ABSTRACT
A novel Wells–Dawson heteropolyacid-based magnetic Inorganic–organic nanohybrid, Fe3O4@SiO2@ADMPT/H6P2W18O62, was fabricated and used as a green, efficient, eco-friendly, and highly recyclable catalyst for the one-pot and multi-component synthesis of 1,4-Dihydopyridine (1,4-DHP) derivatives from the reaction of various aromatic aldehydes with ethyl acetoacetate and ammonium acetate with good to excellent yields and in a short span of time. The nanohybrid catalyst was prepared by the chemical anchoring of Wells–Dawson heteropolyacid H6P2W18O62 onto the surface of functionalized Fe3O4 nanoparticles with 2,4-bis(3,5-dimethylpyrazol)-triazine (ADMPT) linker. These nanocatalysts were identified by using scanning electron microscopy (SEM), transmission electron microscopy (TEM), X-ray diffraction (XRD), infrared spectroscopy (IR) and vibrating sample magnetometer (VSM). This protocol is developed as a safe, cost-effective and convenient alternate method for the synthesis of 1,4-DHP derivatives utilizing an eco-friendly, and a highly reusable catalyst.
GRAPHICAL ABSTRACT
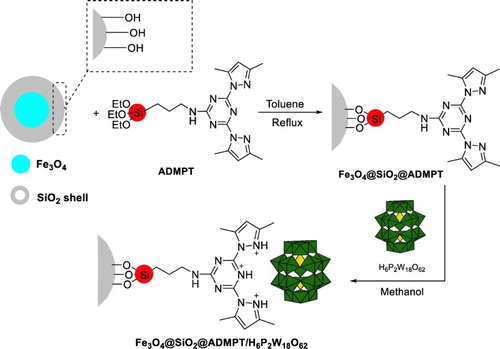
Introduction
Nitrogen-containing heterocycles often play important roles as the scaffolds of biological compounds. Among them, the pyridine infrastructure is one of the most common heterocycles found in functional products, pharmaceuticals, and natural materials ( Citation1,Citation2). In particular, 1 ,4 -Dihydopyridines (1 ,4 -DHPs) have attracted much attention because they include a great family of medicinally active compositions with various therapeutic and pharmacological properties such as MDR reversal ( Citation3), vasodilation ( Citation4), HIV protease inhibition ( Citation5), radioprotection ( Citation6), bronchodilator, antitumor, and also hepatoprotective activities ( Citation7). Moreover, recent studies have shown that these compounds have various medicinal applications such as neuroprotectants, compounds with platelet antiaggregatory, cerebral anti-ischemic agents, and chemosensitizers ( Citation8). Due to their wide range of pharmacological activity and applications, a number of methods have been reported for their synthesis. More than a century ago the first 1,4-DHPs were obtained by Hantzsch multi-component reaction ( Citation9). The classical Hantzsch method includes the one-pot condensation of various aldehydes with ethyl acetoacetate and ammonia either in acetic acid at room temperature or by refluxing in alcohols for a long time ( Citation10). This method suffers from harsh reaction conditions, long reaction times, and very low yields of products.
Therefore, several modified methods have been developed for the synthesis of dihydropyridine derivatives by using conventional heating ( Citation11), ionic liquid ( Citation12), HY-Zeolite ( Citation13), molecular iodine ( Citation14), polymers ( Citation15), cerium(IV) ammonium nitrate ( Citation16), metal triflates ( Citation17), and silica perchloric acid ( Citation18). L-proline and derivatives ( Citation19), montmorillonite K-10 ( Citation20), Ni nanoparticles ( Citation21), P-TSA ( Citation22), and ultrasound and microwave irradiation ( Citation23). Although many of these methods have considerable advantages, some of these methods suffer from the limited scope, such as the use of high temperatures, long reaction times, drastic reaction conditions, environmentally harmful catalysts, difficult work-up procedures, expensive metal precursors, unsatisfactory yields, and a large amount of volatile organic solvents. Moreover, the main disadvantage of most used procedures is that the use of catalyst was destroyed in the work-up process and cannot be reused or recovered.
Nanoparticles have coordination sites and high surface-to-volume ratio, in comparison with other bulk analogs, which provide many of active sites per unit area ( Citation24). Among the different magnetic nanoparticles, Fe3O4 nanoparticles have recently emerged as good supports for the immobilization of core–shell metal nanoparticles and they are certainly the most extensively studied. Due to the potential applications of magnetic Fe3O4 nanoparticles (Fe3O4 MNPs) in catalysis and biomedicine, they have attracted much attention as a significant family of separation materials in chemistry and material sciences ( Citation25). The properties of Fe3O4 nanoparticles such as paramagnetic and insoluble, enable the catalyst to be simply separated from the reaction medium by an external permanent magnet. In general, in order to prepare a suitable surface for the modification of magnetic nanoparticles and also to avoid direct contact between them, a coating of the surface with silica shell is necessary. A silica surface can be functionalized with diverse organic groups for desirable aims such as applications as an adsorbent, enzyme immobilization, and catalysis support ( Citation26). Due to the considerable properties of Fe3O4 MNPs like greater selectivity, operational simplicity, noncorrosive nature, environmentally benign, moisture insensitive, and ease of isolation, they are used as an efficient catalyst in many organic reactions.
Heteropoly compounds are useful and versatile in organic transformations because of their super acidic and redox properties ( Citation27). Among them, heteropolyacids (HPAs) are more active catalysts than conventional mineral acids. The use of HPAs as catalysts is an area of interest in catalysis, which has been studied recently. In addition to the problems related to the environmental and potential hazards of mineral acids and their handling and disposal, the chemist’s attention has been attracted to the development of alternative processes using solid acid catalysts ( Citation28). Because the HPAs have many advantages in comparison to conventional inorganic acids and are environmentally and economically attractive in both industrial and academic settings, these compounds provide green alternatives to homogeneous catalysts in the industrial manufacture of chemicals and in organic reactions ( Citation29). They also have many advantages over homogeneous liquid acid catalysts: higher thermal stabilities and acid strengths; they are cheap, noncorrosive, reusable, and environmentally benign and need less waste disposal ( Citation30). The major disadvantages of HPAs such as high solubility in aqueous solution, low surface area, and continuous leakage during operation limit the scope of their practical applications. To overcome these limitations, heterogenization of the heteropolyacid through immobilization onto supports, with a high surface area, is recommended. Among the HPAs, the Wells–Dawson heteropolyacids (WD HPAs) have super-acidity and a considerable stability both in solid and in the solution state ( Citation31). The structure of the WD HPAs (H6P2W18O62. 24H2O) including a close-packed structure of octahedral WO6 surrounding a central P atom, two same “half units” PW9 are linked through the oxygen atoms ( Citation32). Because of the distinctive features of the WD HPAs, such as higher reactivity and greater selectivity in acid catalyzed reactions, they had been the target of many research groups during the last years ( Citation33–35).
