ABSTRACT
TiO2 has received tremendous attention owing to its potential applications in the field of photocatalysis for solar fuel production and environmental remediation. This review mainly describes various modification strategies and potential applications of TiO2 in efficient photocatalysis. In past few years, various strategies have been developed to improve the photocatalytic performance of TiO2, including noble metal deposition, elemental doping, inorganic acids modification, heterojunctions with other semiconductors, dye sensitization and metal ion implantation. The enhanced photocatalytic activities of TiO2-based material for CO2 conversion, water splitting and pollutants degradation are highlighted in this review.
GRAPHICAL ABSTRACT
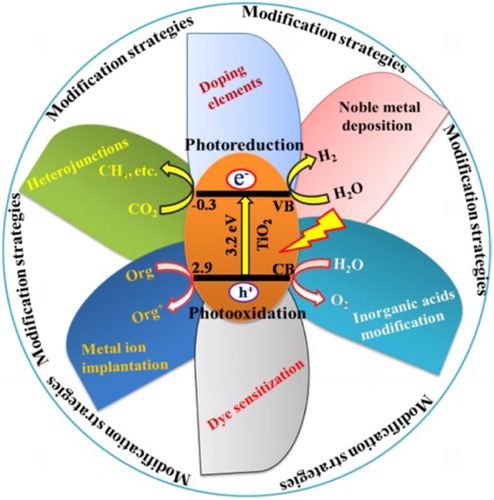
1. Introduction
Semiconductor photocatalytic technology has attracted tremendous attention for effective utilization of solar energy in water reduction to produce hydrogen, carbon dioxide conversion to chemical fuels and pollutant degradation for environmental remediation ( Citation1). In past few years, TiO2 has been extensively studied as a potential photocatalyst for CO2 conversion, water splitting and degradation of hazardous contaminants in both aqueous and gaseous system owing to its low cost, high stability, non-toxicity, biologically inertness, redox ability and environmental friendly merits ( Citation2–6). Naturally, TiO2 exists in three polymorphs such as rutile, anatase and brookite ( Citation7). Among these, anatase phase has been proven to be the most efficient photocatalyst, owing to its high photoactivity, cheapness, stability and negative conduction band potential ( Citation8). It is widely investigated in photocatalysis and as electrode material ( Citation9). Due to the high stability and redox properties of anatase TiO2, it has been used in various fields, such as solar cells, water and air purification, cancer therapy and self-cleaning of antibacterial materials ( Citation10,Citation11). The presence of high-density surface hydroxyl groups slow down the recombination of photogenerated charge carriers. The highly specific surface area and anatase crystallinity greatly influence the photocatalytic performance of TiO2. It is widely accepted that photocatalytic reactions mainly take place on the surface of photocatalysts and the adsorption of pollutants in advance is one of the most important steps in photocatalytic reactions. Thus, the large surface area is generally favorable to enhance photocatalytic activity during photocatalysis ( Citation12,Citation13). However, the large intrinsic band gap (Eg = 3.2 eV), fast recombination of photogenerated charges and limited utilization of solar-light (ca. 4%) greatly limits the practical applications of anatase TiO2. Further, at high temperature (500–600°C) treatment, anatase phase transforms into rutile phase and resulting in the decrease photocatalytic activities under normal conditions ( Citation14). To overcome the above-mentioned shortfalls, extensive research is underway to develop new routes to modify TiO2 by doping metal and non-metal elements, coupling other semiconductor materials and surface modification with inorganic acids ( Citation15–17). It has been investigated that doping elements such as S, C, N and B could greatly extend the absorption capacity of TiO2 from UV to visible region. These elements could easily substitute the oxygen atoms from the lattice of TiO2 and decrease its band gap by contributing their p-orbitals ( Citation18–21). This alters the electronic properties of TiO2 and results in the enhanced reaction rates for photocatalytic processes. Similarly, the heterojunctions with noble metals and other semiconductors also play a vital role in the enhanced photocatalytic activities of TiO2. Besides, modifications with inorganic acids are much meaningful to improve the photocatalytic activities of TiO2.
In this review, we have summarized various modification strategies of TiO2 by doping metals and non-metals, by constructing heterojunctions with noble metals and semiconductor oxides and modification with inorganic acids. Further, the photocatalytic mechanisms for water splitting, CO2 reduction and pollutants degradation have been explained elaborately. Although a number of review articles give deep insight into the structure modification and catalytic performances of TiO2 photocatalyst. This review attempted to provide detail knowledge on the structure modification, photocatalytic mechanisms and applications of TiO2 in photocatalysis.
2. TiO2 photocatalysis
Semiconductor oxide photocatalysts could absorb solar-light, when the energy of the incident photons matches the band gap energy (Eg) of semiconductors. Thus, under solar irradiation, the electrons are excited from the valence band (VB) to its conduction band (CB) and initiate reduction reactions on the catalyst surface. On the other hand, the holes left in the VB take part in oxidation reactions. When the electron–hole pairs are not used in photocatalytic reactions, they recombine very fast and release energy in the form of heat. The basic principles for redox reactions in semiconductor photocatalysts are shown in (A). First, photogenerated electron–hole pairs are produced. Second, the electron–hole pairs are separated and diffused to the electrode surface. Third, redox reactions take place on the photocatalyst surfaces ( Citation22). Among the semiconductor oxide photocatalysts, TiO2 an n-type semiconductor shows optical absorption in UV-region (λ < 400 nm) of solar spectrum. Its CB level (−0.3 eV) is more negative and suitable for the redox potential of H+/H2 (0 eV), while its VB level (2.9 eV) is more positive than the standard redox potential of O2/H2O (1.23 eV) vs. normal hydrogen electrode (NHE) as depicted in (B). When TiO2 is irradiated under solar-light, electron–hole pairs are produced. The electrons in the CB reduce H+ ions to evolve H2 and the holes in the VB oxidize H2O to form O2 ( Citation23). Similarly, TiO2 is also a potential candidate for both CO2 conversion to chemical fuels and pollutant oxidation to inorganic minerals. However, the wide band gap and fast recombination of photogenerated charge carrier’s (electron–hole pairs) still greatly limits its practical applications. To overcome these shortfalls, various attempts have been made. Among them, doping metal and non-metal elements to extend its optical absorption and enhance charge carriers separation, fabricating heterojunctions with other semiconductors to improve charge transfer and separation, surface modification with inorganic acids, noble metal deposition, metal ion implantation, and dye sensitization are widely investigated ( Citation24).
Figure 1. (A) Photogenerated charge carrier’s in semiconductors. Eph represent energy of the photon used. A means electron acceptor and D means electron donor. (B) Schematic illustration for the band gap structure showing the CB and VB positions of TiO2 and the redox potentials vs. NHE. Adapted from references ( Citation23) and ( Citation24) with permissions. Copyright 2015 and 2012, Royal Society of Chemistry.
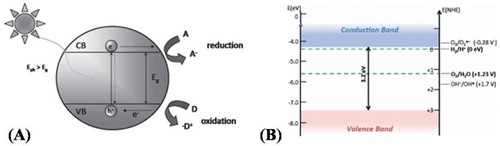
3. Modification strategies of TiO2
3.1. Doping metal and non-metal elements
It is widely accepted that the lattice structure and surface composition greatly affect the functionalities and efficiency of nanomaterials. Many researchers found that introducing electronically active secondary species into the crystal lattice of nanomaterials greatly alter its optical absorption and suppress the charge carrier’s recombination. In 2014, Hou et al. ( Citation25) reported the hydrothermal synthesis of N-doped TiO2 nanotubes, which were immersed in deionized water and various concentration ammonia solution. They investigated that the molar ratio between deionized water and ammonia aqueous solution had a great effect on the morphology of TiO2 nanotubes. They found that the samples immersed in ammonia solution have higher absorption than that immersed in water. Further, the band gap was effectively reduced from 3.2 to 2.84 eV, after doping nitrogen and facilitated the photogenerated carrier’s separation. This resulted in the dramatically enhanced visible-light activity of N-doped TiO2 nanotubes for methyl orange (MO) degradation. The schematic band gap structure of TiO2 and N-doped TiO2 is displayed in (A). Recently, McManamon et al. ( Citation26) reported the sol–gel synthesis of S-doped TiO2 nanoparticles. They investigated that S occupy some interstitial sites in the crystal lattice, which results in the generation of surface states between the VB and CB of TiO2. It is noticed that the incorporation of S into the crystal lattice dramatically reduced the band gap of TiO2 from 3.2 to 1.7 eV and facilitated visible-light absorption as shown in (B). It was found that after doping S, the visible-light activity of TiO2 for malachite green degradation was greatly enhanced.
Figure 2. Schematic illustration of the energy band structures (A) TiO2 and N-doped TiO2, (B) TiO2 and S-doped TiO2. Adapted from references ( Citation25) and ( Citation26) with permissions. Copyright 2014 and 2015, Elsevier Ltd.
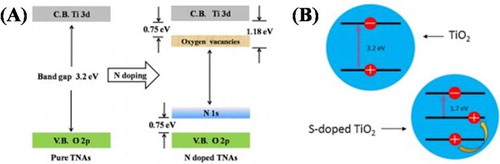
Bakar et al. ( Citation27) reported the synthesis of S-doped TiO2 photocatalysts by template-free oxidant peroxide route followed by crystallization through hydrothermal technique. It was investigated that doping S into the crystal lattice of TiO2 substituted oxygen atoms by sulfur anions and resulted in the narrowed band gap as depicted in (A). This greatly extended the optical absorption of TiO2 from UV to visible region. It was found that the incorporation of electrons deficient S atoms into the crystal lattice of TiO2 has effectively captured the photoinduced electrons and reduced charge carrier’s recombination. Further, S-doping generated surface oxygen vacancies, which acted as trapping center for the photogenerated electrons and reduced the recombination of electron–hole pairs. The synergistic effect of efficiently reduced charge carrier’s recombination and extended visible-light absorption resulted in the enhanced visible-light photocatalytic performance of S-doped TiO2 for methyl orange (MO) degradation. Wu et al. ( Citation28) reported the synthesis of C-doped TiO2 by a facile solvothermal method. It has been demonstrated that the incorporation of carbon atoms into the crystal lattice of TiO2 substituted oxygen atoms and resulted in the generation of oxygen vacancies. The band gap was considerably reduced from 3.2 to 2.39 eV, and the optical absorption was greatly altered. This resulted in the enhanced visible-light photocatalytic activities of TiO2 for nitric oxide (NO) and methyl orange (MO) oxidation, which is much superior to P25 and N-TiO2 photocatalysts. The schematic band gap structure is shown in (B).
