ABSTRACT
NiO is one of the most important candidates for semiconductors metal oxide nanocrystals by the arrangement of photocatalytic application. However, the photocatalytic performance of biosynthesized nanocrystals using Aspalathus linearis (Burm.f.) R. Dahlgren has not been investigated yet. In this contribution, we synthesize α-Ni(OH)2 using an A. linearis. A heat treatment of the α-Ni(OH)2 is carried out at 300–400°C for 2 h at normal air yields. Furthermore, we have characterized the structural, optical and photocatalytic activity of the samples. The optical results indicate that biosynthesized nanocrystals exhibit UV–visible light absorption and a narrow range distribution of intense green light (518.95 nm) emission, which decreases significantly as annealing temperature increases. The bandgap energies of the sample annealed at 300–400°C shift to lower photon energy, compared to bulk NiO (∼ 4 eV). Moreover, the photocatalytic experimental results reveal that NiO nanocrystals enable color switching of methylene blue.
GRAPHICAL ABSTRACT
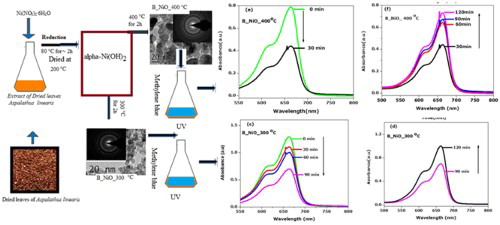
1. Introduction
The semiconductor-metal oxide nanostructures have gained considerable attention from the scientists worldwide due to their promising applications in energy supply and environmental remediation for photoreversible color-switching materials ( Citation1–5). Nickel oxide nanocrystallites has a rock salt structure which exhibits the characteristics of a p-type semiconductor ( Citation6). NiO nanostructures are chemically stable and has good electrical conductivity as well as magnetic and optical properties, which make it a promising material for technological applications such as antiferromagnetic materials ( Citation7), electrode materials for lithium-ion batteries ( Citation8), electrochemical supercapacitors ( Citation9), gas sensors adsorbents ( Citation10), optical amplifier and tunable laser ( Citation11), photovoltaic devices ( Citation12) and electrochromic materials ( Citation13,Citation14). Methods such as sol-gel method ( Citation15), solvothermal route ( Citation16), hydrothermal ( Citation17), thermal decomposition ( Citation18), precipitation method ( Citation19), and plants such as Moringa oleifera ( Citation20), Tamarix serotine ( Citation21), Callistemon viminalis ( Citation22) and Agathosma betulina ( Citation23) allow the synthesis of NiO nanostructures. Among them, TiO2 is one of the excellent metal oxide photocatalysts for water splitting and oxidative degradation of organic pollutants ( Citation24,Citation25). Irradiated with sufficient photon energy, an electron (e−) of TiO2 moves out of its energy level, leaving behind a hole (h+) ( Citation26–28). The hole reacts with adsorbed OH− or H2O on the semiconductor surface to produce (•OH). The electron reduces the molecules present oxygen into (•O2−), •OH and •O2− active species destroy the toxic compounds. Fortunately, electrons and holes recombine mostly, leading a decrease of photocatalytic activity or emigrate into the surface-bound of the semiconductor photocatalyst. Thus, Wang et al. ( Citation29) synthesize TiO2 nanocrystals enabling color switching of methylene blue (MB) in response to light. Their surface-bound molecules scavenge the photogenerated holes of TiO2 nanocrystals upon UV irradiation and the surviving emigrated electrons reduce MB to colorless leuco methylene blue (LMB), which recovers dramatically its blue spectrum upon visible light. Recently, Han et al. ( Citation30) reported the visible light color-switching system of coupling the photoreduction activity of Sn2+-doped SnO2−x nanocrystals with the electrochromic properties of a redox system. The self-doping of Sn2+ in SnO2−x redshifts their absorption to visible region and simultaneously produces oxygen vacancies which effectively scavenge the photogenerated holes and thus enable color switching of redox dye using light irradiation. However, there is no report of NiO nanocrystals enabling color switching of Methylene in response to light source under ambient conditions.
In this work, we have reported NiO nanocrystals through α-Ni(OH)2 by the green novel approach and environmentally friendly pathway using Aspalathus linearis and propose a mechanism of their formation. In our knowledge, we investigated the structural, optical and catalytic performance of NiO nanocrystals in a detailed report.
2. Experimental section
2.1. Materials and methods
In this work, we used A. linearis leaf extract as a reduction/chelating agent. Commonly known as Rooibos, A. linearis is a plant originally found in the Western Cape province of South Africa. It possesses antioxidant, antiaging, anticancer, antidiabetic and anti-inflammatory properties and is used for treating cardiac arrhythmias, colic, diarrhea, asthma and hypertension ( Citation31) as well in nanomaterials production. The most bioactive compounds reducing/ chelating have been reported in our previous works ( Citation28–35). In typical extraction, high-quality dried flowers of A. linearis leaves were weighted and cleaned with cold distilled water. Ten grams were added to 250 ml of deionized water for 48 h; the obtained extraction was filtered using Whatman paper filter paper. The extracted A. linearis leaves dye pH was ∼5 at room temperature (RT). In the typical biosynthesis of NiO nanocrystals, to the 200 ml of the filtered reddish-brown aqueous extract obtained was added 4 g of [Ni(NO3)2·6H2O] at RT. This solution was stirred constantly at a temperature of 80°C for ∼2 h. Respectively, the mixture was collected in Becher and dried at 200°C to obtain light green products [α-Ni(OH)2]. The resulting light green products were thermally treated at 300°C and 400°C for 2 h. The samples will be designed as B_NiO_300°C and 400°C B_NiO_400°C where B means biosynthesized. A schematic diagram of the formation of NiO nanocrystals using A. linearis is shown in .