As part of our current studies on the development of efficient methods for the preparation of biologically active compounds ( Citation36–44), in this paper, Fe3O4@SiO2@ADMPT/H6P2W18O62 as a new inorganic–organic hybrid nanocatalyst was prepared, characterized, and used as an effective catalyst in the synthesis of 1,4-DHPs 4 via one-pot reaction of various aromatic aldehydes 1, ethyl acetoacetate 2, and ammonium acetate 3 with good to high yield in ethanol (Scheme 1). To the best of our knowledge, there are no examples of the use of WD HPAs as the catalyst for the synthesis of 1,4-DHP derivatives.
Experimental
Materials and instrumentation
All starting materials and reagents were obtained from commercial sources and were used without further purification. All solvents were dried and distilled under a nitrogen atmosphere, within standard methods. The progress of the reactions was monitored by thin-layer chromatography (TLC) on silica gel 60 F254 plates. Fourier transform infrared (FT-IR) spectra from 250 to 4000 cm−1 were registered using a Perkin-Elmer 781 FT-IR spectrometer, using KBr pellets. NMR spectra were determined on a Bruker DRX-400-Advance and Bruker DRX-300-Advance instrument using DMSO-d6 or CDCl3 as the solvent with TMS as the internal standard. The morphology and size of the nanostructures was observed on an XL-30 scanning electron microscope (SEM) (Philips, Netherlands) and on an EM 10C Transmission Electron Microscope (TEM). X-ray powder diffraction analysis (XRD) measurements were obtained on a STADI P diffractometer (STOE, Germany) using Cu Kα radiation with a scanning rate of 3° min−1 in the 2θ range between 10° and 80°. Melting points were measured by using the capillary tube method with a Yanagimoto micro melting point apparatus. Magnetic susceptibility measurements were carried out using a vibrating sample magnetometer (VSM, Meghnatis Daghigh Kavir Company, Iran) in the magnetic field at room temperature. The preparation and characterization of the heteropolyacid catalyst H6P2W18O62, was performed following the previously reported procedures ( Citation45).
Synthesis
Synthesis of 2,4-bis(3,5-dimethylpyrazol)-triazine (5)
The 2-chloro-4,6-bis(3,5-dimethyl-1H-pyrazol-1-yl)-1,3,5-triazine 5 was prepared according to our previous reports ( Citation46,Citation47). Briefly, 0.38 g of 3,5-dimethylpyrazol (4.0 mmol) in dry toluene (10 mL) was added dropwise to a solution of N,N-diisopropylethylamine (DIPEA) (0.68 mL, 4.0 mmol) and 0.37 g of cyanuric chloride (2.0 mmol) in 40 mL dry toluene at room temperature under nitrogen atmosphere. The reaction mixture was allowed to stir at room temperature overnight. Upon completion (monitoring by TLC), the toluene was removed under reduced pressure, then water was added, and the reaction mixture was stirred for 30 min. The precipitate was filtered, washed with water, air-dried, and was purified by column chromatography. The 2-chloro-4,6-bis(3,5-dimethyl-1H-pyrazol-1-yl)-1,3,5-triazine 5 was obtained as a white solid (Yield 76%). M.p. 146–149°C; 1H NMR (CDCl3, 300 MHz): δ = 2.35 (6H, s, CH3), 2.76 (6H, s, CH3), 6.12 (2H, s, CH).
Synthesis of 2- APTS -4,6-bis(3,5-dimethyl-1H-pyrazol-1-yl)-1,3,5-triazine (ADMPT)
ADMPT was prepared according to our previous reports ( Citation46,Citation47). Briefly, 0.60 g of 2-chloro-4,6-bis(3,5-dimethyl-1H-pyrazol-1-yl)-1,3,5-triazine (2.0 mmol) and DIPEA (0.34 mL, 2.0 mmol) were dissolved in dry toluene (40 mL) under nitrogen atmosphere, and these mixture was heated to 80°C. Then, 0.51 mL of 3-aminopropyltriethoxysilane (APTES) (2.2 mmol) was added dropwise and the reaction mixture was stirred at 80°C. After 48 h, the solvent was removed under reduced pressure, and the product was purified by column chromatography (yield 68%, Scheme 2). M.p. 101–103°C; 1H NMR (CDCl3, 300 MHz): δ = 0.68 (2H, m, CH2), 1.22 (9H, t, J = 7.2 Hz, CH3), 1.74 (2H, m, CH2), 2.31 (6H, s, CH3), 2.70 (3H, s, CH3), 2.77 (3H, s, CH3), 3.53 (2H, m, CH2), 3.82 (6H, q, J = 6.9 Hz, CH2), 6.03 (2H, s, CH).
Synthesis of Fe3O4 and Fe3O4@SiO2
Fe3O4 magnetic nanoparticles were prepared according to a previous report ( Citation48). Briefly, FeCl3.6H2O (10.4 g) and FeCl2.4H2O (4.0 g) were dissolved in 100 mL of deionized water, degassed with nitrogen gas for 15 min, and heated to 80°C. Then, 15 mL of NH3 (32% solution) was added dropwise to the solution. After 15 min, the solid was separated by a magnet and washed three times with water, acetone and dichloromethane.