Figure 3. Schematic illustration of the band gaps and photocatalytic processes on S-doped TiO2 (A), and C-doped TiO2 (B). Adapted from references ( Citation27) and ( Citation28) with permissions. Copyright 2016, Royal Society of Chemistry. and 2013, Elsevier Ltd.
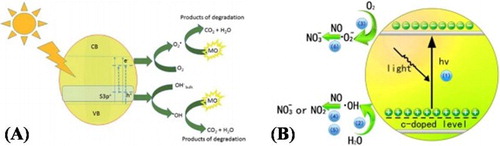
Sood et al. ( Citation29) reported the synthesis of Fe-doped TiO2 by ultrasonic-assisted hydrothermal method. It was investigated that the incorporation of Fe into the crystal lattice of TiO2 favored the substitution of Ti, as both have approximately the same ionic size. From UV-visible absorption spectra, it was observed that Fe doping extended the absorption of TiO2 from UV to visible region. The band gap was effectively reduced from 3.2 to 2.9 eV that resulted in the enhanced visible-light photocatalytic activity for paranitrophenol degradation. The schematic mechanism for pollutant degradation by Fe-doped TiO2 photocatalyst is depicted in (A). Roy et al. ( Citation30) reported the synthesis of Cu-doped TiO2 by facile hydrothermal method. They demonstrated that the band gap of TiO2 was significantly reduced from 3.2 eV to 2.06 eV. Further, the intermediate surface states were produced between the VB and CB of Cu-doped TiO2 and the transitions in visible-light was explained on the basis of d-orbital splitting of transition metals as depicted in (B). They suggested that the VB of TiO2 is composed of Ti−O 2σ, Ti−O 2π, and O 2π molecular orbitals. Similarly its conduction band bottom consists of Ti−Ti σ, Ti−Ti σ*, Ti−Ti π, Ti−Ti π*, and Ti−O π* molecular orbitals while the CB top has Ti−O σ* molecular orbitals. The energy level of π* orbitals in Cu-doped TiO2 shifted toward the VB maxima and increased the atomic number of transition metal. As a result, the energy levels of σ*(1) and σ*(2) were shifted up and downward and resulted in the narrow energy gap. The σ*(1) and σ*(2) molecular orbitals of Cu-TiO2 were found to be located near to the water and peroxides oxidation potentials. Hence, they directly interact with the σ orbitals of the intermediate products thereby reducing the over potential of oxygen evolution reactions.
Figure 4. Schematic illustration of the band gaps structure and photocatalytic processes (A) in Fe-doped TiO2 and (B) Cu-doped TiO2. Adapted from references ( Citation29) and ( Citation30) with permissions. Copyright 2015, Elsevier Ltd. and 2014, American Chemical Society.
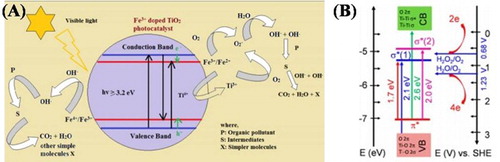
Tahir et al. ( Citation31) reported the synthesis of In-doped TiO2 nanoparticles by sol–gel method. It was found that the absorption band edge of In-doped TiO2 was slightly shifted toward lower wavelength direction and resulted in the enlarge band gap. Further, the incorporation of Indium into the crystal lattice of TiO2 has significantly increased the surface active sites and also suppressed the photogenerated charge carrier’s recombination. As a result, the photocatalytic activity for CO2 reduction under UV-light was obviously increased. The main product during CO2 reduction over TiO2 photocatalyst was CO, while Indium-doping remarkably enhanced the CH4 yield. The photocatalytic processes and charge carriers recombination and separation in TiO2 and In-doped TiO2 photocatalyst is depicted in (A,B).
Figure 5. Schematic illustration for the possible reaction mechanism of photocatalytic CO2 reduction with H2O: (A) photocatalytic CO2 conversion and redox reactions over In-doped TiO2 and (B) photogenerated charge carrier’s recombination and separation. Adapted from reference ( Citation31) with permission. Copyright 2015, Elsevier Ltd.
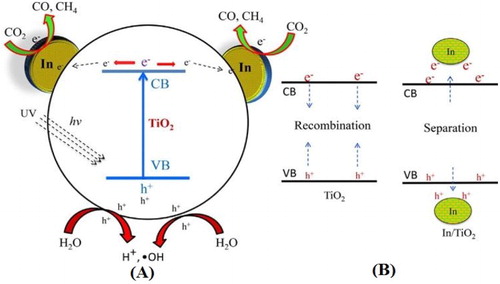
3.2. Modification with inorganic acids
It has been demonstrated that surface modification with inorganic acids can greatly alter the surface carried charge properties of TiO2 photocatalyst in neutral water. The newly introduced surface groups could greatly promote the separation of photogenerated charge carriers, leading to the enhanced photocatalytic activities. Naturally, it is accepted that the surface modification with inorganic acids greatly promotes the adsorption of O2 on the photocatalyst surface, which is much crucial for photocatalytic activities ( Citation32). He et al. ( Citation33) reported the hydrothermal synthesis of sulfuric acid modified TiO2 nanosheets. They investigated that H2SO4 modified TiO2 with {0 0 1} and {1 0 1} facets exhibit superior photocatalytic activity for CO2 conversion to CH4 and H2O oxidation under visible-light irradiation. These enhanced activities were attributed to the promoted surface OH groups, the generated oxygen vacancies and improved visible-light absorption. Further, it was investigated that the surface protonation resulted from acid modification improved the photogenerated charge carrier’s lifetime of TiO2 nanosheets and also prevailed the limitation of unfavorable side length for photogenerated charge carrier’s migration. Moreover, the photocatalyst showed high stability during the photocatalytic conversion of CO2. The mechanism of CO2 reduction and water oxidation over bulk anatase TiO2 and sulfuric acid modified TiO2 nanosheets is depicted in (A,B).
Figure 6. Schematic illustration for the energy band gap of the bulk (A) and sulfuric acid modified anatase TiO2 and the relevant redox potentials for CO2 and H2O (B). Adapted from reference ( Citation33) with permission. Copyright 2016, Elsevier Ltd.
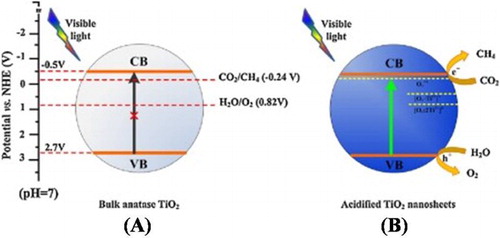
Cao et al. ( Citation34) reported the surface modification of TiO2 nanoparticles by simple post-treatment with monometallic sodium orthophosphate solution. It was found that phosphate modification has greatly promoted the adsorption of O2 on the surface of TiO2 photocatalyst. The enhanced adsorption of O2 was attributed to the substitution of –Ti–OH with –Ti–O–P–OH groups. As a result, the photogenerated electrons were effectively captured by the surface promoted O2, and the activities for photocatalytic degradation of pollutants were significantly improved. The surface forms of un-modified and phosphate-modified TiO2 are depicted in (A,B).
Figure 7. Schematic for the surface forms of un-modified (A) and phosphate-modified TiO2 (B). Adapted from reference ( Citation34) with permission. Copyright 2012, Royal Society of Chemistry.
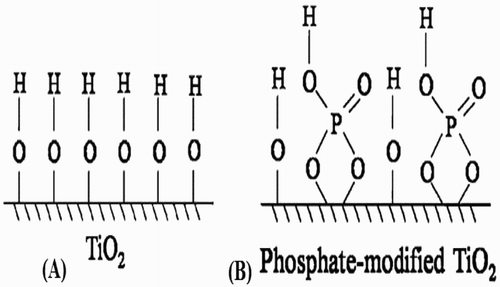
Liu et al. ( Citation35) reported the cobalt phosphate-modified TiO2 films, which were prepared by the post-treatment of TiO2 films with monometallic sodium orthophosphate solution followed by cobalt nitrate solution. They demonstrated that the photoelectrochemical water oxidation of TiO2 photocatalyst was greatly enhanced after modification with cobalt phosphate. This enhanced activity was attributed to the role of Co (II) ions linked with the surface of TiO2 through phosphate groups. It was confirmed that the surface linked cobalt (II) ions could effectively capture the photogenerated holes and generate high valence Co ions, which further induce oxidation reaction with H2O molecules and return to its original oxidation state. The schematic mechanism for the holes captured by Co (II) and the improved photoelectrochemical (PEC) water oxidation of TiO2 after modification with Co (II) phosphate is depicted in .
Figure 8. Schematic illustration for photoelectrochemical (PEC) water oxidation by cobalt (II) phosphate-modified TiO2. Adapted from reference ( Citation35) with permission. Copyright 2013, American Chemical Society.
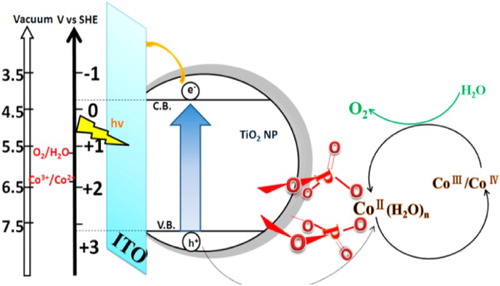
Luan et al. ( Citation36) reported the synthesis of hydrofluoric acid controlled 001-facet TiO2 nanoparticles by hydrothermal method. They observed that the 001-facet TiO2 exhibit exceptional photoactivities for pollutants degradation as compared to the unmodified TiO2. The enhanced photoactivities were attributed to the residual HF linked to the TiO2 surface via coordination bond between Ti4+ and F- as depicted in . The resultant −Ti:F−H bond greatly enhanced the adsorption of O2 on the surface of TiO2. Hence, the photogenerated electrons were effectively captured by the adsorbed O2 and charge carrier’s separation was improved.
Figure 9. Schematic illustration for the surface structure of unmodified (A) and HF modified TiO2 in atmosphere and water (B). Adapted from reference ( Citation36) with permission. Copyright 2013, American Chemical Society.