The major functional groups in products were examined using Fourier transform-infrared absorption spectrometer (Mattson Instruments, Inc., Galaxy 7020A). The crystalline structure of NiO confirmed by powder diffraction, a Bruker D8-Advance diffractometer with Cukα radiation (λ = 0.15406 nm). JEOL JEM 4000EX electron microscopy unit operating at a 400 kV with a point-to-point resolution of 0.17 nm was conducted to investigate the microstructure of the biosynthesized NiO matrix. The UV–VIS absorption spectra of NiO nanocrystals were performed by using an Agilent 8453 spectrophotometer. Photoluminescence (PL) spectra were measured at RT on a Cary Eclipse Fluorescence Spectrophotometer.
2.2. Photocatalytic activity
The photocatalytic activity of biosynthesized NiO nanocrystals system of (B_NiO_W_MB) was tested at RT in a photocatalytic reactor chamber equipped with UV lamps emitting in the range (100–280 nm) located ∼5 cm above the reactor. For the photocatalytic experiment, 5 mg of NiO catalysts was dispersed in 50 ml of 10 mgl−1 MB aqueous solutions containing in 50 ml Becher. The initial pH of the solution was 6.5 for all experiments. Prior to UV illumination, the system was stirred by a magnetic stirrer in dark for 30 min to ensure the adsorption equilibrium of MB. In addition to that, during UV exposure the system is kept homogeneously. At the specific time intervals between 30 min, about 2 ml of the solution was taken from the reaction chamber by a syringe and analyzed with the Agilent 8453 spectrophotometer.
3. Results and discussion
3.1. Structural and morphological analysis
displays FTIR spectra of dried products [spectrum (a)] and the specimen thermally treated at 300°C [spectrum (b)]. The spectra of the sample annealed at 400°C were similar to the specimen thermally treated at 300°C within the considered spectral region. The spectrum (a) shows the typical features α-Ni(OH)2, which are described in detail elsewhere (
Citation36). The broad absorption band spectrum centered at 3405 cm−1 corresponds to the O–H of hydrogen-bonded water molecules which exist the interlamellar space of α-Ni(OH)2 (
Citation37). The band at 1643 cm−1 is assigned to bending vibrations of the interlayer water molecules (
Citation38,Citation39). The weak band at 2380 and 994 cm−1 are attributed to intercalated and
(
Citation40,Citation41). The presence of 1390 cm−1 arises from the interaction of the samples with the KBr in the pellet (
Citation42). In the lower wavenumber, the two bands appearing at 715 and 495 cm−1 are attributed to δOH and ν(Ni–O) vibrations, respectively (
Citation43–45). After annealing at 300°C for 2 h, the δOH disappeared and a strong band at 449 cm−1 corresponding to the stretching vibration of Ni–O (
Citation46) was observed, which clearly reveal the complete dehydration α type of hydroxides, leading to the formation of NiO nanostructures.
Figure 2. FTIR spectra of dried products (spectrum (a)) and the sample thermally treated at 300°C (spectrum (b)).
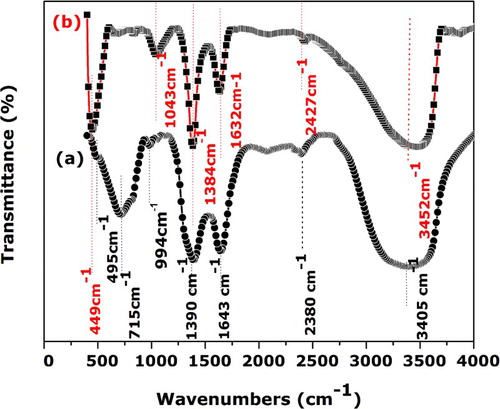
(a) shows the XRD spectrum of the synthesized nanosamples dried at 200°C, which clearly exhibits the characteristic diffraction of α-Ni(OH)2 (JCPDS card no. 38–0715) ( Citation47,Citation48). While annealed temperature is raised up to 300°C, the specimens manifest a uniform crystal structure with five diffraction peaks centered 37.24°, 43.27°, 62.87°, 75.41° and 79.40°, as shown in (b). These diffraction peaks are ascribed respectively to crystallographic reflections from (111), (200), (220), (311) and (222) planes of NiO (space group Fm3m, JCPDS card no 00-047-1049) and are in agreement with several reported results ( Citation49–51). The broad peaks indicate the nanoscaled nature of the synthesized NiO nanostructure. The deduced average crystallite size of the NiO particles annealed at 300°C and 400°C, calculated by the Scherer equation ( Citation52) was about 6 and 10 nm, respectively.
Figure 3. RT X-ray diffraction of the samples: (a) sample annealed at 200°C, and (b) samples annealed at 300°C and 400°C.
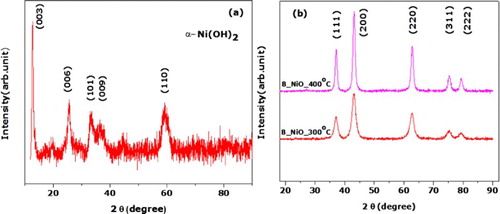
(a) shows the TEM image of B_NiO_300°C. It is observed that B_NiO_300°C consists of quasi-spherical shape nanoscaled particles with size around 7 nm, as shown in (e). Once rising the annealed temperature up to 400°C, the growth direction of the nanocrystals occurs ((c)). The size of B_NiO_400°C is around 14 nm, as shown in (f). One was to note for B_NiO-300°C, most of the lattice fringes were not discernable, which indicate the presence of defects in some zone of B_NiO_300°C. However, the lattice is clearly seen from the HRTEM image as shown in (d), in which the interplanar distance is about 0.24 nm and consistent with the d spacing of (111) of fcc NiO. The reason for that part of the defect has been altered by the annealing.