The interlayers of SiO2 were prepared through a modified Stober method ( Citation49). 1.5 g Fe3O4 particles were dispersed in a mixture of deionized water (30 mL), ethanol (120 mL) and concentrated ammonia aqueous solution (25 wt%, 3.8 ml) by ultrasonication for 15 min. Then, 5 mL of tetraethyl orthosilicate (TEOS) was added dropwise. After stirring for 12 h, the products were collected and washed by deionized water and methanol to eliminate excess reactants and then collected by an external magnet. Finally, the Fe3O4@SiO2 particles were dried at room temperature for 24 h.
Functionalizing of silica-coated magnetite nanoparticles Fe3O4@SiO2 with ADMPT (Fe3O4@SiO2@ADMPT)
For the synthesis of triazine-functionalized Fe3O4@SiO2, in the first step, 0.50 g of the Fe3O4@SiO2 was suspended in dry toluene (50 ml). Then, 1.46 g of ADMPT was added and refluxed for 48 h. After this time, the dark solid was removed from the solvent by a strong magnet, washed with deionized water and ethanol, and subsequently dried at room temperature. FT-IR spectroscopy showed ADMPT anchored onto Fe3O4@SiO2. A schematic diagram for the synthesis of Fe3O4@SiO2@ADMPT is shown in Scheme 2.
Immobilization of Wells–Dawson heteropolyacid on Fe3O4@SiO2@ADMPT (Fe3O4@SiO2@ADMPT/HPA)
For the immobilization of H6P2W18O62 on Fe3O4@SiO2@ADMPT, 1.0 g of Fe3O4@SiO2@ADMPT was added to the suspension of 0.6 g of H6P2W18O62 in methanol (50 ml) and the reaction mixture was refluxed for 4 h. Then, the heterogeneous catalyst was separated by an external magnetic field and extracted by using methanol as a solvent in a Soxhlet extractor. After overnight, catalyst was dried at room temperature. A schematic for the preparation of Fe3O4@SiO2@ADMPT/H6P2W18O62 nanocatalyst is shown in Scheme 3. In first step, the scheme shows grafting of ADMPT onto Fe3O4@SiO2 via condensation of ethoxy and hydroxyl groups of the linker and support, respectively; then, the H6P2W18O62 was anchored to Fe3O4@SiO2@ADMPT through electrostatic interaction in the second step.
General procedure for the synthesis of 1,4-DHPs by using Fe3O4@SiO2@ADMPT/H6P2W18O62 nanocatalyst
First, a mixture of aromatic aldehyde 1 (1.0 mmol), ethyl acetoacetate 2 (2.0 mmol), ammonium acetate 3 (1.2 mmol) and 5 mL ethanol in the presence of 20 mg of the Fe3O4@SiO2@ADMPT/H6P2W18O62 nanocatalyst were mixed thoroughly. The mixture was heated at 70°C with concomitant stirring for appropriate time (). After completion of the reaction confirmed by TLC (eluent: n-hexane/EtOAc; 8:2), solid catalyst was separated by an external magnet and the solution was cooled to room temperature and extracted with 15 ml water and dichloromethane (3 × 10 mL) and dried with anhydrous Na2SO4, then the products were purified by recrystallized from ethanol, gave the pure products in 87–98% yields based on the starting aldehyde. The products were characterized by IR, 1H NMR and via comparison of their melting points with the previous reported.
Results and discussion
Structural characterization of Fe3O4@SiO2@ADMPT/H6P2W18O62 nanocatalyst
FT-IR spectroscopy
The Wells–Dawson structure of H6P2W18O62 consists of two half units composed of a central tetrahedral PO4 surrounded by nine octahedral WO6. Therefore, the H6P2W18O62 structure involves four kinds of oxygen atoms. The first is P–O in which the oxygen atom connects with the tungsten atom, the second is W–O–W oxygen bridges (corner-sharing oxygen bridges between different W3O13 groups), the third is W–O–W oxygen bridges (edge-sharing oxygen bridge within W3O13 groups), and the last is W = O terminal oxygen atoms. Therefore, the Wells–Dawson heteropolyacid has four characteristic bands in the range of 750–1100 cm−1 ( Citation50). The absorption bands around 950–998 and 1070–1100 cm−1 are related to W = O and P–O bonds, respectively. The vibration bands at 912 and 776 cm−1 were assigned to the “inter” and “intra” W–O–W bridges, respectively ().
ADMPT was used to modify the Fe3O4@SiO2 nanoparticles in refluxing toluene. The triazine-functionalized magnetic nanoparticles have been synthesized by direct reaction of the surface hydroxyl groups of Fe3O4@SiO2 and the ethoxy groups of ADMPT. A schematic diagram for this synthesis route is illustrated in Scheme 2. shows the FT-IR spectrums of Fe3O4@SiO2@ADMPT/H6P2W18O62 in comparison with Fe3O4, Fe3O4@SiO2 and Fe3O4@SiO2@ADMPT.
Fe3O4 nanoparticles were synthesized according to the co-precipitation method, and their formation was confirmed by IR spectroscopy (). The strong and broad band that observed at 569 cm−1 is ascribed to the stretching vibration of Fe–O bonds of Fe3O4 nanoparticles. The broad band in the 3200–3500 cm−1 regions is attributed to the symmetric stretching vibration mode of the adsorbed water molecules. shows the IR spectrum of the Fe3O4@SiO2 sample. In addition to the previous bands, there is a new strong band around 1078 cm−1 that originates from the Si–O bond of silica and other bond around 633 cm−1 is indicated to the Si–O–Fe in the Fe3O4@SiO2 sample ( Citation51). This result indicates that SiO2 is immobilized on the surfaces of Fe3O4 nanoparticles. indicated grafting of ADMPT group on the surface of Fe3O4@SiO2 sample. A medium broad band appeared in the range of 2878–2976 cm−1 (centered at 2926 cm−1), mainly attributed to the C–H stretching vibration, and confirmed graft of ADMPT on the Fe3O4@SiO2 sample surface. In addition, the appearance of bands at 1538, 1078, and 1603 cm−1 which attributed to the NH bending, the Si–O and the C = N stretching vibrations, respectively, supports the above conclusion. However, the characteristic band at 3264 cm−1 for the NH stretching vibration of the ADMPT is covered by the broad stretching vibration band of the adsorbed water molecules in the 3100–3500 cm−1 regions. The Wells–Dawson heteropolyacid has four characteristic bands in the range of 750–1100 cm−1 ( Citation50). The absorption bands around 950–998 and 1070–1100 cm−1 are related to W = O and P–O bonds, respectively. The vibration bands at 912 and 776 cm−1 were assigned to the “inter” and “intra” W–O–W bridges, respectively (). The new absorption bands in the fingerprinting IR bands of Fe3O4@SiO2@ADMPT/HPA () confirm that loading of H6P2W18O62 was occurred over the Fe3O4@SiO2@ADMPT and H6P2W18O62 was successfully immobilized on the surface of Fe3O4@SiO2@ADMPT support through strong chemical interaction.