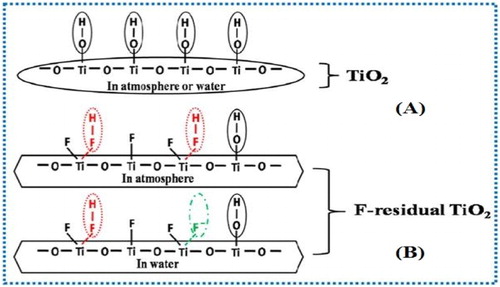
3.3. Constructing heterojunctions
The utilization of solar energy in a cost-effective manner is a mean of meeting the global energy demand and environmental remediation. Hence, it is highly desirable to convert solar-light into an energy storage medium ( Citation37). The photocatalytic water splitting to produce H2 and O2, CO2 conversion to chemical fuels and pollutant degradation under direct solar irradiation are the main issues of scientific research nowadays. The direct water splitting and CO2 reduction can be achieved using a single semiconductor. However, the proper redox potentials and effective absorption of visible-light are both the fundamentals of photocatalytic processes ( Citation38). In general, the semiconductor photocatalyst should have a wide band gap with proper VB and CB positions to provide effective separation of photogenerated charges, transfer energetic electrons and high stability toward photocorrosion. Semiconductor TiO2 fulfill all these requirements, but unfortunately the low quantum yield limits its practical application. This is because; TiO2 could absorb only UV-light, which accounts for ca. 4% of solar spectrum ( Citation39). To overcome this shortfall, constructing heterojunctions with noble metals and other semiconductor oxides with wide and narrow band gaps are widely employed. Constructing heterojunctions could effectively improve the optical absorption, charge carrier’s separation and stability of TiO2 photocatalyst. Therefore, it is highly desirable to choose suitable photocatalysts for coupling, in order to improve the stability and efficiency of TiO2 photocatalyst. Photocatalysts with large surface area exhibit more active sites, which is much crucial for photocatalytic reactions. Besides, the proper band gap and band edge alignment is also a key point because the relative energy level at interface junction also reveals the charge separation direction and transportation. Based on the above consideration, the heterojunctions are categorized into two classes; noble-metals-TiO2 heterojunctions and semiconductor oxides-TiO2 heterojunctions.
3.3.1. Noble-metals-TiO2 heterojunctions
Recently, it has been investigated that incorporation of noble metals into the crystal lattice of wide band gap semiconductors such as TiO2 have significantly improved the photocatalytic activity of TiO2 in visible-light region due to surface plasmon resonance (SPR) effect of noble metals. It has been demonstrated that the surface plasmon resonance effect mainly arises from the collective oscillations of electrons on surfaces of noble metal, and these energetic electrons transfer into the conduction band of the coupled semiconductor due to the Schottky barrier. Schottky barrier between noble metals and semiconductor oxides arises via Fermi levels equilibrium, as a result built-in electric field is formed at the interface which favors separation of photogenerated charges as depicted in ( Citation40,Citation41).
Figure 10. Equilibrium position of Semiconductor-Metal nanocomposites with Redox Couple (A) before and (B) after UV-Irradiation. Schematic illustration of photogenerated charge transfer and separation in semiconductor-metal heterojunctions (C) under UV-light and (D) under visible-light irradiation. Adapted from references ( Citation40) and ( Citation41) with permissions. Copyright 2004, American Chemical Society and 2013, Royal Society of Chemistry.
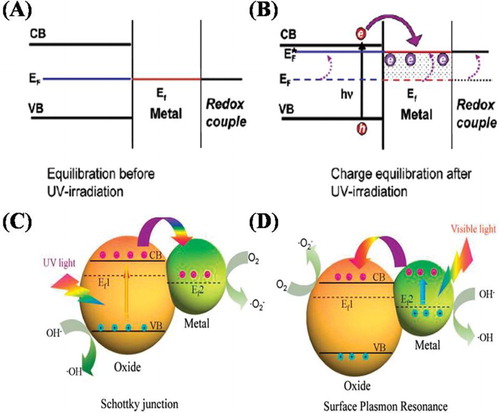
Ismail et al. ( Citation42) reported the synthesis of Pt/TiO2 nanocomposites by photodeposition and in-situ methods. TEM images of Pt/TiO2 nanocomposites ((A)), prepared via photodeposition method revealed that the pt nanoparticles with average diameter of ∼3 nm are uniformly dispersed on the surface of TiO2 nanoparticles. On the other hand, the TEM images of Pt/TiO2 nanocomposites obtained via in-situ method ((B)), showed that the Pt nanoparticles with average diameter of ∼15 nm are in close contact with TiO2 nanoparticles. HRTEM image of Pt/TiO2 nanocomposite is shown in (C), while the dark-field TEM image of mesoporous Pt/TiO2 nanocomposite and Pt/TiO2 nanocomposite is shown in (D and E). The photocatalytic activity of both type nanocomposites was evaluated for degradation of dichloroacetic acid (DCA). The result showed that the photocatalytic activity of TiO2 for DCA degradation was significantly enhanced by the addition of Pt islands and directly associated with the particle size of Pt. The activity of the nanocomposites prepared via photodeposition method was much higher than that of nanocomposites prepared by the in-situ method, even higher than the commercially available TiO2 (P25). This enhanced activity was attributed to the well-dispersed small-sized (3 nm) Pt nanoparticles on the surface of TiO2. They demonstrated that when Pt/TiO2 nanocomposites are irradiated with photons having energy higher than the band gap of TiO2, photogenerated electrons from the valance band are excited to the conduction band of TiO2 leaving the holes in the valance band. The closely contact Pt nanoparticles act as electrons sink to promote the reduction of oxygen on their surfaces and the holes in the valance band migrate to the surface of photocatalysts for oxidation of organic pollutants as depicted in (F).
Figure 11. TEM images of mesoporous Pt/TiO2 nanocomposites calcined at 450°C for 4 h: PtTiO2-3 (A) and in situ prepared PtTiO2-1 (B). HRTEM image of PtTiO2-3 (C), Dark-field TEM image of mesoporous PtTiO2-1 nanocomposites (D) and PtTiO2-3 nanocomposites (E). Schematic for the photocatalytic degradation of dichloroacetic acid over Pt/TiO2 nanocomposites (F). Adapted from reference ( Citation42) with permission. Copyright 2011, American Chemical Society.
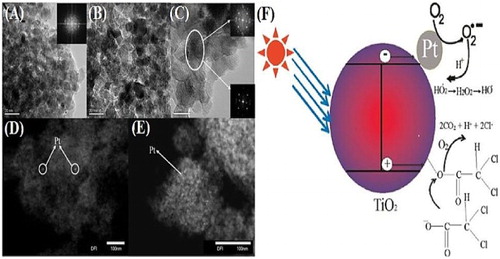
Jun Fang et al. ( Citation43) reported the synthesis of Au-TiO2 nanocomposites by a co-polymer assisted sol–gel method. From TEM micrograph ((A)), it was confirmed that Au and TiO2 nanoparticles are in close contact with each other. HRTEM image ((B)), showed well resolved (101) direction lattice fringes with d-spacing (d = 0.35 nm) and (200) direction lattice fringes with d-spacing (d = 0.23 nm) for TiO2 and Au, respectively. The activity of the as-prepared nanocomposites was evaluated for photocatalytic water splitting in the presence of electron donor (ascorbic acid), under visible light as shown in (C). All the samples showed excellent photocatalytic activity for H2 evolution, which were attributed to the surface Plasmon resonance effect of Au nanoparticles. To study the SPR effect of Au nanoparticles, they performed controlled wavelengths irradiation (500 ± 20 nm) experiments. It was confirmed that the surface plasmon excited hot electrons transfers from Au nanoparticles to the CB of TiO2; as a result, the visible-light photocatalytic activity of TiO2 for water reduction was significantly enhanced. The schematic mechanism for hydrogen evolution over Au-TiO2 nanocomposite is depicted in (D).
Figure 12. TEM image (A) HRTEM image (B), photocatalytic H2 production under visible light (λ > 420 nm) irradiation (C), and schematic mechanism for hydrogen evolution (D) of Au-TiO2 nanocomposites. Adapted from reference ( Citation43) with permission. Copyright 2012, Elsevier Ltd.
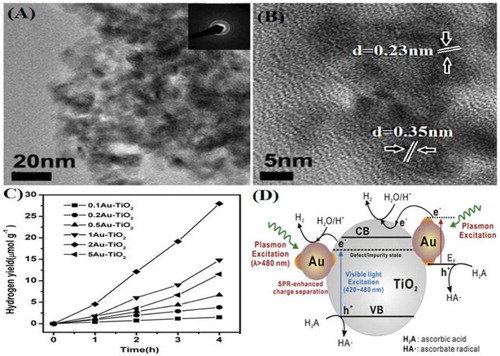
Dong Yang et al. ( Citation44) reported the synthesis of Ag/TiO2 nanocomposites by two different methods. Firstly, they prepared Ag/TiO2 heterojunctions by simple ultrasonic treatment and stirring of AgNO3 and TiO2 nanotubes (TNT) with the aid of 3-(3, 4 Dihydroxyphenyl) propionic acid (DiHPP), which acts both as a linker and reducer. Secondly, they followed photochemical reduction method for the synthesis of Ag/TiO2 heterojunctions without adding DiHPP. In the prepared Ag/TNT composite, about 3.8 nm in diameter of Ag nanocrystals were uniformly dispersed over the TiO2 nanotubes (TNT) surface and formed the heterojunction structure with TiO2 nanotubes (TNT). They demonstrated that the DiHPP first links to the TiO2 nanotubes (TNT) surface through the bidentate chelation of catechol group with Ti4+ and then acts as both an anchor and a reducer to in situ nucleate and grow Ag nanocrystals on the TiO2 nanotubes (TNT) surface. By adjusting the AgNO3 concentration, the loading amount of Ag nanocrystals on the TiO2 nanotubes (TNT) surface can be controlled easily, and the visible-light absorption ability of Ag/TNT heterojunctions enhances with increasing the Ag loading amount. Moreover, their photocatalytic activity was evaluated by the degradation capability of rhodamine B (RhB) under visible light. The Ag/TNT heterojunctions exhibit the high visible-light photocatalytic activity, which can almost degrade 100% rhodamine B (RhB) within 2 h. This excellent performance can be attributed to the local electric field caused by the surface plasmon resonance (SPR) of Ag nanocrystals and the high adsorption capability of TiO2 nanotubes (TNT) with the large specific surface area. The TEM and HRTEM images and the mechanism for photogenerated charge transfer and the photocatalytic degradation of RhB over Ag/TNT heterojunctions under visible-light irradiation are shown in .