3.2. Optical studies
(a) displays the UV–visible absorption spectrum of biosynthesized NiO nanocrystals. B_NiO_300°C exhibits the absorption maximum ranges from 190 to 237 nm with the long shoulder that extends to visible region when compared to B_NiO_400°C, which showed a noticeable shift toward a shorter wavelength. It is observed that absorption intensity of B_NiO decreases with an increase in annealing temperature. Furthermore, the extension spectrum of visible region is related to the presence of oxygen vacancies in B_NiO nanocrystals. Wang et al. (
Citation53) reported similar findings regarding the ZnO nanoparticles, where oxygen species extend to visible region absorption. However, to decrease the absorption peak with an increase in annealing temperature is due to increasing the crystalline size and the reduction effect of crystal defects. The B_NiO_300°C sample captures the photons energy efficiently and exhibits a strong absorption in UV region. The B_NiO_400°C sample possesses a larger particle size to exhibit the weak UV absorption since higher annealing temperature allows improving their structure crystallinity, which may lead to decreasing the bandgap energy (
Citation9). This is in accord with XRD and HRTEM analysis, the annealing effect which crystalline has increased, the defects and the grain boundary density of nanograins are decreased, subsequently, the scattering of the carrier effect at grain voids has decreased (
Citation54). The absorption bandgap Eg is determined by the following equation (
Citation26):(1) where α is the absorption coefficient, hυ is the photon energy, B is a constant related to the material expansion and n (which is equal to 2 for a direct transition or 1/2 for an indirect transition) is determined by quantum selection rules. In this work, we chose n = 2 for the reason that it gives a good fit on the photon energy axis. (b) shows the plot (α hυ)2 vs. hυ for the NiO nano samples annealed at 300°C and 400°C. According to Equation (1), the optical bandgap the NiO nanosamples were estimated through the extrapolation of the linear region on the energy axis as shown in (b). The NiO nanocrystals annealed at 300°C and 400°C possess a bandgap value of 3.4 and 3.5 eV, respectively. Thus, the biosynthesized NiO nanocrystals are the potential candidate for optoelectric applications. It is established that the nanoscaled semiconductor system shows a blue shift in UV–visible studies by the cause of quantum confinement effects. However, one of the energies gaps of B_NiO nanosamples are smaller than the bulk product (4 eV) (
Citation55), which is noted possibly as a result of news energy levels in the bandgap region due to the presence of vacancies or chemical defects (
Citation56).
Figure 5. (a) UV–vis absorption spectrum of biosynthesized NiO nanocrystals, and (b) plot (α hυ)2 vs. hυ for the biosynthesized NiO nanocrystals.
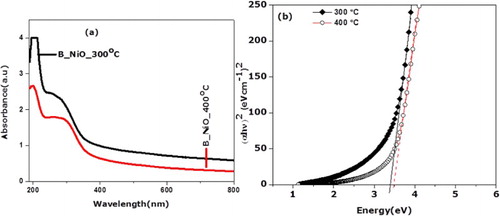
To examine the nature of the defect and ion deficiencies, RT Photoluminescence measurements of B_NiO nanosamples under 257 nm excitation wavelength were conducted. (a) showed the PL spectra of B_NiO nanosamples in the wavelength range of ∼258–800 nm. Let us note that the relative intensity of narrow edge distribution of green emission of B_NiO_300°C sample at 518.95 nm (∼2.4 eV), which decreases about 64% for the sample B_NiO_400°C. The diminution in PL intensity of B_NiO_400°C at 518.95 nm compared to B_NiO_300°C is attributed to the filling of oxygen voids during heating treatment. Kim et al. ( Citation57) reported similar findings in ZnO nanostructures where green emission about 518 nm is attributed to the oxygen vacancies. Second, the effect of Ni2+ vacancies is not evident, as shown in (a). In fact, one O2− vacancy in NiO annihilates the effect of Ni2+ vacancy. Therefore, if in sample of O2− and Ni2+ vacancies, Ni2+ effect will be manifest only if Ni2+ vacancies is more than the number of O2− vacancies ( Citation58). (b) displays the inset of PL spectrum of B_NiO samples in the range of ∼258–600 nm (I < 7 cps). B_NiO_300°C nanosample shows UV emission band at 283 nm (4.38 eV) and 353 nm (3.51 eV) and visible emission bands at 431 nm (2.87 eV), 460 nm (3.08 eV) and 586 nm (2.11 eV). PL spectrum of B_NiO_400°C represents relatively the UV emission bands at 356 nm (3.48 eV) and 390 nm (3.17 eV) and visible emission bands at 431 nm (2.87 eV), 460 nm and 586 nm. The PL UV emissions at 283 and 356 nm corresponding respectively to B_NiO_300°C and B_NiO_400°C nanosamples are ascribed to the functional optical bandgap of NiO nanosamples. It is well known that the heat treatment results are relative deviation of NiO stoichiometry, in nickel vacancy and/or oxygen trapping in the NiO lattice as well as an enhancement of optical transition in the range 2–4 eV due to the intraionic transition 3d8–3d8* of Ni2+ ( Citation58–61). In this report, the biosynthesized NiO nanoparticles were treated of visible emission bands of B_NiO_400°C nanosample at 431 nm (2.87 eV), 460 nm (3.08 eV) and 586 nm (2.11 eV) were enhanced compared to B_NiO_300°C sample. Hence, these later visible emission peaks are attributed to the electronic transition of Ni2+. The color change of the biosynthesized NiO (greenish to black) can also be justified by the existence of nickel vacancy and or/interstitial oxygen defects. However, in the presence form of O2− vacancies, the intensities of the visible emissions due to the intraionic transition are negligible compared to the green luminescence originated to the oxygnen vacancies as shown in (a).