X-ray diffraction study
The crystalline structures of the Fe3O4 nanoparticles and magnetic hybrids were determined by powder X-ray diffraction (XRD). As it can be seen in , the patterns show peaks at 2θ = 30.06, 35.45, 43.2, 53.54, 57.16, 62.72, and 73.99, which correspond to d220, d311, d400, d422, d511, d440, and d533, respectively, and confirms that the Fe3O4 structure has remained intact after functionalization by ADMPT and Immobilization of Wells–Dawson heteropolyacid on Fe3O4@SiO2@ADMPT did not decompose or convert to Fe2O3. Furthermore, the broad peaks in XRD pattern show that the Fe3O4 particles are nanosize. The diameter of magnetite nanoparticles was also determined from X-ray line broadening using the Scherrer formula (D = 0.9λ/βcosθ, where D is the average crystalline size, λ is the X-ray wavelength used, β is the angular line width at half maximum intensity, and θ is the Bragg’s angle). For the (311) reflection the average crystalline size of the Fe3O4 NPs was obtained to be around 13 nm.
VSM analysis
The magnetic properties of the hybrids consisting a magnetite component were demonstrated using a VSM. shows the magnetic hysteresis loops of the Fe3O4, Fe3O4@SiO2, Fe3O4@SiO2@ADMPT, and Fe3O4@SiO2@ADMPT/HPA nanocatalyst. The magnetization curves show that all the samples are superparamagnetic behavior at room temperature. The magnetic saturation (Ms) values for Fe3O4, Fe3O4@SiO2, Fe3O4@SiO2@ADMPT, and Fe3O4@SiO2@ADMPT/HPA are 57.36, 35.20, 27.77, and 19.53 emu/g, respectively. It is noticed that the magnetization was decreased considerably when the coated groups of the surface of the Fe3O4@SiO2 are increase. These results indicated that all of these samples have strong magnetic responsivity and can be separated easily from the solution with an external magnetic field.
SEM and TEM study
The size and morphology details of the nanocatalyst were achieved by SEM measurement (). The SEM image of Fe3O4@SiO2@ADMPT/H6P2W18O62 indicated that average size of the Fe3O4@SiO2@ADMPT/H6P2W18O62 is around 81–89 nm. The TEM image of Fe3O4@SiO2@ADMPT/H6P2W18O62 has been shown in and indicates that the Fe3O4@SiO2 composites made as supports for the catalyst had a regular core–shell structures and good spherical morphologies.
Investigation of the catalyst activity
In this work, we have synthesized 1,4-DHPs 4 via one-pot, the three-component reaction of aromatic aldehydes 1, ethyl acetoacetate 2, and ammonium acetate 3 in the presence of catalytic amounts of the Fe3O4@SiO2@ADMPT/H6P2W18O62 nanocatalyst in ethanol at 70°C.
Effect of catalyst concentration on the catalytic efficiency
At the outset of our work, to optimize the reaction conditions, a model reaction was carried out by starting from benzaldehyde 1a (1 mmol), ethyl acetoacetate 2 (2 mmol), and ammonium acetate 3 (1.2 mmol) in the presence of catalytic amounts of the Fe3O4@SiO2@ADMPT/H6P2W18O62 nanocatalyst in ethanol at 70°C (Scheme 4).
First, the catalytic efficiency of the Fe3O4@SiO2@ADMPT/H6P2W18O62 nanocatalyst was investigated for the model reaction with different amounts of catalyst (). To establish the real effectiveness of the catalyst, we studied the model reaction by using 15–50 mg of Fe3O4@SiO2@ADMPT/H6P2W18O62 nanocatalyst. These results showed that the higher yield was obtained with 20 mg of Fe3O4@SiO2@ADMPT/H6P2W18O62 as a catalyst (, entry 2). Therefore, 20 mg of catalyst was found to be the optimal amount and sufficient to produce the best yield of products. As can be seen from , by decreasing and increasing the amount of nanocatalyst, the yield of the product was not improved (entry 1, 3, and 4, respectively).
Table 1. Effect of the catalyst amount for the Hantzsch synthesis of 1,4-DHP 4a.a
also compares the catalytic activity of Fe3O4@SiO2@ADMPT/H6P2W18O62 nanocatalyst with H6P2W18O62, Fe3O4@SiO2@ADMPT, and Fe3O4@SiO2, separately, for the preparation of 1,4-DHP 4a under the optimized reaction conditions (entry 5–7). As can be seen, 5 mg of H6P2W18O62 catalyst led to 55% yield of the desired product after 300 min (entry 5); whereas, 20 mg of the Fe3O4@SiO2@ADMPT/H6P2W18O62 nanocatalyst, which includes the same amount of H6P2W18O62 (5 mg), produced 92% yield of product after a short time of 35 min (entry 2). The triazine-functionalized Fe3O4@SiO2, Fe3O4@SiO2@ADMPT, and Fe3O4@SiO2 were also catalytically active; the results showed that the Fe3O4@SiO2@ADMPT led to 33% of product after 420 min and the Fe3O4@SiO2 produced 21% of product after 600 min (entries 6 and 7, respectively). Although the components of the nanohybrid showed trace catalytic activity, Fe3O4@SiO2@ADMPT/H6P2W18O62 gave the best yield in the desired organic synthesis (entry 2). Clearly, grafting the heteropolyacid onto the modified solid material Fe3O4@SiO2@ADMPT drastically increased its catalytic activity toward the organic reaction.