Figure 13. TEM micrograph of TNT (A), TEM image of Ag/TNT heterojunction (B), HRTEM image (C), and TEM image of Ag/TNT nanocomposites synthesized without DiHPP (D). Schematic mechanism for charge transfer phenomenon and the photocatalytic degradation of RhB over Ag/TNT heterojunctions under visible light (E). Adapted from reference ( Citation44) with permission. Copyright 2015, American Chemical Society.
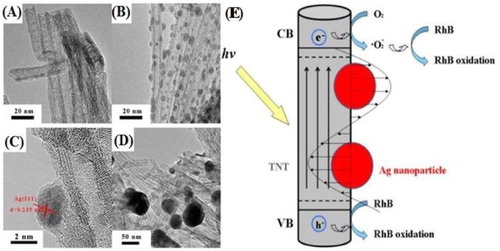
3.3.2. Semiconductors-TiO2 heterojunctions
In semiconductors-TiO2 heterojunctions, both the components can be excited by photons to generate charge carriers (electron–hole pairs). The charge transfer direction would mainly depend on the relative VB and CB position of both semiconductors. Primarily, the heterojunctions between TiO2 and other semiconductors are categorized into three types as shown in . In Type-I heterojunction, the two semiconductors should be either n-type or p-type. Suppose the two semiconductors are represented by A and B. The CB of B should be higher and its valance band should be lower than that of A. Therefore, both the electrons and holes would transfer to semiconductor A. In type-II heterojunctions, the photogenerated electrons would transfer from B to A, while the photogenerated holes would transfer from A to B. Such type of heterojunction is much beneficial, because of the effectively separation of electron–hole pairs. Type-III heterojunction is similar to type-II except for the more prominent difference in VB and CB positions, which requires a huge driving force for photogenerated charge transfer.
Figure 14. Band gap structures and migration of the charges in three type combination systems of TiO2 with other semiconductors. Adapted from reference ( Citation41) with permission. Copyright 2013, Royal Society of Chemistry.
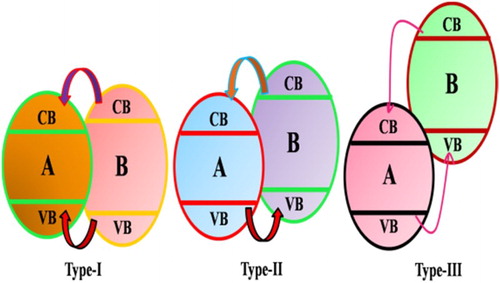
3.3.2.1. Heterojunctions with wide band gap semiconductors
Since the first report on solar-driven photoelectrochemical (PEC) energy conversion by TiO2 semiconductor photoelectrode in 1972, TiO2 has been widely investigated and regarded as one of the most promising candidates for water splitting, owing to its low cost, high stability, strong optical absorption and suitable band edge positions ( Citation45). However, TiO2 normally suffers from the intrinsic drawback of photocorrosion, which reduces its photoactivity and photostability. To solve this issue, research has been proliferated to improve the photostability and photocatalytic properties of nanosized TiO2 by coupling with ZnO. It has been well-established to remarkably enhance the photogenerated charge carrier’s separation efficiency because of the formation of heterojunction between them ( Citation46). Recently, some researchers ( Citation47–49) found that the photoelectrochemical performance of TiO2 photoanodes could be obviously improved by constructing heterojunction with ZnO. Zha et al. ( Citation50) reported TiO2/ZnO nanocomposites prepared via solvothermal method. They demonstrated that the as-prepared nanocomposites exhibit exceptional photocatalytic activity (97% in 30 min) for methyl orange (MO) degradation under UV light; which is attributed to the hierarchical nanostructure with a large surface area. The SEM images ((A,B)) and TEM image ((C)) of the heterojunctions show multi-layers of fan blades like morphology with an average length of 2.5–3 mm. The HRTEM image ((D)) reveals that the lattice fringes with d-spacing 0.28 nm in (101) planes correspond to anatase TiO2, while the later at (100) planes are attributed to hexagonal wurtzite ZnO. The photocatalytic mechanism over TiO2/ZnO heterojunctions is shown in (E). As the energy band gap of ZnO is 3.37 eV, hence, its conduction band (CB) and valence band (VB) potential is a little bit more negative than that of TiO2. When TiO2/ZnO nanocomposite is irradiated by photon energy to surpass its band gap energy, the photogenerated electrons from its VB are excited to the CB, which then transfer to the CB of TiO2 due to the potential difference between them. Simultaneously, the holes from the VB of TiO2 transfer thermodynamically to the VB of ZnO. This results in the enhanced photoactivities of TiO2.
Figure 15. SEM images (A, B), TEM image (C) and HRTEM image (D) of fan blades. Schematic diagram illustrating p–n junction and charge transfer and separation mechanism in TiO2/ZnO heterojunctions under UV light irradiation (E). Adapted from reference ( Citation50) with permission. Copyright 2015, Royal Society of Chemistry.
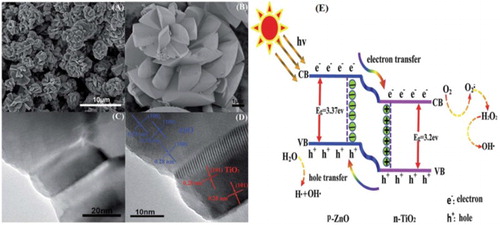
Recent reports ( Citation51–53) show that SrTiO3 (3.4 eV) could effectively improve the charge separation and photoelectrochemical performance of TiO2 by shifting its Fermi level to more negative potential in the formed SrTiO3/TiO2 heterojunction. Guo et al. ( Citation54) reported SrTiO3/TiO2 nanosheet heterojunctions prepared via hydrothermal method. They demonstrated that the as-prepared SrTiO3/TiO2 heterojunction exhibit porous structure with a high surface area. It was found that the material exhibit enhanced charge carriers separation, which is significant for dye sensitized solar cells (DSSCs). The photoelectric conversion efficiency and short-circuit current density of DSSCs based on SrTiO3/TiO2 heterojunctions were obviously enhanced as compared to the pure TiO2 nanosheets. The short-circuit current density of 12.55 mA cm−2 and photoelectric conversion efficiency of 7.42% was observed for DSSCs based on the SrTiO3/TiO2 heterojunctions under solar illumination. The photogenerated charge transfer and separation in SrTiO3/TiO2 heterojunction under UV-light irradiation is shown in .
Figure 16. Schematic diagram illustrating the energy band gaps and photogenerated charge transfer and separation phenomenon in SrTiO3/TiO2 heterojunction under UV light irradiation. Adapted from references ( Citation54) with permission. Copyright 2015, Royal Society of Chemistry.
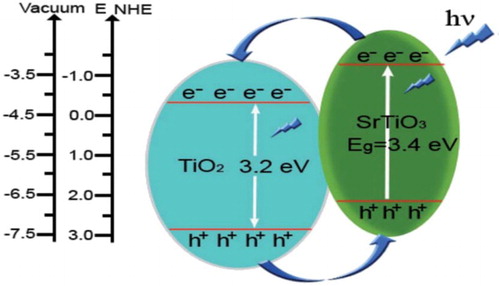
It is widely accepted that TiO2 exhibit fast recombination of photogenerated charge carrier’s which leads to the low photocatalytic efficiency. In order to overcome this shortfall, recent studies ( Citation55–58) reveal that photogenerated charge separation and catalytic activities of TiO2 can be obviously improved after coupling nanocrystalline SnO2. Wang et al. ( Citation59) reported hierarchical SnO2/TiO2 heterojunctions. They demonstrated that under UV light irradiation, the nanocomposites exhibit enhanced photocatalytic activities for Rhodamine B (RhB) degradation as compared to the bare TiO2 nanofibres. This enhanced photoactivity was attributed to the UV-light excited electrons transfer from the CB of TiO2 to the CB of SnO2. To well understand the charge transfer mechanism and the enhanced photoactivities, they proposed a schematic mechanism as shown in (A). The band gap and work function of anatase TiO2 is 3.2 and 4.2 eV, respectively. Its electron affinity is approximately 4.2 eV. On the other hand, the band gap and work function of rutile SnO2 are 3.5 and 4.4 eV, respectively. Its electron affinity is 0.5 eV higher than that of TiO2. The Fermi energy level of TiO2 is higher than that of SnO2, hence the photogenerated electrons of TiO2 easily transfer to SnO2 and the photogenerated holes of SnO2 transfer to the VB of TiO2. Therefore, the efficient charge separation will increase the lifetime of charge carriers and then enhance photocatalytic activity. On the other hand, in a single component system, most of the charges quickly recombine without doing any photochemical process and only a few charges take place in photocatalytic reactions. This results in the low photocatalytic activity. As can be seen from (B), the PL emission spectrum of TiO2 (TS0) is much intense compared to that of SnO2/TiO2 nanocomposite (TS1). This indicates that the charge recombination is greatly inhibited in the SnO2/TiO2 nanocomposite. This resulted in the enhanced photoactivity for RhB degradation.
Figure 17. (A) Schematic diagram showing the energy band structure and electron–hole pair separation in the SnO2/TiO2 heterostructure. (B) PL emission spectra of TS0 and TS1. Adapted from reference ( Citation59) with permission. Copyright 2009, American Chemical Society.
