and hardworking student doing pain and cardiovascular Physiology. He is very active and involved in all ongoing projects in the research laboratory such as pain and inflammation research, toxicity profile of various plant materials, antioxidant and antihypertensive effects of various indigenous medicinal plants. He also guides younger students with their research projects. He holds a Masters’ degree in the department of Physiology, Faculty of Health Sciences in Walter Sisulu University in 2016. Moving forward, he is furthering his Doctorate program where he is exploring the ventricular remodelling in rheumatic heart diseased patients in Cape Universities using cardiac magnetic resonance imaging.
Benedicta N Nkeh-Chungag is a Professor of Physiology at the Walter Sisulu University, Eastern Cape Province. She received her PhD from the Physiology Department, University of the Witwatersrand, Johannesburg and later a Master’s Degree in Public Health from the Walter Sisulu University. She has been teaching Physiology at the tertiary level for over 18 years and has supervised both undergraduate and postgraduate research projects in this field. She serves on the South African National Committee for the International Union of Physiological Sciences and as a reviewer for several scientific journals. She is a member of several knowledge societies such as the American Heart Association and the Physiological Society of Southern Africa. She has published over 50 peer-reviewed articles and gave over 50 presentations at both local and international conferences.
Prof. Sandile P. Songca currently serves at the University of Zululand as the deputy vice chancellor responsible for Teaching and Learning, and continues to serve as a member of the board of the Eastern Cape Information Technology Initiative. He earned a doctor of philosophy degree from Queen Mary and Westfield College, University of London, in organic chemistry. His main research interests include spectroscopic analytical techniques, teaching and learning innovations, synthesis and evaluation of amphiphilic porphyrins for use in photodynamic therapy, fabrication, functionalization and characterization of nanomaterials, medicinal plants and human geophagia. He has attracted several research grants, so far in excess of R50M. I have read more than 70 conference papers and published more than 50 articles in both local and international Journals and books. In addition, he has one patent pending in medicinal plants and a license in nanotechnology. He has supervised more than 25 honours, masters and PhD students, and currently supervising 8 PhD and six MSc students. I have lectured at 5 universities, from 1983 until 2008.
Adebola Oyedeji is professor of organic chemistry at Walter Sisulu University, Mthatha campus. She heads a natural product research group which researches into isolation and characterization of terpenes compounds for medicinal and cosmetic end uses. She obtained her PhD degree from University of Ibadan, Nigeria in 2001.
Dr. Sneha Mohan received her PhD in 2015 from Department of Applied chemistry, Cape Peninsula University of Technology, Cape Town, South Africa under the supervision of Prof. SO Oluwafemi. She is working currently as Research Associate at the University of Johannesburg, South Africa. Her research interest includes synthesis of various nanomaterials, polymer composites and their applications in Energy, water treatment and biomedical fields. She has published 12 journal articles, 3 book chapters and 1 book to her credit.
Prof. Oluwatobi S. Oluwafemi is a National Research Foundation (NRF), South Africa rated researcher serving at the department of Applied Chemistry, University of Johannesburg. His research is in the broad area of nanotechnology and include green synthesis of semiconductor and metal nanomaterials for different applications, which include but not limited to biological (Imaging, labeling, therapeutic), optical, environmental and water treatment. He has authored or co-authored many journal publications, book chapters and books. He is a reviewer for many international journals in the field of nanotechnology and has won many accolades, both local and international.
Additional information
Funding
References
- Dai, J.; Mumper, R.J. Molecules 2010, 15, 7313–7352. doi: 10.3390/molecules15107313
- AbdelHamid, A.A.; Al-Ghobashy, M.A.; Fawzy, M.; Mohamed, B.M.; Abdel-Mottaleb, M. ACS Sustain. Chem. Eng. 2013, 1, 1520–1529. doi: 10.1021/sc4000972
- Gnanajobitha, G.; Paulkumar, K.; Vanaja, M.; Rajeshkumar, S.; Malarkodi, C.; Annadurai, G.; Kannan, C. J. Nanostructure Chem. 2013, 3(1), 67. doi: 10.1186/2193-8865-3-67
- Ajitha, B.; Reddy, Y.A.K.; Reddy, P.S. Spectrochim. Acta A 2014, 128, 257–262. doi: 10.1016/j.saa.2014.02.105
- Bindhu, M.R.; Umadevi, M. Spectrochim. Acta A 2014, 128, 37–45. doi: 10.1016/j.saa.2014.02.119
- Devi, J.S.; Bhimba, B.V. Sci. Rep. 2014, 1(4), 1–5.