Effect of reaction media on the catalytic efficiency
For optimizing the reaction conditions, we examined the model reaction in the presence 20 mg of Fe3O4@SiO2@ADMPT/H6P2W18O62 as a nanomagnetic catalyst in various solvents at 70°C (). Solvents such as water, ethanol were screened in the desired reaction. As shown in , 1,4-DHP 4a was formed in all cases, but the highest yield for these product was achieved in ethanol (Entries 2 and 3). We prefer to use ethanol as a green solvent in these reactions.
Table 2. Effect of different solvents on the synthesis of 1,4-DHP 4a.a
Effect of reaction time on the catalytic efficiency
shows the yield of 1,4-DHP 4a in the presence of Fe3O4@SiO2@ADMPT/HPA nanocatalyst (20 mg) in various times at 70°C. As indicated in , only 35 min is sufficient to get 92% yield of this product. No obvious increase was detected in the yield after a prolonged reaction time.
Preparation of 1,4-DHP derivatives in the presence of Fe3O4@SiO2@ADMPT/H6P2W18O62 nanocatalyst
To evaluate the generality of the present protocol for the synthesis of 1,4-DHPs 4, we investigated the reaction by using a wide range of diverse aromatic aldehydes carrying either electron-donating, electron-withdrawing, and halogen groups on their aromatic rings under optimized conditions and gave the corresponding products in best yields in short reaction times based on the Hantzsch procedure (). As shown in , the nature of aromatic aldehyde has significant effect on these reaction; when the electron-withdrawing substituents are present in benzaldehyde, the reaction rate increases, whereas the effect is the reverse in the case of benzaldehyde with strong electron-donating substituents such as –OCH3 and –OH, of course with lower yields. The structures of all the products were characterized by FT-IR and 1H NMR spectral analysis and their melting points.
Table 3. Synthesis of 1,4-DHP derivatives 4 via one-pot, three-component reaction in the presence of Fe3O4@SiO2@ADMPT/H6P2W18O62 nanocatalyst in EtOH at 70°C.a
Reusability of the catalyst
From industrial point of view, the reusability of the catalyst is very important in the large scale synthetic processes. A major drawback of free heteropolyacid is the separation and recovery of the catalyst after completion the reaction. Heterogenization of the H6P2W18O62 via anchoring to Fe3O4@SiO2@ADMPT is an attractive strategy that allowing simple separation of the catalyst from the reaction mixture. Moreover, recyclability and reusability of the catalyst reduce amount of wastage and production of wasteful materials; both are important factors in green chemistry. To investigate the reusability of the Fe3O4@SiO2@ADMPT/H6P2W18O62 nanocatalyst, after completion the reaction, it was easily separated from the products by an external magnet and washed thoroughly with chloroform to remove undesired materials, dried, and activated to reuse for another condensation reaction. As illustrated in , it revealed nearly no loss of activity after five successive runs. The results showed that the catalyst could be a satisfactory catalyst for this reaction with good reusability and high activity.
Comparison of the catalytic efficiency of Fe3O4@SiO2@ADMPT/H6P2W18O62 nanocatalyst with reported different catalytic systems
In order to explore the merit of the present method in comparison with other literature methods for the synthesis of the 1,4-DHP compounds, the reaction of benzaldehyde 1a with ethyl acetoacetate 2, and ammonium acetate 3 for the synthesis of the corresponding product were selected as model reaction and the comparison was in terms of solvent, temperature, reaction time, and percentage yields (). Obviously, the Fe3O4@SiO2@ADMPT/H6P2W18O62, novel magnetic inorganic–organic nanocatalyst, is a more efficient catalyst with respect to time, yield, temperature, and solvent than other literature reported catalysts.
Table 4. Comparison of the catalytic efficiency of Fe3O4@SiO2@ADMPT/H6P2W18O62 nanocatalyst with reported different catalytic systems for the synthesis of 1,4-DHP 4a.
Proposed reaction pathway for the catalytic system
A tentative mechanism for the synthesis of 1,4-DHPs catalyzed by Fe3O4@SiO2@ADMPT/H6P2W18O62 nanocatalyst is proposed in Scheme 5. In step I, the ammonium acetate was converted to the ammonia and acetic acid. Then, the ammonia as a base was produced the 4-ethoxy-4-hydroxybut-3-en-2-one (A). In step II, the formation of product B as an intermediate can be performed via the standard Knoevenagel condensation by the nucleophilic addition of A component to the carbonyl group of the aldehyde which was activated by the Fe3O4@SiO2@ADMPT/H6P2W18O62 nanocatalyst. Another key intermediate is ester enamine C, produced by condensation of ammonia with the second equivalent of the ethyl acetoacetate which was activated by the Fe3O4@SiO2@ADMPT/H6P2W18O62 nanocatalyst. In step III, the A and B intermediates were reacted via Michael addition to afford the intermediate D. Finally, Intramolecular cyclization of enamine D by the nucleophilic addition of enamine to the carbonyl group which was activated by the nanocatalyst was produced the target product 1,4-DHP 4a.
Conclusion
This work has explained the preparation of functionalized magnetic nanomaterials by reacting 2,4-bis(3,5-dimethylpyrazol)-triazine (ADMPT) modified silica-coated magnetite nanoparticles with Wells–Dawson diphosphooctadecatungstic acid (H6P2W18O62·24H2O) and fabrication of a novel organic – inorganic nanohybrid catalyst has been the target of this research. This catalytic system as a straightforward and efficient method was used in an environmentally friendly route to prepare a variety of 1,4-dihydropyridines from the reaction of different aryl aldehydes, ethyl acetoacetate, and ammonium acetate. Compared with a homogenous catalyst, easy separation by magnet was a valuable advantage that means low costs in industrial applications. Magnetic recyclability, mild reaction conditions, and environmental friendliness may be its superior characteristics for green chemistry. Moreover, the advantages such as excellent product yields, shorter reaction times, simplicity of the reaction, the use of non-toxic and inexpensive materials, the easy procedures to carry out the reaction make this protocol as an attractive and useful methodology, among the other methods reported in the literature, for the synthesis of 1,4-DHPs.