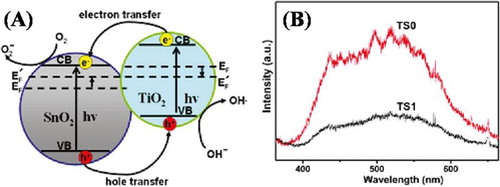
3.3.2.2. Heterojunctions with narrow band gap semiconductors
Hematite (a-Fe2O3) a narrow band gap (∼2.1 eV) semiconductor oxide has attracted tremendous attention owing to its potential applications as a low-cost, high stability, natural abundance, non-toxicity, visible-light responsive and electrode material for photoelectrochemical (PEC) water splitting (
Citation60,Citation61). The theoretical solar-to-chemical energy conversion efficiency for Fe2O3 is reported to be 16% (
Citation62). Coupling a-Fe2O3 to semiconductor TiO2 has seldom been reported, however, recently Li et al. (
Citation63) reported the branch-shaped a-Fe2O3/TiO2 nanocomposites, which were prepared by a combine electrospinning and hydrothermal approach. The nanocomposites consist of 3D porous TiO2 nano-fibers (diameter ∼ 70 nm) and the coupled a-Fe2O3 nanorods (length ∼ 100–200 nm). The nanocomposites exhibit large surface area (∼ 42.8 m2 g−1). They investigated that the resultant nanocomposites exhibit excellent visible-light photocatalytic activities for degradation of organic dyes, methylene blue (MB), including Congo red (CR), methyl orange (MO) and eosin red (ER). These enhanced activities were attributed to the large surface area, extended visible-light absorption and improved photogenerated charge separation. They proposed a charge transfer and separation mechanism, which is shown in . They demonstrated that under visible-light (400 cutoff) irradiation, the excited high-level energy electrons from the valance band (VB) of Fe2O3 would transfer thermodynamically to the conduction band (CB) of TiO2, and react with molecular oxygen (O2) to produce the superoxide anion and hydrogen peroxide (H2O2). While the photogenerated holes in the VB of Fe2O3 will react with hydroxyl species on the catalyst surface and produce the highly reactive hydroxyl radicals (.
OH), which are responsible for the oxidation of organic dye pollutants.
Figure 18. Schematic for band gap structures and visible-light induced charge carrier transfer and separation in a-Fe2O3/TiO2 nanocomposites. Adapted from reference ( Citation63) with permission. Copyright 2016, Royal Society of Chemistry.
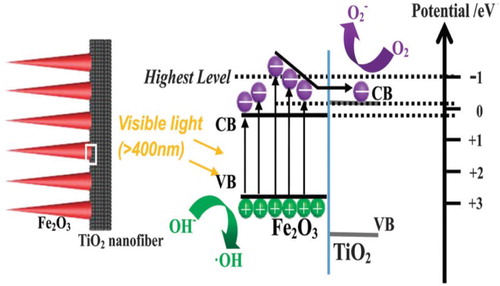
Mu et al. ( Citation64) reported the synthesis of In2O3–TiO2 nanocomposites via electrospinning and solvothermal techniques. They investigated that the In2O3 nanoparticles with an average particle size of about 30 nm were successfully grown onto the surface of TiO2 as confirmed by means of TEM analysis ((A)). Further, it was observed that TiO2 nanoparticles exhibit an average particle size of about 130 nm. Moreover, the In2O3–TiO2 heterojunction was confirmed by HRTEM image ((B)). The lattice fringes at (101) plane with d-spacing 0.31 nm were attributed to anatase TiO2, while the fringes at (222) plane with d-spacing 0.29 nm were attributed to In2O3. From selected area electron diffraction (SAED) patterns ((C)), it was confirmed that the nanoparticles exhibit polycrystalline structure. The visible-light photocatalytic activity of the fabricated nanocomposites was evaluated for RhB degradation. It was investigated that the nanocomposites exhibit superior photocatalytic activity for RhB degradation as compared to the TiO2 and In2O3 alone. From photocatalytic stability recycle test, it was confirmed that the photocatalyst exhibit high stability. The photocatalytic mechanism for In2O3–TiO2 nanocomposites was designed as shown in (D). The VB and CB position of TiO2 stand at 2.8 and −0.4 eV, respectively vs. NHE. Similarly, the VB and CB of In2O3 located at 2.17 and −0.63 eV vs. NHE. When heterojunction is formed between In2O3 and TiO2, and irradiated under visible light, the photogenerated electrons from the VB of In2O3 would transfer to the CB of TiO2. This will reduce the recombination of electron–hole pairs. Consequently, the improved charge separation would enhance the interfacial charge transfer to the adsorbed substrates, leading to a superior photocatalytic activity of In2O3–TiO2 nanocomposites for RhB degradation.
Figure 19. TEM image (A), HRTEM image (B), SAED patterns (C), and schematic diagram energy band structure and charge separation (D) of In2O3–TiO2 nanocomposites. Adapted from reference ( Citation64) with permission. Copyright 2012, American Chemical Society.
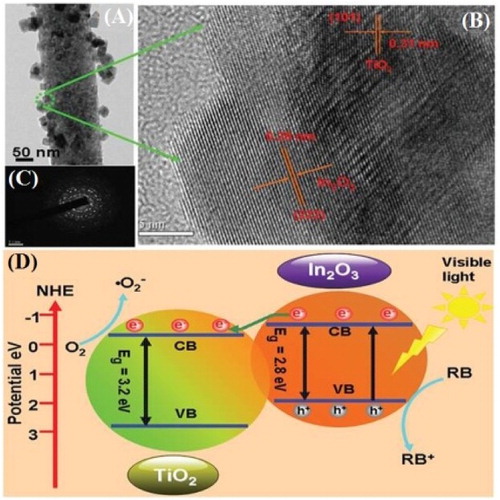
CdS is a visible-light responsive n-type semiconductor, which has been widely investigated among narrow band gap semiconductors. It has been regarded as an attractive material owing to its high efficiency for water reduction to evolve hydrogen. The narrow band gap (∼2.4 eV) with sufficient negative conduction band position for the reduction potential of protons made this material more valuable for practical applications (
Citation65). However, the poor stability, particle agglomeration and high recombination rates of photogenerated charges still greatly impair its practical use for large-scale water splitting (
Citation66,Citation67). Therefore, the fabrication of CdS-TiO2 nanocomposites has been attempted to diminish recombination, improve its surface area and to stabilize the material. Liu et al. (
Citation68) reported One-dimensional (1D) CdS@TiO2 core−shell nanocomposites (CSNs) prepared via two-step solvothermal method. The visible-light photocatalytic activities of the as-prepared 1D CdS@TiO2 CSNs nanocomposites were evaluated for selective oxidation of alcohols to aldehydes. It was investigated that the nanocomposites exhibit obviously enhanced visible-light activity for both conversion and yield as compared to bare CdS NWs, which is attributed to the prolonged lifetime of photogenerated charge carriers after coupling TiO2. Moreover, it is noticed that the photogenerated holes from CdS core can be stuck by the TiO2 shell, as confirmed by means of controlled radical trapping experiments and the selective reduction of heavy-metal ions, Cr(VI), over 1D CdS@TiO2 CSNs. Hence, it is concluded that the reaction mechanism of visible-light catalytic oxidation of alcohols over 1D CdS@TiO2 CSNs is actually different from that over 1D CdS NWs. It is demonstrated that under visible-light irradiation (λ = 520 ± 15 nm), the excited electrons from the valence band (VB) of CdS NWs in the 1D CdS@TiO2 CSNs would transfer thermodynamically to the TiO2 shell. Simultaneously, the holes in the valance band of CdS NWs would stuck by the TiO2 shell. As a result, the lifetime of charge carriers will prolong. The photogenerated electrons react with molecular oxygen and produce superoxide radicals , which oxidize the alcohols to the corresponding aldehydes ().
Figure 20. Schematic diagram for the energy band gaps and the possible reaction mechanism for the selective oxidation of alcohols to the corresponding aldehydes over 1D CdS@TiO2 CSNs under visible-light. Adapted from reference ( Citation68) with permission. Copyright 2012, American Chemical Society.
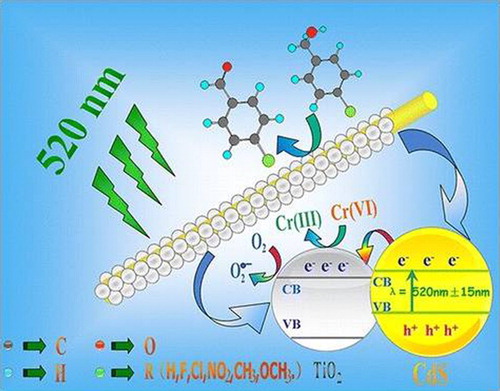
3.4. Dye-sensitized TiO2
Sensitization of TiO2 with organic dyes is a promising and extremely effective technique used for renewable solar H2 production, electricity generation, and various organic pollutants oxidation. In this technique, the dye molecules anchored on TiO2 are excited with solar photons to generate highly excited electrons which then transfer to the conduction band of TiO2. Subsequently, the electrons in the conduction band of TiO2 are transported to the interfacial electron acceptors (e.g. H2O, I3−, H+, O2, etc.) and induce various redox reactions as depicted in . In this way, the effective solar energy utilization and interfacial charge transfer from the excited dye to the conduction band of TiO2 should be the key parameters to accomplish a high level of photo-conversion efficiency ( Citation69).
Figure 21. Schematic of the visible-light induced H2 evolution and pollutant oxidation on organic dye-sensitized TiO2 in water. (1) Photo-excitation and relaxation of organic dye, (2) electrons injection to the conduction band of TiO2 (or Pt/TiO2), (3) H2 production, (4) oxidation of dye, (5) regeneration of dye, (6) oxidation of the electron donors (ED), (7) and (9): reduction of Cr(VI) to Cr(III), (8): reduction of the dissolved O2 to superoxide anion, (10) oxidation of the superoxide anion. Adapted from reference ( Citation69) with permission. Copyright 2012, Elsevier Ltd.