3.3. Photocatalytic application
The light responses of biosynthesized NiO nanocrystals were recorded at functional irradiation time. As shown in (a), upon the UV exposure of methylene without B_NiO, slight decreases of absorption peak are recorded after 120 min. It is worth noting that 3% of MB is bleached and recolouration process of MB is not observed. The MB bleached detection is negligible
Figure 7. Light responses of biosynthesized NiO nanocrystals/water/MB system. (a) UV–visible spectra displaying the bleaching process of MB under UV radiation without biosynthesized NiO nanocrystals. (b) The plots of the ratio of MB in biosynthesized NiO nanocrystals/water/MB system vs. irradiation time. (c–f) UV–visible spectra displaying the bleaching/ recoloration process of biosynthesized NiO nanocrystals/water/MB systems.
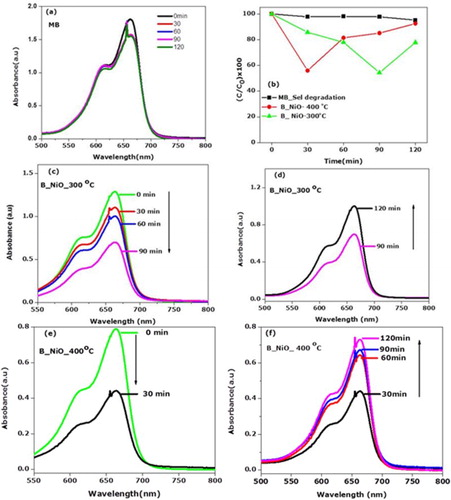
by MB degradation dye, it is seen that the B_NiO_300°C and B_NiO_400°C showed obvious photo-bleaching following the recombination effect of MB, as shown in (b–f). We required 90 min of UV irradiation so as to bleach about 46% with B_NiO_300°C, while it took only 30 min with B_NiO_400°C. Thus, the photo-bleaching ratio of B_NiO_400°C is about three times as that of B_NiO_300°C, which indicated that the heating can improve the bleaching effect of the biosynthesized NiO nanoparticles because photogenerated electron–hole pairs are generated less on the surface morphology of photocatalytic, act as a poor crystallinity and the crystal orientation growth would be imperfect ( Citation26). The heating effect which leads to a perfect crystal pattern of B_NiO_400°C has a good crystallinity (narrow XRD peaks in (b)). As we have highlighted in Section 1, upon UV light irradiation with sufficient equal energy, a mass of electrons and holes are generated in the matrix. The huge amount of electrons and holes would recombine at recombination centers immediately once the irradiation stopped working and a little amount would diffuse to the surface of OH radical ( Citation62). Therefore, the recombination of the photogenerated electrons and holes has been prevented as much as bleaching reaction be favored. Upon UV, B_NiO_400°C reproduced huge electron–hole recombination of charge photocatalyst compound B_NiO_400°C, leading to the right reduction of MB to its colorless LMB. However, to compare the B_NiO_300°C that the fast reduction could not happen for a long duration due to lower content of oxygen vacancy to scavenge the photogenerated hole, which leads to recombination of electrons and holes. In fact, with the oxygen-deficient photocatalytic semiconductor, the oxygen vacancies scavenge the photogenerated holes and subsequently promote the reduction of MB to its colorless leuco ( Citation5). On the other hand, the higher photogenerated electron–hole ratio of surface morphology and lower oxygen vacancies of B_NiO_400°C nanosample compared to B_NiO_300°C are the reason that the bleaching ratio of B_NiO_400°C is about three times as that of B_NiO_300°C. In this case, it is observed from the (a,b) that UV irradiation of MB without NiO photocatalysts cannot reverse the decoloration process. Furthermore, the photo-oxidation quenching process ( Citation63) is also negligible at the limitation of experimental condition. To our knowledge, with the presence of NiO photocatalysts, while increasing the UV exposure irradiation time, the reactions were found to occur in the reverse direction of recoloration effect. Thus the recoloration process act by the self-catalyzed oxidation of LMB. Similarly to our findings, the recoloration effect of self-oriented oxidation of leuco methylene blue was reported elsewhere ( Citation29). To our understanding, NiO nanocrystals absorb the visible light irradiation and excite LMB to LMB* which reacts the dissolved oxygen species of MB to enhance the recoloration ratio of B_NiO_300°C due to its larger oxygen vacancy content which leads to increase the absorption of visible range spectrum as shown in (a).
4. Conclusion
In summary, we have successfully prepared α-Ni(OH)2 via A. linearis leaf extract by the heating treatment of nickel hydroxide in normal air atmosphere at 300°C to allow acquiring single phase NiO nanocrystalline matrix with average particles size of 14 nm as supportable of FTIR, XRD and HRTEM investigation. The biosynthesized NiO nanocrystals exhibited UV–visible light absorption and a narrow range distribution of intense green light (518.95 nm) emission. We reported the photocatalytic performance of MB, which shows that the biosynthesized NiO nanocrystals enable color switching of Methylene in response to a light source under ambient conditions. Furthermore, in this work, based on the device orientation of photo-rewritable printers, gas sensing and optoelectronics requires to be carried out and the results obtained compared with existing literatures.