- Gaddala, B.; Nataru, S. Appl. Nanosci. 2014, 5(1), 99–104. doi: 10.1007/s13204-014-0295-4
- Vaseeharan, B.; Ramasamy, P.; Chen, J.C. Lett. Appl. Microbiol. 2010, 50, 352–356. doi: 10.1111/j.1472-765X.2010.02799.x
- Shanthi, S.; Jayaseelan, B.D.; Velusamy, P.; Vijayakumar, S.; Chih, C.T.; Vaseeharan, B. Microb. Pathog. 2016, 93, 70–77. doi: 10.1016/j.micpath.2016.01.014
- Oluwafemi, O.S.; Ncapayi, V.; Olubomehin, O.; Osibote, O.A.; Songca, S.P. Mater. Sci. Semicond. Process. 2014, 27, 427–432. doi: 10.1016/j.mssp.2014.07.007
- Raveendran, P.; Fu, J.; Wallen, S.I.; Hill, C.; Carolina, N. J. Am. Chem. Soc. 2003, 125, 13940–13941. doi: 10.1021/ja029267j
- Sun, Y.; Xia, Y. Science ( New York, NY) 2003, 298(5601), 2176–2179. doi: 10.1126/science.1077229
- Tran, Q.H..; Nguyen, V.Q.; Le, A.T. Adv. Nat. Sci: Nanosci. Nanotechnol. 2013, 4(3), 033001.
- Xiong, J.; Wang, Y.; Xue, Q.; Wu, X. Green Chem. 2011, 13(4), 900–904. doi: 10.1039/c0gc00772b
- Iravani, S. Green Chem. 2011, 13(10), 2638–2650. doi: 10.1039/c1gc15386b
- Dickson, D.P.E. J. Magn. Magn. Mater. 1999, 203, 46–49. doi: 10.1016/S0304-8853(99)00178-X
- Gardea-Torresdey, J.L.; Gomez, E.; Peralta-Videa, J.R.; Parsons, J.G.; Troiani, H.; Jose-Yacaman, M. Langmuir 2003, 19, 1357–1361. doi: 10.1021/la020835i
- Gardea-Torresdey, J.L.; Parsons, J.G.; Gomez, E.; Peralta-Videa, J.; Troiani, H.E.; Santiago, P.; Yacaman, M.J. Nano Lett. 2002, 4, 397–401. doi: 10.1021/nl015673+
- Huang, J.; Li, Q.; Sun, D.; Lu, Y.; Su, Y.; Yang, X.; Chen, C. Nanotechnology 2007, 18(10), 105104. doi: 10.1088/0957-4484/18/10/105104
- Bar, H.; Bhui, D.K.; Sahoo, G.P.; Sarkar, P.; De, S.P.; Misra, A. Colloids Surf. A 2009, 339, 134–139. doi: 10.1016/j.colsurfa.2009.02.008
- Velmurugan, P.; Anbalagan, K.; Manosathyadevan, M.; Lee, K.K.; Cho, M.; Lee, S.M.; Oh, B.T. Bioprocess Biosyst. Eng. 2014, 37(10), 1935–1943. doi: 10.1007/s00449-014-1169-6
- Sherry, S.P. The effectiveness of different allelopathic substances De Wild; University of Natal Press: Pietermaritzburg, 1971.
- Ikarashi, N.; Sato, W.; Toda, T.; Ishii, M.; Ochiai, W.; Sugiyama, K. eCAM 2012, 2012, 120389.
- Ikarashi, N.; Toda, T.; Okaniwa, T.; Ito, K.; Ochiai, W.; Sugiyama, K. eCAM 2011, 2011, 952031.
- Bukhari, I.A.; Khan, R.A.; Gilani, A.H.; Ahmed, S.; Saeed, S.A. Inflammopharmacology 2010, 18, 187–196. doi: 10.1007/s10787-010-0038-4
- Burnett, B.P.; Jia, Q.; Zhao, Y.; Levy, R. J. Med. Food, 2007, 10(3), 442–451. doi:10.1089/jmf.2006.255
- Maldini, M.; Sosa, S.; Montoro, P.; Giangaspero, A.; Balick, M.J.; Pizza, C.; Loggia, R.D. J. Ethnopharmacol. 2009, 122, 430–433. doi: 10.1016/j.jep.2009.02.002
- Kusano, R.; Ogawa, S.; Matsuo, Y.; Tanaka, T.; Yazaki, Y.; Kouno, I. J. Nat. Prod. 2011, 74, 119–128. doi: 10.1021/np100372t
- Avoseh, O.; Oyedeji, O.; Aremu, K.; Nkeh-Chungag, B.; Songca, S.; Oluwafemi, S.O.; Oyedeji, A. Nat. Prod. Res. 2015, 29(12), 1184–1188. doi: 10.1080/14786419.2014.983504
- Babaahmadi, V.; Montazer, M.; Toliyat, T.; Ghanbarafjeh, M. CTAB Proc. NAP 2011, 1, 183–190.