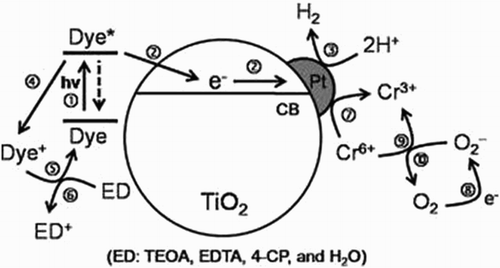
Choi et al. ( Citation69) reported the synthesis of organic dye-sensitized TiO2 particles for H2 evolution from H2O and 4-chlorophenol and Cr(VI)) pollutants oxidation under visible-light (λ > 420 nm). They investigated the effect of various anchoring groups. For this, they prepared Ru-free organic dyes of donor–acceptor configuration with different numbers (n = 1, 2, and 3) of carboxylate-anchoring groups which were referred as D1, D2 and D3, respectively. They found that all the three kinds of dyes are effective for H2 production in the presence of electron donors such as tri-ethanol-amine (TEOA) and ethylene-diamine-tetra-acetic acid (EDTA) with the orders: D3–D2 > D1 (TEOA) and D3 > D2–D1 (EDTA). From FTIR analysis, it was confirmed that D1 and D3 are probably anchored on the surface of TiO2 mainly by means of bidentate modes with a single and double carboxylates, respectively. Further, they investigated that D2 is anchored with single and double carboxylates, depending on the competing electron donors. They demonstrated that the carboxylates number is less important in the sensitized pollutant oxidation because of the different photochemical conditions and reaction pathways. The tri-branched organic dye molecules with mono-, di-, and tri-carboxylate anchoring groups (sensitizers D1, D2 and D3, respectively are depicted in .
Figure 22. The structural representation of tri-branched organic dye molecules with mono-, di-, and tri-carboxylate anchoring groups (sensitizers D1, D2, and D3). Adapted from reference ( Citation69) with permission. Copyright 2012, Elsevier Ltd.
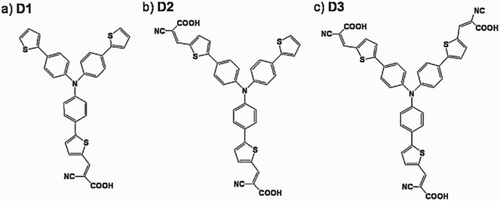
Tiwari et al. ( Citation70) reported a series of donor-based triphenylamine (TPA) dyes in sensitized visible-light H2 generation over TiO2-Pt photocatalysts. First, different dyes such as 4-(diphenylamino) phenylcyanoacrylic acid (DN-F01), 5-[4-(diphenylamino)phenyl]thiophene-2-cyanoacrylic acid (DN-F02), and 3-(5-(4-(diphenylamino)styryl) thiophen-2-yl)-2-cyanoacrylic acid (DN-F03), (E)-3-(5-(4-(bis(20,40-dibutoxy-[1,10-biphenyl]-4-yl)amino)phenyl) thiophen-2-yl)-2-cyanoacrylic acid (DN-F04) and 3-(6-(4-(bis(20,40-dibutoxy-[1,10-biphenyl]-4-yl)amino)phenyl)-4,4-dihexyl-4H-cyclopenta[1,2-b:5,4-b0] dithiophen-2-yl)-2-cyanoacrylic acid (DN-F05) were adsorbed on the surface of TiO2-Pt, and the resultant photocatalysts were subjected for H2 evolution under visible-light in the presence of sacrificial electron donor triethanolamine (TEOA). They investigated that the extended visible-light absorption and the enhanced interfacial charge transfer from excited dye molecules to the conduction band of TiO2 are much significant in DN-F05-TiO2. They found that the DN-F05 dye-sensitized TiO2-Pt photocatalyst display superior turnover number (1864) and noticeable quantum efficiency (AQE ∼44%) compared to their analogous simple architecture dye molecules. Further, they noticed that the additional donating-groups such as dibutoxyphenyl and dihexylcyclopentyl p-conjugated-bridge units provide exceptional surface protection through steric hindrance. This leads to the high photocatalytic activity. The mechanism of H2 evolution over the dye-sensitized TiO2-Pt photocatalyst is shown in . The HOMO and LUMO positions of the dye attribute to the formal redox potentials and the formal excited state oxidation potential, respectively. In H2 evolution process, first, the dye molecules are excited from the ground state to the excited state by the absorption of incident-photon flux, due to the intra-molecular p–p* transition. Second, the excited electrons immediately transfer to the conduction band of TiO2 before quenching by emission and subsequent formation of the oxidized state dye. Third, the transferred electrons to the conduction band of TiO2 are transported toward the co-catalyst Pt site. Fourth, these electrons reduce H2O molecules at Pt site. Finally, the oxidized dyes reduce to its ground state by accepting electrons from the oxidized sacrificial electron donors. Hence, the reduction of the oxidized dye and the regenerated by sacrificial electron donors resolve the efficiency of H2 evolution.
Figure 23. Proposed mechanism for the efficient visible-light H2 evolution over dye-sensitized TiO2 photocatalyst. Adapted from reference ( Citation70) with permission. Copyright 2015, Elsevier Ltd.
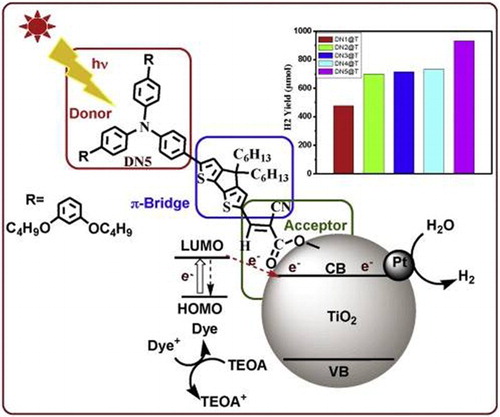
Lee et al. ( Citation71) reported the fabrication of phenothiazine-based organic dyes/TiO2-Pt composites with systematic alkyl-chains and two-anchoring groups. They demonstrated that the composite showed excellent visible-light photoactivities for H2O splitting. Further, they investigated that the phenothiazine dyes with longer alkyl-chains exhibit high stability during photocatalytic processes. Their stability was attributed to the cationic radical produced by one electron oxidation. They demonstrated that the two-anchoring groups can be introduced at position 3 and 7 of the phenothiazine dyes. However, the multi-anchoring groups can greatly improve the photostability of the photocatalysts during photocatalytic processes. They demonstrated that the phenothiazine-based dyes with a directly connected anchoring group exhibit enhance visible-light harvesting efficiencies compared to the common donor–acceptor sort of dyes with additional p-conjugated bridges. The alkyl groups on nitrogen can induce orientation of dyes on TiO2, which may possibly result in the efficient electron transfer from the excited dye molecules to the conduction band of TiO2. This results in the improved photoactivities of the composites for H2O splitting. The schematic of phenothiazine-based dyes (P1–P5) with two anchoring groups is depicted in .
Figure 24. Schematic of the phenothiazine-based dyes/TiO2-Pt composites for efficient visible-light-driven H2O splitting. Adapted from reference ( Citation71) with permission. Copyright 2012, Royal Society of Chemistry.
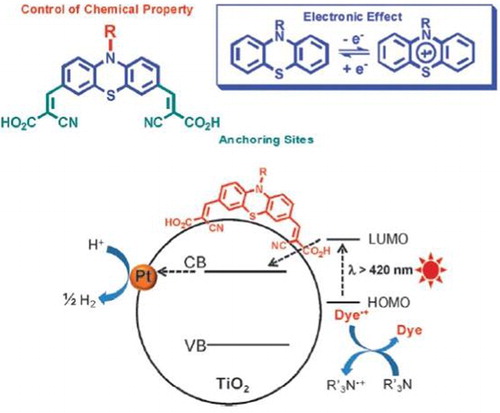
Bae et al. ( Citation72) reported the synthesis of a variety of Ru-bipyridyl with di-, tetra-, and hexa-carboxylate functionalities (C2, C4 and C6) and with di-, tetra-, and hexa-phosphonate anchoring groups (P2, P4 and P6) sensitized TiO2 samples for visible-light catalytic H2 evolution in aqueous suspension containing an electron donating agent (EDTA). They investigated that the efficiencies of C and P sensitizers depend on the number and type of anchoring groups. The adsorption of P sensitizers on TiO2 surface was strong enough not to be hindered by EDTA, whereas the adsorption of C sensitizers was significantly inhibited. Thus, the P-TiO2 exhibited exceptional visible-light activity for H2 evolution compared to that of the C-TiO2. Among the six different kinds of sensitizers, P2 was the most active for H2 evolution.
When the Ru-bipyridyl-TiO2 samples were irradiated under visible-light, the excited electrons of dye molecules were transferred to the conduction band (CB) of TiO2 which then initiated interfacial chemical reaction. The schematic of visible-light induced H2 evolution over dye-sensitized TiO2 is depicted in .
Figure 25. The electron pathways are represented by various numbers: (1) electron transfer from the excited sensitizer to the conduction band of TiO2; (2) back-electron transfer to the oxidized sensitizer (RuIIILx); (3) the migration and trapping of electrons in Pt deposits; (4) the interfacial transfer of electron to H2O (or H+) on Pt; (5) the regeneration of sensitizer by an electron donor (D) (i.e. EDTA). Adapted from reference ( Citation72) with permission. Copyright 2006, American Chemical Society.
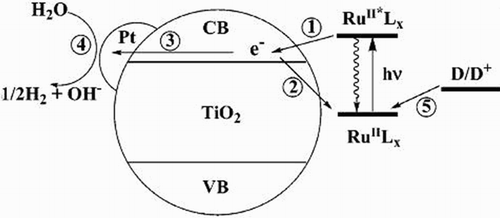
3.5. Metal ion-implanted TiO2
The metal ion implantation is another strategy used to modify the electronic structure of semiconductors. In this technique, the metal ions are accelerated in the electronic field and injected to the target (sample) as the ion beam. These metal ions interact with the sample surfaces in different ways depending on their kinetic energy. Further, the metal ions are highly accelerated to have enough kinetic energy (50–200 keV) and then implanted into the semiconductors bulk ( Citation73). Yamashita et al. ( Citation73) reported the synthesis of (V+, Mn+, Fe+) metal ion-implanted TiO2 photocatalysts. They investigated that at high energy acceleration and subsequent annealing of these metal ion-implanted TiO2 photocatalysts in O2 at 725 K, the absorption spectra was remarkably shifted toward visible-light region. The resultant photocatalysts showed exceptional photocatalytic activity for 2-propanol (diluted in H2O) degradation under visible-light irradiation. They confirmed by means of XAFS analysis that the implanted metal ions are sited at Ti4+ lattice positions in TiO2 after annealing. They demonstrated that the substitution of Ti ions in TiO2 matrix with the implanted metal ions is much important to modify TiO2 for visible-light photocatalytic activities. Takeuchi et al. ( Citation74) reported the synthesis of transition metal ions (Cr and V) implanted TiO2 thin film photocatalysts by an advanced metal ion-implantation technique. They observed that the absorption spectra of the resultant thin film photocatalysts were remarkably shifted toward visible-light region. As a result, the photocatalysts showed significant visible-light catalytic decomposition of NOx into N2 and O2 environment at 275 K. Zhou et al. ( Citation75) reported the fabrication of V+ ion-implanted P25 TiO2 photocatalysts by an ion implantation method. They investigated that the materials exhibited remarkably enhanced visible-light catalytic activity for the degradation of formic acid. From HRTEM micrographs, they have confirmed that the V ions exist in the form of VO2(T) in the P25 TiO2 matrix. Based on the photoluminescence spectra, they suggested that the enhanced visible-light activity of the V+ ion-implanted P25 TiO2 photocatalyst for formic acid degradation is also attributed to improved charge separation. Lam et al. ( Citation76) reported the synthesis of chromium (Cr) ion-implanted TiO2 thin films. They investigated that the visible-light catalytic activity of the resultant Cr/TiO2 photocatalyst for degradation of gaseous formaldehyde was considerably enhanced. Wang et al. ( Citation77) reported the fabrication of Sn ion-implanted TiO2 thin films by an ion implantation technique. They used different doses of Sn ion implantation for surface modification of TiO2. They demonstrated that the Sn4+ ions substitute Ti lattice site in TiO2 matrix. They investigated that TiO2 with an optimum dose of implanted Sn ions exhibited enhanced photoactivity for Rhodamine B (RhB) degradation. The enhanced photoactivity was attributed to the extended light absorption from ultraviolet to visible region and enhanced charge separation as a result of Sn4+ introduced electron and holes trapping states near the conduction band bottom and valance band top of TiO2.