Disclosure statement
No potential conflict of interest was reported by the authors.
Notes on contributors
A. Diallo completed his B.Sc in 2005 at Cheikh Anta Diop University, Senegal, followed by his M.Sc. in Physics and Chemistry at the same University in 2006. In 2016, he completed his Ph.D. in Condensed Mater Physics, also at Cheikh Anta Diop University. In 2017, he completed his PhD in Physics at the University of South Africa. He is currently a Lecturer at the Department of Physics and Chemistry, Faculty of Sciences and Technologies of Formation and Education, University Cheikh Anta Diop, Senegal.
K. Kaviyarasu is an expert in materials sciences and engineering from iThemba Labs.
S. Ndiaye is an expert in X-rays diffraction & Raman spectroscopy from Cheikh Anta Diop.
B. M. Mothudi is an expert in nanophosphors and photoluminescence from the University of South Africa.
A. Ishaq is an expert in ion beam modification & analysis of nanomaterials from the National Center for Physics, Quaid-i-Azam University, Islamabad-44000, Pakistan.
V. Rajendran is an expert in materials sciences and director of the centre for Nano Science & Technology, K.S. Rangasamy College of Technology, Tiruchengode, Tamil Nadu, India.
M. Maaza holds the UNESCO-UNISA Africa Chair in nanosciences and nanotechnology. He is an expert in materials at the nanoscale. He is a fellow of the Royal Society of Chemistry, London, African Academy of Sciences, Islamic Academy of Sciences, and the New York Academy of Sciences.
ORCID
A. Diallo http://orcid.org/0000-0002-4032-0477
V. Rajendran http://orcid.org/0000-0001-9471-5311
Additional information
Funding
References
- Gharagozlou, M.; Bayati, R. Photocatalytic Characteristics of Single Phase Fe-Doped Anatase TiO2 Nanoparticles Sensitized with Vitamin B12. Mater. Res. Bull. 2015, 61, 340–347. doi: 10.1016/j.materresbull.2014.10.043
- Wang, H.; Zhang, L.; Chen, Z.; Hu, J.; Li, S.; Wang, Z.; Liu, J.; Wang, X. Semiconductor Heterojunction Photocatalysts: Design, Construction, and Photocatalytic Performances. Chem. Soc. Rev. 2014, 43, 5234–5244. doi: 10.1039/C4CS00126E
- Krýsa, J.; Keppert, M.; Jirkovský, J.; Štengl, V.; Šubrt, J. The Effect of Thermal Treatment on the Properties of TiO2 Photocatalyst. Mater. Chem. Phys. 2004, 86, 333–339. doi: 10.1016/j.matchemphys.2004.03.021
- Kaviyarasu, K.; Kotsedi, L.; Simo, A.; Fuku, X.; Mola, G.T.; Kennedy, J.; Maaza, M. Photocatalytic Activity of ZrO2 Doped Lead Dioxide Nanocomposites: Investigation of Structural and Optical Microscopy of RhB Organic Dye. Appl. Surf. Sci. 2017, 421, 234–239. doi: 10.1016/j.apsusc.2016.11.149
- Wang, W.; Ye, Y.; Feng, J.; Chi, M.; Guo, J.; Yin, Y. Enhanced Photoreversible Color Switching of Redox Dyes Catalyzed by Barium-Doped TiO2 Nanocrystals. Angew. Chem. Int. Ed. 2015, 127, 1337–1342. doi: 10.1002/ange.201410408
- Molaei, R.; Bayati, M.R.; Alipour, H.M.; Nori, S.; Narayan, J. Enhanced Photocatalytic Efficiency in Zirconia Buffered n-NiO/p-NiO Single Crystalline Heterostructures by Nanosecond Laser Treatment. J. Appl. Phys. 2013, 113, 233708. doi: 10.1063/1.4811540
- Krishnakumar, S.; Liberati, M.; Grazioli, C.; Veronese, M.; Turchini, S.; Luches, P.; Valeri, S.; Carbone, C. Magnetic Linear Dichroism Studies of In Situ Grown NiO Thin Films. J. Magn. Magn. Mater. 2007, 310, 8–12. doi: 10.1016/j.jmmm.2006.07.020
- Ni, S.; Li, T.; Yang, X. Fabrication of NiO Nanoflakes and Its Application in Lithium Ion Battery. Mater. Chem. Phys. 2012, 132, 1108–1111. doi: 10.1016/j.matchemphys.2011.12.082
- Zhao, J.; Liu, H.; Zhang, Q. Preparation of NiO Nanoflakes Under Different Calcination Temperatures and their Supercapacitive and Optical Properties. Appl. Surf. Sci. 2017, 392, 1097–1106. doi: 10.1016/j.apsusc.2016.09.128
- Zhang, Y.; Zeng, W. New Insight into Gas Sensing Performance of Nanoneedle-Assembled and Nanosheet-Assembled Hierarchical NiO Nanoflowers. Mater. Lett. 2017, 195, 217–219. doi: 10.1016/j.matlet.2017.02.124