Supplementary_Material.docx
Download MS Word (3.7 MB)Disclosure statement
No potential conflict of interest was reported by the authors.
Notes on contributors
Mohammad Ghanbari graduated with the Ph.D. degree in Organic Chemistry from the University of Tehran, Iran (2012). In 2013, he was appointed as assistant professor in the Department of Organic Chemistry at the University of Kashan. His research program is based on synthetic organic and organometallic chemistry. His principal focus of research is the development and application of new catalytic methods for the synthesis of bioactive compounds.
Sanaz Moradi received her B.Sc. degree in Chemistry from Vali-e-Asr University of Rafsanjan, in 2013 and her M.Sc. degree in Organic Chemistry from the University of Kashan (Kashan, Iran) in 2017.
Moslem Setoodehkhah received his B.Sc. degree in Chemistry from Persian Gulf University (Bushehr, Iran) in 2001 and his M.Sc. degree in Inorganic chemistry from the Shiraz University (Shiraz, Iran) in 2004. He received his Ph.D. degree in Inorganic Chemistry from the Shiraz University in 2011. He joined the Department of Inorganic Chemistry at the University of Kashan in 2013. His research interest includes the Synthesis and application of Schiff bases, magnetic core-shell nano-structures as well as nano-particles.
ORCID
Mohammad Ghanbari http://orcid.org/0000-0003-2435-1860
Additional information
Funding
References
- Henry, G.D. De Novo Synthesis of Substituted Pyridines. Tetrahedron 2004, 60 (29), 6043–6061.
- Cocco, M.T.; Congiu, C.; Lilliu, V.; Onnis, V. Synthesis and Antiproliferative Activity of 2, 6-Dibenzylamino-3, 5-Dicyanopyridines on Human Cancer Cell Lines. Euro J Med Chem 2005, 40 (12), 1365–1372.
- Boumendjel, A.; Baubichon-Cortay, H.; Trompier, D.; Perrotton, T.; Di Pietro, A. Anticancer Multidrug Resistance Mediated by MRP1: Recent Advances in the Discovery of Reversal Agents. Med Re Re 2005, 25 (4), 453–472.
- Atwal, K.S.; Swanson, B.N.; Unger, S.E.; Floyd, D.M.; Moreland, S.; Hedberg, A.; O’Reilly, B.C. Dihydropyrimidine Calcium Channel Blockers. 3. 3-Carbamoyl-4-Aryl-1, 2, 3, 4-Tetrahydro-6-Methyl-5-Pyrimidinecarboxylic Acid Esters as Orally Effective Antihypertensive Agents. J Me Chem 1991, 34 (2), 806–811.
- Hilgeroth, A. Dimeric 4-Aryl-1, 4-Dihydropyridines: Development of a Third Class of Nonpeptidic HIV-1 Protease Inhibitors. Mini Revn Me Chem 2002, 2 (3), 235–245.
- Donkor, I.O.; Zhou, X.; Schmidt, J.; Agrawal, K.C.; Kishore, V. Synthesis and Radioprotective Effects of Adamantyl Substituted 1, 4-Dihydropyridine Derivatives. Bioorganic & Me Chem 1998, 6 (5), 563–568.
- Atwal, K.S.; Rovnyak, G.C.; Kimball, S.D.; Floyd, D.M.; Moreland, S.; Swanson, B.N.; Gougoutas, J.Z.; Schwartz, J.; Smillie, K.M.; Malley, M.F. Dihydropyrimidine Calcium Channel Blockers. II. 3-Substituted-4-Aryl-1, 4-Dihydro-6-Methyl-5-Pyrimidinecarboxylic Acid Esters as Potent Mimics of Dihydropyridines. J Me Chem 1990, 33 (9), 2629–2635.
- Boer, R.; Gekeler, V. Chemosensitizers in Tumor Therapy: new Compounds Promise Better Efficacy. Drugs Future 1995, 20 (5), 499–510.
- Hantzsch, A. Ueber die Synthese Pyridinartiger Verbindungen aus Acetessigäther und Aldehydammoniak. Eur J Org Chem 1882, 215 (1), 1–82.
- Loev, B.; Snader, K.M. The Hantzsch Reaction. I. Oxidative Dealkylation of Certain Dihydropyridines. J Org Chem 1965, 30 (6), 1914–1916.
- Suárez, M.; Ochoa, E.; Verdecia, Y.; Pita, B.; Morán, L.; Martín, N.; Quinteiro, M.; Seoane, C.; Soto, J.; Novoa, H. A Joint Experimental and Theoretical Structural Study of Novel Substituted 2, 5-Dioxo-1, 2, 3, 4, 5, 6, 7, 8-Octahydroquinolines. Tetrahedron 1999, 55 (3), 875–884.
- Zhang, X.-Y.; Li, Y.-Z.; Fan, X.-S.; Qu, G.-R.; Hu, X.-Y.; Wang, J.-J. Multicomponent Reaction in Ionic Liquid: A Novel and Green Synthesis of 1, 4-Dihydropyridme Derivatives. Chin Chem Lett 2006, 17 (2), 150–152.
- Sabitha, G.; Reddy, G.K.K.; Reddy, C.S.; Yadav, J. A Novel TMSI-Mediated Synthesis of Hantzsch 1, 4-Dihydropyridines at Ambient Temperature. Tetrahedron Lett 2003, 44 (21), 4129–4131.
- Kumar, S.; Sharma, P.; Kapoor, K.K.; Hundal, M.S. An Efficient, Catalyst-and Solvent-Free, Four-Component, and one-pot Synthesis of Polyhydroquinolines on Grinding. Tetrahedron 2008, 64 (3), 536–542.
- Das, B.; Ravikanth, B.; Ramu, R.; Rao, B.V. An Efficient one-pot Synthesis of Polyhydroquinolines at Room Temperature Using HY-Zeolite. Chem Pharmaceutical Bulletin 2006, 54 (7), 1044–1045.