4. Conclusions
In this review, we have described various modification strategies of TiO2 and their applications in photocatalysis. We have discussed basic concepts related to surface modification including metal nanoparticles deposition, inorganic acids modification, elemental doping, heterojunctions with other semiconductors, dye sensitization and metal ion implantation. Further, this review emphasizes the basic mechanisms for photophysical and photochemical processes and the physical concepts suitable for material properties interpretation and structural property correlations. This review would help us to synthesize low-cost TiO2-based material and also help us to deeply understand the photophysical and photochemical processes involved during the photocatalytic CO2 conversion, water splitting, and pollutants degradation over TiO2-based materials.
Disclosure statement
No potential conflict of interest was reported by the authors.
Notes on contributors
M. Humayun received his PhD degree in Chemistry & Materials Science from Heilongjiang University China in 2017. He received the distinguished PhD scholar award (30000 RMB) from the Chinese Government in 2016. Furthermore, he received the outstanding graduate award from Heilongjiang University in 2017. Currently, he is working in Prof. Luo Wei group as a postdoc researcher in the School of Optical & Electronics Information, Huazhong University of Science & Technology (HUST) China, under the China postdoc research program. He received the China Postdoctoral Science Foundation project grant in 2017 (Grant no. 2017M622404). His current research interests include the design of visible-light-driven nanophotocatalysts for solar fuel production and environmental purification.
F. Raziq received his PhD degree in Chemistry & Materials Science from Heilongjiang University, in 2017. He received the distinguished PhD scholar award (30000 RMB) from the Chinese Government in 2016. Currently, he is working as a postdoc researcher in Prof. Liang Qiao group Institute of Fundamental and Frontier of Sciences, University of Electronic Science and Technology of China, under the China postdoc research program. His current research interests include synthesis of visible-light responsive materials for CO2 conversion and water splitting.
A. Khan received his PhD degree in Physical Chemistry from Quaid-I-Azam University, Islamabad, Pakistan, in 2010. He joined the Department of Chemistry Abdul Wali Khan University Mardan Pakistan as an Assistant Professor in 2011. His research interests include Polymer Science, Colloids & Surfactants, and Materials Chemistry.
W. Luo received his PhD degree in Electronic Science and Technology from Huazhong University of Science & Technology China, in 2009. From 2009 to 2012, he worked as a postdoc researcher in the School of Optical & Electronics Information, Huazhong University of Science & Technology (HUST) China. From 2012 to 2016, he worked as a Lecturer in the School of Optical & Electronics Information, (HUST), China. From 2014 to 2016, he worked as a postdoc researcher in the United States University of Michigan (ANA). From 2016 till date, he is working as an Associate Professor in the School of Optical & Electronics Information, (HUST), China. His current research interests include SAW sensors and low-dimensional semiconductor sensing materials.
Additional information
Funding
References
- Bian, Z.; Tachikawa, T.; Zhang, P.; Fujitsuka, M.; Majima, T. J. Am. Chem. Soc. 2014, 136, 458. doi: 10.1021/ja410994f
- Rossetti, I.; Villa, A.; Compagnoni, M.; Prati, L.; Ramis, G.; Pirola, C.; Bianchi, C.; Wang, W.; Wang, D. Catal. Sci. Technol. 2015, 5, 4481. doi: 10.1039/C5CY00756A
- Venkatkarthick, R.; Davidson, D.; Ravichandran, S.; Vengatesan, S.; Sozhan, G.; Vasudevan, S. Catal. Sci. Technol. 2015, 5, 5016. doi: 10.1039/C5CY00805K
- Chen, J.; Cen, J.; Xu, X.; Li, X. Catal. Sci. Technol. 2016, 6, 349. doi: 10.1039/C5CY01289A
- Chen, H.; Tang, M.; Rui, Z.; Wang, X.; Ji, H. Catal. Today 2016, 264, 23. doi: 10.1016/j.cattod.2015.08.024
- Ferrari-Lima, A.; De Souza, R.; Mendes, S.; Marques, R.; Gimenes, M.; Fernandes-Machado, N. Catal. Today 2015, 241, 40. doi: 10.1016/j.cattod.2014.03.042
- Kaplan, R.; Erjavec, B.; Pintar, A. Appl. Catal. A: Gen. 2015, 489, 51. doi: 10.1016/j.apcata.2014.10.018
- Alkaim, A.F.; Kandiel, T.A.; Hussein, F.H.; Dillert, R.; Bahnemann, D.W. Appl. Catal. A: Gen. 2013, 466, 32. doi: 10.1016/j.apcata.2013.06.033
- Lin, W.; Zheng, H.; Zhang, P.; Xu, T. Appl. Catal. A: Gen. 2016, 521, 75. doi: 10.1016/j.apcata.2015.10.032
- Liu, X.; Zhang, H.; Liu, C.; Chen, J.; Li, G.; An, T.; Wong, P.-K.; Zhao, H. Catal. Today 2014, 224, 77. doi: 10.1016/j.cattod.2013.09.041
- Luan, Y.; Jing, L.; Meng, Q.; Nan, H.; Luan, P.; Xie, M.; Feng, Y. J. Phys. Chem. C. 2012, 116, 17094. doi: 10.1021/jp305142j
- Pang, Y.L.; Lim, S.; Ong, H.C.; Chong, W.T. Appl. Catal. A: Gen. 2014, 481, 127. doi: 10.1016/j.apcata.2014.05.007
- Chen, J.; Qiu, F.; Xu, W.; Cao, S.; Zhu, H. Appl. Catal. A: Gen. 2015, 495, 131. doi: 10.1016/j.apcata.2015.02.013
- Xie, M.; Feng, Y.; Luan, Y.; Fu, X.; Jing, L. Chem. Plus Chem. 2014, 79, 737.
- Kočí, K.; Matějů, K.; Obalová, L.; Krejčíková, S.; Lacný, Z.; Plachá, D.; Čapek, L.; Hospodková, A.; Šolcová, O. Appl. Catal. B: Environ. 2010, 96, 239. doi: 10.1016/j.apcatb.2010.02.030
- Yang, J.; Zhang, X.; Liu, H.; Wang, C.; Liu, S.; Sun, P.; Wang, L.; Liu, Y. Catal. Today 2013, 201, 195. doi: 10.1016/j.cattod.2012.03.008
- Zhang, X.; Cui, H.; Humayun, M.; Qu, Y.; Fan, N.; Sun, X.; Jing, L. Sci. Rep. 2016, 6.