- Wu, B.T.; Qiu, J.R.; Wu, E.; Zeng, H.P. Broadband Near-Infrared Luminescence from Transparent Glass–Ceramics Containing Ni2+-Doped SrTiO3 Nanocrystals. Opt. Mater. 2013, 35, 983–987. doi: 10.1016/j.optmat.2012.12.004
- Borgström, M.; Blart, E.; Boschloo, G.; Mukhtar, E.; Hagfeldt, A.; Hammarström, L.; Odobel, F. Sensitized Hole Injection of Phosphorus Porphyrin into NiO: Toward New Photovoltaic Devices. J. Phys. Chem. B. 2005, 109, 22928–22934. doi: 10.1021/jp054034a
- Wang, X.; Wan, L.; Yu, T.; Zhou, J.; Guana, J.; Yu, Z.; Li, Z.; Zou, Z. Non-Basic Solution Eco-Routes to Nano-Scale NiO with Different Shapes: Synthesis and Application. Mater. Chem. Phys. 2011, 126, 494–499. doi: 10.1016/j.matchemphys.2011.01.040
- Dorneanu, P.P.; Airinei, A.; Olaru, N.; Homocianu, M.; Nica, V.; Doroftei, F. Preparation and Characterization of NiO, ZnO and NiO–ZnO Composite Nanofibers by Electrospinning Method. Mater. Chem. Phys. 2014, 148, 1029–1035. doi: 10.1016/j.matchemphys.2014.09.014
- Sankar, S.; Sharma, S.K.; An, N.; Lee, H.; Kima, D.Y.; Im, Y.B.; Cho, Y.D.; Ganesh, R. S.; Ponnusamy, S.; Raji, P.; Purohit, L.P. Photocatalytic Properties of Mn-Doped NiO Spherical Nanoparticles Synthesized from Sol-Gel Method. Optik – Int. J. Light Electron. Opt. 2016, 127, 10727–10734. doi: 10.1016/j.ijleo.2016.08.126
- Qing, Z.; Haixia, L.; Huali, L.; Yu, L.; Huayong, Z.; Tianduo, L. Solvothermal Synthesis and Photocatalytic Properties of NiO Ultrathin Nanosheets with Porous Structure. Appl. Surf. Sci. 2015, 328, 525–530. doi: 10.1016/j.apsusc.2014.12.077
- Motevalli, K.; Zarghami, Z.; Panahi-Kalamuei, M. Simple, Novel and Low-Temperature Synthesis of rod-Like NiO Nanostructure via Thermal Decomposition Route Using a New Starting Reagent and Its Photocatalytic Activity Assessment. J. Mater. Sci.: Mater. Electron. 2016, 27, 4794–4799.
- Nassar, M.Y.; Aly, H.M.; Abdelrahman, E.A.; Moustafa, M.E. Synthesis, Characterization, and Biological Activity of Some Novel Schiff Bases and Their Co(II) and Ni(II) Complexes: A New Route for Co3O4 and NiO Nanoparticles for Photocatalytic Degradation of Methylene Blue Dye. J. Mol. Struct. 2017, 1143, 462–471. doi: 10.1016/j.molstruc.2017.04.118
- Gnanasekaran, L.; Hemamalini, R.; Saravanan, R.; Ravichandran, K.; Gracia, F.; Agarwal, S.; Gupta, V.K. Synthesis and Characterization of Metal Oxides (CeO2, CuO, NiO, Mn3O4, SnO2 and ZnO) Nanoparticles as Photo Catalysts for Degradation of Textile Dyes. J. Photochem. Photobiol., B. 2017, 173, 43–49. doi: 10.1016/j.jphotobiol.2017.05.027
- Ezhilarasi, A.A.; Vijaya, J.J.; Kaviyarasu, K.; Maaza, M.; Ayeshamariam, A.; John Kennedy, L. Green Synthesis of NiO Nanoparticles Using Moringa Oleifera Extract and Their Biomedical Applications: Cytotoxicity Effect of Nanoparticles Against HT-29 Cancer Cells. J. Photochem. Photobiol., B. 2016, 164, 352–360. doi: 10.1016/j.jphotobiol.2016.10.003
- Nasseri, M.A.; Ahrari, F.; Zakerinasab, B. A Green Biosynthesis of NiO Nanoparticles Using Aqueous Extract of Tamarix Serotina and Their Characterization and Application. Appl. Organomet. Chem. 2016, 30, 978–984. doi: 10.1002/aoc.3530
- Sone, B.T.; Fuku, X.G.; Maaza, M. Physical & Electrochemical Properties of Green Synthesized Bunsenite NiO Nanoparticles via Callistemon Viminalis’ Extracts. Int. J. Electrochem. Sci. 2016, 11, 8204–8220. doi: 10.20964/2016.10.17
- Thema, F.T.; Manikandan, E.; Gurib-Fakim, A.; Maaza, M. Single Phase Bunsenite NiO Nanoparticles Green Synthesis by Agathosma Betulina Natural Extract. J. Alloys. Compd. 2016, 657, 655–661. doi: 10.1016/j.jallcom.2015.09.227
- Fujihira, M.; Satoh, Y.; Osa, T. Heterogeneous Photocatalytic Oxidation of Aromatic Compounds on TiO2. Nature 1981, 293, 206–208. doi: 10.1038/293206a0