- Dondoni, A.; Massi, A.; Minghini, E.; Bertolasi, V. Multicomponent Hantzsch Cyclocondensation as a Route to Highly Functionalized 2-and 4-Dihydropyridylalanines, 2-and 4-Pyridylalanines, and Their N-Oxides: Preparation via a Polymer-Assisted Solution-Phase Approach. Tetrahedron 2004, 60 (10), 2311–2326.
- Reddy, C.S.; Raghu, M. Cerium (IV) Ammonium Nitrate Catalysed Facile and Efficient Synthesis of Polyhydroquinoline Derivatives Through Hantzsch Multicomponent Condensation. Chin Chem Lett 2008, 19 (7), 775–779.
- Song, G.; Wang, B.; Wu, X.; Kang, Y.; Yang, L. Montmorillonite K10 Clay: An Effective Solid Catalyst for one-pot Synthesis of Polyhydroquinoline Derivatives. Synthetic Commun 2005, 35 (22), 2875–2880.
- Maheswara, M.; Siddaiah, V.; Damu, G.L.V.; Rao, C.V. An Efficient one-pot Synthesis of Polyhydroquinoline Derivatives via Hantzsch Condensation Using a Heterogeneous Catalyst Under Solvent-Free Conditions. Arkivoc 2006, 2, 201–206.
- Cherkupally, S.R.; Mekala, R. P-TSA Catalyzed Facile and Efficient Synthesis of Polyhydroquinoline Derivatives Through Hantzsch Multi-Component Condensation. Chem Pharm Bull 2008, 56 (7), 1002–1004.
- Sapkal, S.B.; Shelke, K.F.; Shingate, B.B.; Shingare, M.S. Nickel Nanoparticle-Catalyzed Facile and Efficient one-pot Synthesis of Polyhydroquinoline Derivatives via Hantzsch Condensation Under Solvent-Free Conditions. Tetrahedron Lett 2009, 50 (15), 1754–1756.
- Singh, S.K.; Singh, K.N. Glycine-Catalyzed Easy and Efficient One-pot Synthesis of Polyhydroquinolines Through Hantzsch Multicomponent Condensation Under Controlled Microwave. J Heterocycl Chem 2010, 47 (1), 194–198.
- Li, M.; Zuo, Z.; Wen, L.; Wang, S. Microwave-assisted Combinatorial Synthesis of Hexa-Substituted 1, 4-Dihydropyridines Scaffolds Using one-pot two-Step Multicomponent Reaction Followed by a S-Alkylation. J Comb Chem 2008, 10 (3), 436–441.
- Min, Y.; Akbulut, M.; Kristiansen, K.; Golan, Y.; Israelachvili, J. The Role of Interparticle and External Forces in Nanoparticle Assembly. Nature Materials 2008, 7 (7), 527–538.
- Gao, J.; Zhang, W.; Huang, P.; Zhang, B.; Zhang, X.; Xu, B. Intracellular Spatial Control of Fluorescent Magnetic Nanoparticles. J Am Chem Soc 2008, 130 (12), 3710–3711.
- Masteri-Farahani, M.; Movassagh, J.; Taghavi, F.; Eghbali, P.; Salimi, F. Magnetite–Polyoxometalate Hybrid Nanomaterials: Synthesis and Characterization. Chem Eng J 2012, 184, 342–346. doi:10.1016/j.cej.2011.12.094.
- Kozhevnikov, I.V. Catalysis by Heteropoly Acids and Multicomponent Polyoxometalates in Liquid-Phase Reactions. Chem Rev 1998, 98 (1), 171–198.
- Romanelli, G.; Autino, J.; Baronetti, G.; Thomas, H. A Fast and Efficient Deprotection of Aldehydes from Acylals Using a Wells-Dawson Heteropolyacid Catalyst (H6P2W18O62· 24H2O). Synthetic Commun 2004, 34 (21), 3909–3914.
- Corma, A.; García, H. Lewis Acids: From Conventional Homogeneous to Green Homogeneous and Heterogeneous Catalysis. Chem Rev 2003, 103 (11), 4307–4366.
- Okuhara, T.; Mizuno, N.; Misono, M. Catalytic Chemistry of Heteropoly Compounds. Adv Catal 1996, 41, 113–252.
- Valle, G.M.; Briand, L.E. Stability of Phospho-Molybdic Wells–Dawson-Type ion P 2 Mo 18 O 62 6− in Organic Media. Mater Lett 2003, 57 (24), 3964–3969.
- Baronetti, G.; Briand, L.; Sedran, U.; Thomas, H. Heteropolyacid-based Catalysis. Dawson Acid for MTBE Synthesis in gas Phase. Appl Catal, A 1998, 172 (2), 265–272.
- Romanelli, G.P.; Autino, J.C. Recent Applications of Heteropolyacids and Related Compounds in Heterocycles Synthesis. Mini-Rev Org Chem 2009, 6 (4), 359–366.
- Sambeth, J.E.; Romanelli, G.; Autino, J.C.; Thomas, H.J.; Baronetti, G.T. A Theoretical–Experimental Study of Wells-Dawson Phospho-Tungstic Heteropolyacid: An Explanation of the Pseudoliquid or Surface-Type Behaviour. Appl Catal, A 2010, 378 (1), 114–118.
- G Sathicq, A.; P Romanelli, G.; Ponzinibbio, A.; T Baronetti, G.; J Thomas, H. An Efficient One-Step Hantzsch Multicomponent Synthesis of 1, 4-Dihydropyridines Via a Wells-Dawson Heteropolyacid Catalyst Under Solvent-Free Conditions. Lett Org Chem 2010, 7 (7), 511–518.
- Ghanbari, M.; Jadidi, K.; Mehrdad, M.; Assempour, N. A Simple Route for the Synthesis of Novel 1, 4-Benzoxazine Derivatives by Baeyer–Villiger Oxidation Reaction. Tetrahedron 2016, 72 (29), 4355–4360.
- Ghanbari, M.; Kianmehr, E.; Behzad, S.K.; Ng, S.W. Direct Synthesis of Benzo [a] Carbazoles by Palladium-Catalyzed Domino Reactions: Synthesis and Photophysical Properties of Diverse Benzo [a] Carbazoles. J Iran Chem Soc 2016, 13 (1), 7–18.