- Zhang, G.; Zhang, Y.C.; Nadagouda, M.; Han, C.; O’Shea, K.; El-Sheikh, S.M.; Ismail, A.A.; Dionysiou, D.D. Appl. Catal. B: Environ. 2014, 144, 614. doi: 10.1016/j.apcatb.2013.07.058
- Todorova, N.; Vaimakis, T.; Petrakis, D.; Hishita, S.; Boukos, N.; Giannakopoulou, T.; Giannouri, M.; Antiohos, S.; Papageorgiou, D.; Chaniotakis, E.; Trapalis, C. Catal. Today 2013, 209, 41. doi: 10.1016/j.cattod.2012.11.019
- Bessegato, G.G.; Cardoso, J.C.; Zanoni, M.V.B. Catal. Today 2015, 240, 100. doi: 10.1016/j.cattod.2014.03.073
- Cavalcante, R.P.; Dantas, R.F.; Bayarri, B.; Gonzalez, O.; Gimenez, J.; Esplugas, S.; Machulek, A. Catal. Today 2015, 252, 27. doi: 10.1016/j.cattod.2014.09.030
- Marschall, R. Adv. Funct. Mater. 2014, 24, 2421. doi: 10.1002/adfm.201303214
- Gao, M.; Zhu, L.; Ong, W.L.; Wang, J.; Ho, G.W. Catal. Sci. Technol. 2015, 5, 4703. doi: 10.1039/C5CY00879D
- Dhakshinamoorthy, A.; Navalon, S.; Corma, A.; Garcia, H. Energ. Environ. Sci. 2012, 5, 9217. doi: 10.1039/c2ee21948d
- Hou, X.; Wang, C.-W.; Zhu, W.-D.; Wang, X.-Q.; Li, Y.; Wang, J.; Chen, J.-B.; Gan, T.; Hu, H.-Y.; Zhou, F. Solid State Sci. 2014, 29, 27. doi: 10.1016/j.solidstatesciences.2014.01.007
- McManamon, C.; O’Connell, J.; Delaney, P.; Rasappa, S.; Holmes, J.D.; Morris, M.A. J. Mol. Catal. A: Chem 2015, 406, 51. doi: 10.1016/j.molcata.2015.05.002
- Bakar, S.A.; Ribeiro, C. RSC Adv. 2016, 6, 36516. doi: 10.1039/C6RA03819K
- Wu, X.; Yin, S.; Dong, Q.; Guo, C.; Li, H.; Kimura, T.; Sato, T. Appl. Catal. B: Environ. 2013, 142–143, 450. doi: 10.1016/j.apcatb.2013.05.052
- Sood, S.; Umar, A.; Mehta, S.K.; Kansal, S.K. J. Colloid Interf. Sci. 2015, 450, 213. doi: 10.1016/j.jcis.2015.03.018
- Roy, N.; Sohn, Y.; Leung, K.T.; Pradhan, D. J. Phys. Chem. C 2014, 118, 29499. doi: 10.1021/jp508445t
- Tahir, M.; Amin, N.S. Appl. Catal. B: Environ. 2015, 162, 98. doi: 10.1016/j.apcatb.2014.06.037
- Li, Z.; Luan, Y.; Qu, Y.; Jing, L. ACS Appl. Mater. Inter. 2015, 7, 22727. doi: 10.1021/acsami.5b04267
- He, Z.; Tang, J.; Shen, J.; Chen, J.; Song, S. Appl. Surf. Sci. 2016, 364, 416. doi: 10.1016/j.apsusc.2015.12.163
- Cao, Y.; Jing, L.; Shi, X.; Luan, Y.; Durrant, J.R.; Tang, J.; Fu, H. Phys. Chem. Chem. Phys. 2012, 14, 8530. doi: 10.1039/c2cp41167a
- Liu, D.; Jing, L.; Luan, P.; Tang, J.; Fu, H. ACS Appl. Mater. Inter. 2013, 5, 4046. doi: 10.1021/am400351m
- Luan, Y.; Jing, L.; Xie, Y.; Sun, X.; Feng, Y.; Fu, H. ACS Catal. 2013, 3, 1378. doi: 10.1021/cs400216a
- Resasco, J.; Zhang, H.; Kornienko, N.; Becknell, N.; Lee, H.; Guo, J.; Briseno, A.L.; Yang, P. ACS Cen. Sci. 2016, 2, 80. doi: 10.1021/acscentsci.5b00402
- Raziq, F.; Qu, Y.; Zhang, X.; Humayun, M.; Wu, J.; Zada, A.; Yu, H.; Sun, X.; Jing, L. J. Phys. Chem. C. 2016, 120, 98. doi: 10.1021/acs.jpcc.5b10313
- Ghasemi, S.; Esfandiar, A.; Setayesh, S.R.; Habibi-Yangjeh, A.; Gholami, M. Appl. Catal. A: Gen. 2013, 462–463, 82. doi: 10.1016/j.apcata.2013.04.029
- Subramanian, V.; Wolf, E.E.; Kamat, P.V. J. Am. Chem. Soc. 2004, 126, 4943. doi: 10.1021/ja0315199
- Jing, L.; Zhou, W.; Tian, G.; Fu, H. Chem. Soc. Rev. 2013, 42, 9509. doi: 10.1039/c3cs60176e
- Ismail, A.A.; Bahnemann, D.W. J. Phys. Chem. C. 2011, 115, 5784. doi: 10.1021/jp110959b
- Fang, J.; Cao, S.-W.; Wang, Z.; Shahjamali, M.M.; Loo, S.C.J.; Barber, J.; Xue, C. Int. J. Hydrogen Energ. 2012, 37, 17853. doi: 10.1016/j.ijhydene.2012.09.023
- Yang, D.; Sun, Y.; Tong, Z.; Tian, Y.; Li, Y.; Jiang, Z. J. Phys. Chem. C. 2015, 119, 5827. doi: 10.1021/jp511948p
- Xu, M.; Da, P.; Wu, H.; Zhao, D.; Zheng, G. Nano Lett. 2012, 12, 1503. doi: 10.1021/nl2042968
- Xiao, F.-X. ACS Appl. Mater. Inter. 2012, 4, 7055. doi: 10.1021/am302462d
- Pan, K.; Dong, Y.; Zhou, W.; Pan, Q.; Xie, Y.; Xie, T.; Tian, G.; Wang, G. ACS Appl. Mater. Inter. 2013, 5, 8314. doi: 10.1021/am402154k
- Chen, D.; Zhang, H.; Hu, S.; Li, J. J. Phys. Chem. C. 2008, 112, 117. doi: 10.1021/jp077236a
- Yuan, S.; Mu, J.; Mao, R.; Li, Y.; Zhang, Q.; Wang, H. ACS Appl. Mater. Inter. 2014, 6, 5719. doi: 10.1021/am500314n
- Zha, R.; Nadimicherla, R.; Guo, X. J. Mater. Chem. A. 2015, 3, 6565. doi: 10.1039/C5TA00764J
- Zhu, F.; Li, C.; Ha, M.N.; Liu, Z.; Guo, Q.; Zhao, Z. J. Mater. Sci. 2016, 51, 4639. doi: 10.1007/s10853-016-9779-9
- Zhang, J.; Bang, J.H.; Tang, C.; Kamat, P.V. ACS Nano. 2010, 4, 387. doi: 10.1021/nn901087c
- Yu, T.; Hu, W.-l.; Jia, L.; Tan, X.; Huang, J.; Huang, X. Ind. Eng. Chem. Res. 2015, 54, 8193. doi: 10.1021/acs.iecr.5b01903
- Guo, E.; Yin, L. J. Mater. Chem. A. 2015, 3, 13390. doi: 10.1039/C5TA02556G
- Cao, Y.; Zhang, X.; Yang, W.; Du, H.; Bai, Y.; Li, T.; Yao, J. Chem. Mater. 2000, 12, 3445. doi: 10.1021/cm0004432
- Huang, M.; Yu, J.; Li, B.; Deng, C.; Wang, L.; Wu, W.; Dong, L.; Zhang, F.; Fan, M. J. Alloy. Compd. 2015, 629, 55. doi: 10.1016/j.jallcom.2014.11.225
- Li, H.; Xie, C.; Liao, Y.; Liu, Y.; Zou, Z.; Wu, J. J. Alloy. Compd. 2013, 569, 88. doi: 10.1016/j.jallcom.2013.03.126
- Zhou, B.; Yang, S.; Wu, W.; Sun, L.; Lei, M.; Pan, J.; Xiong, X. Cryst. Eng. Comm. 2014, 16, 10863. doi: 10.1039/C4CE01774A
- Wang, C.; Shao, C.; Zhang, X.; Liu, Y. Inorg. Chem. 2009, 48, 7261. doi: 10.1021/ic9005983
- Xie, M.; Meng, Q.; Luan, P.; Feng, Y.; Jing, L. RSC Adv. 2014, 4, 52053. doi: 10.1039/C4RA08451A
- Luan, P.; Xie, M.; Fu, X.; Qu, Y.; Sun, X.; Jing, L. Phys. Chem. Chem. Phys. 2015, 17, 5043. doi: 10.1039/C4CP04631E
- Wang, M.; Pyeon, M.; Gönüllü, Y.; Kaouk, A.; Shen, S.; Guo, L.; Mathur, S. Nanoscale 2015, 7, 10094. doi: 10.1039/C5NR01493J
- Li, X.; Lin, H.; Chen, X.; Niu, H.; Liu, J.; Zhang, T.; Qu, F. Phys. Chem. Chem. Phys. 2016, 18, 9176. doi: 10.1039/C5CP06681F
- Mu, J.; Chen, B.; Zhang, M.; Guo, Z.; Zhang, P.; Zhang, Z.; Sun, Y.; Shao, C.; Liu, Y. ACS Appl. Mater. Inter. 2012, 4, 424. doi: 10.1021/am201499r
- Matsumura, M.; Furukawa, S.J.; Tsubomura, H. J. Phys. Chem. 1985, 89, 1327. doi: 10.1021/j100254a001
- Zhukovskyi, M.; Tongying, P.; Yashan, H.; Wang, Y.; Kuno, M. ACS Catal. 2015, 5, 6615. doi: 10.1021/acscatal.5b01812
- Wang, M.; Jiang, J.; Shi, J.; Guo, L. ACS Appl. Mater. Inter. 2013, 5, 4021. doi: 10.1021/am400851q
- Liu, S.; Zhang, N.; Tang, Z.-R.; Xu, Y.-J. ACS Appl. Mater. Inter. 2012, 4, 6378. doi: 10.1021/am302074p
- Choi, S.K.; Yang, H.S.; Kim, J.H.; Park, H.W. Appl. Catal. B: Environ. 2012, 121–122, 206. doi: 10.1016/j.apcatb.2012.04.011
- Tiwari, A.; Pal, U. Int. J. Hydrogen Energ. 2015, 40, 9069. doi: 10.1016/j.ijhydene.2015.05.101
- Lee, J.; Kwak, J.; Ko, K.C.; Park, J.H.; Ko, J.H.; Park, N.; Kim, E.; Ryu, D.H.; Ahn, T.K.; Lee, J.Y.; Son, S.U. Chem. Commun. 2012, 48, 11431. doi: 10.1039/c2cc36501d
- Bae, E.; Choi, W. J. Phys. Chem. B. 2006, 110, 14792. doi: 10.1021/jp062540+
- Yamashita, H.; Harada, M.; Misaka, J.; Takeuchi, M.; Ikeue, K.; Anpo, M. J. Photoch. Photobio A: Chem. 2002, 148, 257. doi: 10.1016/S1010-6030(02)00051-5
- Takeuchi, M.; Yamashita, H.; Matsuoka, M.; Anpo, M.; Hirao, T.; Itoh, N.; Iwamoto, N. Catal. Lett. 2000, 67, 135. doi: 10.1023/A:1019065521567
- Zhou, J.; Takeuchi, M.; Ray, A.K.; Anpo, M.; Zhao, X.S. J. Colloid Interf. Sci. 2007, 311, 497. doi: 10.1016/j.jcis.2007.03.007
- Lam, R.C.W.; Leunga, M.K.H.; Leunga, D.Y.C.; Vrijmoed, L.L.P.; Yamc, W.C.; Ng, S.P. Sol. Energ. Mat. Sol. C. 2007, 91, 54. doi: 10.1016/j.solmat.2006.07.004
- Wang, C.; Wang, T.; Zheng, S.; Physica E. Physica, E, Low-dimens. Syst. Nanostruct. 2002, 14, 242. doi: 10.1016/S1386-9477(02)00393-4