- Roy, P.; Berger, S.; Schmuki, P. TiO2 Nanotubes: Synthesis and Applications. Angew. Chem., Int. Ed. 2011, 50, 2904–2939. doi: 10.1002/anie.201001374
- Wan, X.; Yuan, M.; Tie, S.-L.; Lan, S. Effects of Catalyst Characters on the Photocatalytic Activity and Process of NiO Nanoparticles in the Degradation of Methylene Blue. Appl. Surf. Sci. 2013, 277, 40–46. doi: 10.1016/j.apsusc.2013.03.126
- Marien, C.B.D.; Cottineau, T.; Robert, D.; Drogui, P. TiO2 Nanotube Arrays: Influence of Tube Length on the Photocatalytic Degradation of Paraquat. Appl. Catal., B. 2016, 194, 1–6. doi: 10.1016/j.apcatb.2016.04.040
- Abou-Gamra, Z.M.; Ahmed, M.A. Synthesis of Mesoporous TiO2–Curcumin Nanoparticles for Photocatalytic Degradation of Methylene Blue dye. J. Photochem. Photobiol., B. 2016, 160, 134–141. doi: 10.1016/j.jphotobiol.2016.03.054
- Wang, W.; Ye, M.; He, L.; Yin, Y. Nanocrystalline TiO2-Catalyzed Photoreversible Color Switching. Nano. Lett. 2014, 14, 1681–1686. doi: 10.1021/nl500378k
- Han, D.; Jiang, B.; Feng, J.; Yin, Y.; Wang, W. Photocatalytic Self-Doped SnO2−x Nanocrystals Drive Visible-Light-Responsive Color Switching. Angew. Chem. Int. Ed. 2017, 56, 7792–7796. doi: 10.1002/anie.201702563
- Khan, A.-u.; Gilani, A.H. Selective Bronchodilatory Effect of Rooibos Tea (Aspalathus linearis) and Its Flavonoid, Chrysoeriol. Eur. J. Nutr. 2006, 45, 463–469. doi: 10.1007/s00394-006-0620-0
- Diallo, A.; Mothudi, B.M.; Manikandan, E.; Maaza, M. Luminescent Eu2O3 Nanocrystals by Aspalathus linearis’ Extract: Structural and Optical Properties. J. Nanophotonics 2016, 10, 026010. doi: 10.1117/1.JNP.10.026010
- Diallo, A.; Ngom, B.D.; Park, E.; Maaza, M. Green Synthesis of ZnO Nanoparticles by Aspalathus linearis: Structural & Optical Properties. J. Alloys. Compd. 2015, 646, 425–430. doi: 10.1016/j.jallcom.2015.05.242
- Diallo, A.; Beye, A.C.; Doyle, T.B.; Park, E.; Maaza, M. Green Synthesis of Co3O4 Nanoparticles via Aspalathus linearis: Physical Properties. Green Chem. Lett. Rev. 2015, 8, 30–36. doi: 10.1080/17518253.2015.1082646
- Diallo, A.; Doyle, T.B.; Mothudi, B.M.; Manikandan, E.; Rajendran, V.; Maaza, M. Magnetic Behavior of Biosynthesized Co3O4 Nanoparticles. J. Magn. Magn. Mater. 2017, 424, 251–255. doi: 10.1016/j.jmmm.2016.10.063
- Portemer, F.; Delahaye-Vidal, A.; Figlarz, M. Characterization of Active Material Deposited at the Nickel Hydroxide Electrode by Electrochemical Impregnation. J. Electrochem. Soc. 1992, 139, 671. doi: 10.1149/1.2069283
- de Soler-Illia, G.J.A.A.; Jobbágy, M.; Regazzoni, A.E.; Blesa, M.A. Synthesis of Nickel Hydroxide by Homogeneous Alkalinization. Precipitation Mechanism. Chem. Mater. 1999, 11, 3140–3146. doi: 10.1021/cm9902220
- Li, Y.; Xie, X.; Liu, J.; Cai, M.; Rogers, J.; Shen, W. Synthesis of α-Ni(OH)2 with Hydrotalcite-Like Structure: Precursor for the Formation of NiO and Ni Nanomaterials with Fibrous Shapes. Chem. Eng. J. 2008, 136, 398–408. doi: 10.1016/j.cej.2007.06.001
- Xu, L.P.; Ding, Y.S.; Chen, C.H.; Zhao, L.L.; Rimkus, C.; Joesten, R.; Suib, S.L. 3D Flowerlike α-nickel Hydroxide with Enhanced Electrochemical Activity Synthesized by Microwave-assisted Hydrothermal Method. Chem. Mater. 2007, 20, 308–316. doi: 10.1021/cm702207w
- Tong, G.-X.; Liu, F.-T.; Wu, W.-H.; Shen, J.-P.; Hu, X.; Liang, Y. Polymorphous α- and β-Ni(OH)2 Complex Architectures: Morphological and Phasal Evolution Mechanisms and Enhanced Catalytic Activity as non-Enzymatic Glucose Sensors. Cryst. Eng. Comm. 2012, 14, 5963–5973. doi: 10.1039/c2ce25622c
- Rajamathi, M.; Vishnu Kamath, P. On the Relationship Between α-Nickel Hydroxide and the Basic Salts of Nickel. J. Power Sources 1998, 70, 118–121. doi: 10.1016/S0378-7753(97)02656-6
- Genin, P.; Delahyde-Vidal, A.; Portemer, F.; Tekaia-Ehlsissen, K.; Figlarz, M. Preparation and Characterization of Alpha-type Nickel Hydroxides Obtained by Chemical Precipitation: Study of the Anionic Species. Eur. J. Solid State Inorg. Chem. 1991, 28, 505.