- Hashmi, A.S.K.; Ghanbari, M.; Rudolph, M.; Rominger, F. Combining Gold and Palladium Catalysis: One-Pot Access to Pentasubstituted Arenes From Furan–Yne and En–Diyne Substrates. Chem–A Euro J 2012, 18 (26), 8113–8119.
- Jadidi, K.; Ghahremanzadeh, R.; Mehrdad, M.; Ghanbari, M.; Arvin-Nezhad, H. A Simple Indirect Route for the Synthesis of N-Alkyl-4-Imino-1, 4-Dihydro-2H-3, 1-Benzoxazin-2-Ones. Monatshefte für Chemie-Chemical Monthly 2008, 139 (3), 277–280.
- Kianmehr, E.; Ghanbari, M.; Faghih, N.; Rominger, F. A Palladium-Catalyzed one-pot Procedure for the Regioselective Dimerization and Cyanation of Indoles. Tetrahedron Lett 2012, 53 (15), 1900–1904.
- Kianmehr, E.; Ghanbari, M.; Niri, M.N.; Faramarzi, R. Novel One-Pot Three Component Reaction for the Synthesis of [2-(Alkylsulfanyl) Imidazo [1, 2-a] Pyridin-3-yl](Aryl) Methanone. J Comb Chem 2009, 12 (1), 41–44.
- Kianmehr, E.; Rajabi, A.; Ghanbari, M. Palladium-Catalyzed Addition of Arylboronic Acids to Isocyanates. Tetrahedron Lett 2009, 50 (15), 1687–1688.
- Kianmehr, E.; Zafarghandi, N.S.; Ghanbari, M. A Simple Route for the Synthesis of Novel N-Alkyl-2-(Alkylthio)-1H-Imidazole Derivatives. Mol Diversity 2013, 17 (2), 383–388.
- Kianmehr, E.; Ghanbari, M. A Direct Palladium-Catalyzed Route for the Synthesis of Benzo [a] Carbazoles Through Sequential C–C Bond Formation and C–H Bond Functionalization. Eur J Org Chem 2012, 2012 (2), 256–259.
- Tayebee, R.; Amini, M.M.; Rostamian, H.; Aliakbari, A. Preparation and Characterization of a Novel Wells–Dawson Heteropolyacid-Based Magnetic Inorganic–Organic Nanohybrid Catalyst H 6 P 2 W 18 O 62/Pyridino-Fe 3 O 4 for the Efficient Synthesis of 1-Amidoalkyl-2-Naphthols Under Solvent-Free Conditions. Dalton Trans 2014, 43 (4), 1550–1563.
- Behzad, S.K.; Balati, A.; Amini, M.M.; Ghanbari, M. Triazine-modified Magnetite Nanoparticles as a Novel Sorbent for Preconcentration of Lead and Cadmium Ions. Microchimica Acta 2014, 181 (15-16), 1781–1788.
- Behzad, S.K.; Amini, M.M.; Balati, A.; Ghanbari, M.; Sadeghi, O. Adsorption and Determination of Cr (VI) in Environmental Samples Using Triazine-Modified Fe3O4 Nanoparticles: Kinetics and Equilibrium Modeling. J Sol-Gel Sci Technol 2016, 78 (2), 446–456.
- Liu, X.l.; Ma, Z.; Xing, J.; Liu, H. Preparation and Characterization of Amino–Silane Modified Superparamagnetic Silica Nanospheres. J Magn Magn Mater 2004, 270 (1), 1–6.
- Safari, J.; Zarnegar, Z. A Magnetic Nanoparticle-Supported Sulfuric Acid as a Highly Efficient and Reusable Catalyst for Rapid Synthesis of Amidoalkyl Naphthols. J Mol Catal A: Chem 2013, 379, 269–276.
- Tayebee, R.; Nehzat, F.; Rezaei-Seresht, E.; Mohammadi, F.Z.; Rafiee, E. An Efficient and Green Synthetic Protocol for the Preparation of bis (Indolyl) Methanes Catalyzed by H 6 P 2 W 18 O 62· 24H 2 O, with Emphasis on the Catalytic Proficiency of Wells-Dawson Versus Keggin Heteropolyacids. J Mol Catal A: Chem 2011, 351, 154–164.
- Gao, Q.; Chen, F.; Zhang, J.; Hong, G.; Ni, J.; Wei, X.; Wang, D. The Study of Novel Fe 3 O 4@ γ-Fe 2 O 3 Core/Shell Nanomaterials with Improved Properties. J Magn Magn Mater 2009, 321 (8), 1052–1057.
- Ren, Y.-M.; Shao, J.-J.; Wu, Z.-C.; Xu, M.-D. PEG1000-based Dicationic Acidic Ionic Liquid Catalyzed one-pot Synthesis of 1, 4-Dihydropyridines via the Hantzsch Reaction. Org Prep Proced Int 2014, 46 (6), 545–550.
- Mirzaei, H.; Davoodnia, A. Microwave Assisted sol-gel Synthesis of MgO Nanoparticles and Their Catalytic Activity in the Synthesis of Hantzsch 1, 4-Dihydropyridines. Chinese J Catal 2012, 33 (9), 1502–1507.
- Murthy, Y.; Rajack, A.; Ramji, M.T.; Praveen, C.; Lakshmi, K.A. Design, Solvent Free Synthesis, and Antimicrobial Evaluation of 1, 4 Dihydropyridines. Bioorgan & Med Chem Lett 2012, 22 (18), 6016–6023.
- Debache, A.; Ghalem, W.; Boulcina, R.; Belfaitah, A.; Rhouati, S.; Carboni, B. An Efficient one-Step Synthesis of 1, 4-Dihydropyridines via a Triphenylphosphine-Catalyzed Three-Component Hantzsch Reaction Under Mild Conditions. Tetrahedron Lett 2009, 50 (37), 5248–5250.
- Antonyraj, C.A.; Kannan, S. Hantzsch Pyridine Synthesis Using Hydrotalcites or Hydrotalcite-Like Materials as Solid Base Catalysts. Appl Catal, A 2008, 338 (1), 121–129.