- Prabhu, V.V.; Nalini, G.; Chidambaranathan, N.; Kisan, S.S. Int. J. Pharm. Pharm. Sci. 2011, 3(2), 1–4.
- Mohan, Y.M.; Raju, K.M.; Sambasivudu, K.; Singh, S.; Sreedhar, B. J. Appl. Polym. Sci. 2007, 106, 3375–3381. doi: 10.1002/app.26979
- Takatsuji, Y.; Ikeno, S.; Haruyama, T. Sensors 2012, 12(4), 4952–4961. doi: 10.3390/s120404952
- Wu, R.H.; Nguyen, T.P.; Marquart, G.W.; Miesen, T.J.; Mau, T.; Mackiewicz, M.R. Molecules 2014, 19(5), 6754–6775. doi: 10.3390/molecules19056754
- Jana, B.; Mondal, G.; Biswas, A.; Chakraborty, I.; Ghosh, S. RSC Adv. 2013, 3(22), 8215–8219. doi: 10.1039/c3ra41068d
- Asha, V.; Jeeva, S.; Paulraj, K. J. Chem. Pharm. Res. 2014, 6(7), 2083–2088.
- Vanaja, M.; Gnanajobitha, G.; Paulkumar, K.; Rajeshkumar, S.; Malarkodi, C.; Annadurai, G. J. Nano Chem. 2013, 3(1), 17. doi: 10.1186/2193-8865-3-17
- Ghosh, S.; Patil, S.; Ahire, M.; Kitture, R.; Kale, S.; Pardesi, K.; Cameotra, S.; Bellare, J.; Dhavale, D.; Jadgunde, A.; Chopade, B. Int. J. Nanomed. 2012, 7, 483–496.
- Shameli, K.; Bin Ahmad, M.; Jaffar Al-Mulla, E.A.; Ibrahim, N.A.; Shabanzadeh, P.; Rustaiyan, A.; Zidan, M. Molecules 2012, 17(7), 8506–8517. doi: 10.3390/molecules17078506
- Singho, N.D.; Johan, M.R.; Lah, N.A. Nanoscale Res. Lett. 2014, 9(1), 42. doi: 10.1186/1556-276X-9-42
- Giri, N.; Natarajan, R.K.; Gunasekaran, S.; Shreemathi, S. Arch. Appl. Sci. Res. 2011, 3(5), 624–630.
- Li, J.; Yu, K.; Qian, K.; Cao, H.; Lu, X.; Sun, J. Nanoscale Res. Lett. 2014, 9(1), 172. doi: 10.1186/1556-276X-9-172
- Kumar, H.; Rani, R. Int. J. Eng. Innov. Tech. 2013, 3(3), 344–348.
- Panneerselvam, C.; Ponarulselvam, S.; Murugan, K. Arch. Appl. Sci. Res. 2011, 3(6), 208–217.
- Hunskaar, S.; Hole, K. Pain 1987, 1, 103–114. doi: 10.1016/0304-3959(87)90088-1
- Correa, C.R.; Calixto, J.B. Br. J. Pharmacol. 1993, 49, 193–198. doi: 10.1111/j.1476-5381.1993.tb13791.x
- Habiboallah, G.; Mahdi, Z.; Majid, Z.; Nasroallah, S. Mod. Res. Inflamm. 2014, 3, 128–138. doi: 10.4236/mri.2014.33016
- Hebeish, A.; El-Rafie, M.H.; El-Sheikh, M.; Seleem, A.; El-Naggar, M.E. Int. J. Biol. Macromolecules 2014, 65, 509–515. doi:10.1016/j.ijbiomac.2014.01.071
- Wong, K.K.; Cheung, S.O.; Huang, L.; Niu, J.; Tao, C.; Chi-Ming, C.; Tam, P.T. Chem. Med. Chem. 2009, 4, 1129–1135. doi: 10.1002/cmdc.200900049