- Le Bihan, S.; Figlarz, M. Croissance de L'hydroxyde de Nickel Ni(OH)2 à Partir D'un Hydroxyde de Nickel Turbostratique. J. Cryst. Growth. 1972, 13–14, 458–461. doi: 10.1016/0022-0248(72)90280-1
- Coudun, C.; Grillon, F.; Hochepied, J.-F. Surfactant Effects on pH-Controlled Synthesis of Nickel Hydroxides. Colloids Surf., A. 2006, 280, 23–31. doi: 10.1016/j.colsurfa.2006.01.018
- Oliva, P.; leonardi, J.; Laurent, J.F.; Delmas, C.; Braconnier, J.J.; Figlarz, M.; Fievet, F.; de Guibert, A. Review of the Structure and the Electrochemistry of Nickel Hydroxides and Oxy-Hydroxides. J. Power Sources 1982, 8, 229–255. doi: 10.1016/0378-7753(82)80057-8
- Sheena, P.A.; Priyanka, K.P.; Sabu, N.A.; Sabu, B.; Varghese, T. Effect of Calcination Temperature on the Structural and Optical Properties of Nickel Oxide Nanoparticles, Nanosystems. Phys. Chem. Math. 2014, 5, 441–449.
- Luo, Y.; Li, G.; Duan, G.; Zhang, L. One-step Synthesis of Spherical α-Ni(OH) 2 Nanoarchitectures. Nanotechnology 2006, 17, 4278–4283. doi: 10.1088/0957-4484/17/16/046
- Han, D.; Xu, P.; Jing, X.; Wang, J.; Yang, P.; Shen, Q.; Liu, J.; Song, D.; Gao, Z.; Zhang, M. Trisodium Citrate Assisted Synthesis of Hierarchical NiO Nanospheres with Improved Supercapacitor Performance. J. Power Sources 2013, 235, 45–53. doi: 10.1016/j.jpowsour.2013.01.180
- Lang, F.; Sun, D.; Liu, J.; Wang, H.; Yan, H. Improved Size-Tunable Synthesis of Monodisperse NiO Nanoparticles. Mater. Lett. 2016, 181, 328–330. doi: 10.1016/j.matlet.2016.06.056
- Kaviyarasu, K.; Manikandan, E.; Kennedy, J.; Jayachandran, M.; Ladchumananandasiivam, R.; Umbelino De Gomes, U.; Maaza, M. Synthesis and Characterization Studies of NiO Nanorods for Enhancing Solar Cell Efficiency Using Photon Upconversion Materials. Ceram. Int. 2016, 42, 8385–8394. doi: 10.1016/j.ceramint.2016.02.054
- Liu, S.; Zeng, W.; Chen, T. Synthesis of Hierarchical Flower-Like NiO and the Influence of Surfactant. Physica E. 2017, 85, 13–18. doi: 10.1016/j.physe.2016.08.016
- Cullity, B.D.; Stock, S.R. Elements of X-ray Diffraction, 3rd ed.; Prentice-Hall: Upper Saddle River, NJ, 2001.
- Wang, J.; Wang, Z.; Huang, B.; Ma, Y.; Liu, Y.; Qin, X.; Zhang, X.; Dai, Y. Oxygen Vacancy Induced Band-Gap Narrowing and Enhanced Visible Light Photocatalytic Activity of ZnO. ACS Appl. Mater. Interfaces 2012, 4, 4024–4030. doi: 10.1021/am300835p
- Lee, J.H.; Ko, K.H.; Park, B.O. Electrical and Optical Properties of ZnO Transparent Conducting Films by the sol–gel Method. J. Cryst. Growth 2003, 247, 119–125. doi: 10.1016/S0022-0248(02)01907-3
- Varkey, A.J.; Fort, A.F. Solution Growth Technique for Deposition of Nickel Oxide Thin Films. Thin Solid Films. 1993, 235, 47–50. doi: 10.1016/0040-6090(93)90241-G
- Song, X.; Gao, L. Facile Synthesis of Polycrystalline NiO Nanorods Assisted by Microwave Heating. J. Am. Ceram. Soc. 2008, 91, 3465–3468. doi: 10.1111/j.1551-2916.2008.02667.x
- Kim, T.Y.; Kim, J.Y.; Senthil Kumar, M.; Suh, E.K.; Nahm, K.S. Influence of Ambient Gases on the Morphology and Photoluminescence of ZnO Nanostructures Synthesized with Nickel Oxide Catalyst. J. Cryst. Growth 2004, 270, 491–497. doi: 10.1016/j.jcrysgro.2004.07.009
- Madhu, G.; Biju, V. Effect of Ni2+ and O2− Vacancies on the Electrical and Optical Properties of Nanostructured Nickel Oxide Synthesized Through a Facile Chemical Route. Physica E. 2014, 60, 200–205. doi: 10.1016/j.physe.2014.02.011
- Cao, H.; Qiu, X.; Liang, Y.; Zhang, L.; Zhao, M.; Zhu, Q. Sol-Gel Template Synthesis and Photoluminescence of n- and p-Type Semiconductor Oxide Nanowires. Chem. Phys. Chem. 2006, 7, 497–501. doi: 10.1002/cphc.200500452
- Subramanian, B.; Mohamed Ibrahim, M.; Senthilkumar, V.; Murali, K.R.; Vidhya, V.S.; Sanjeeviraja, C.; Jayachandran, M. Optoelectronic and Electrochemical Properties of Nickel Oxide (NiO) Films Deposited by DC Reactive Magnetron Sputtering. Physica B. 2008, 403, 4104–4110. doi: 10.1016/j.physb.2008.08.014
- Chakrabarty, S.; Chatterjee, K. . J. Phys. Sci. 2009, 13, 245–250.
- Li, L.; Xu, K.; Wang, Y.; Hu, Z.; Zhao, H. Enhanced Persistent Luminescence and Photocatalytic Properties of Ga2O3:Cr3+ by In3+ Doping. Opt. Mater. Express. 2016, 6, 1022–1030.
- Lee, S.-K.; Mills, A. Novel Photochemistry of Leuco-Methylene Blue. Chem. Commun. 2003, 2366–2367. doi: 10.1039/b307228b