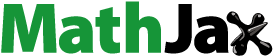
ABSTRACT
Herein, we describe an environmentally friendly strategy to synthesize Ag nanoparticles on Nanodiamond (ND) by utilizing natural adhering and reduction properties of tea polyphenols (TPs). Firstly, the surface of ND was modified by TPs through their natural adhering ability. Then, the modified TPs functioned as reducing agents for reducing Ag precursor in situ. The synthesis process, structure and its physicochemical properties of the nanohybrid were tracked and characterized by UV-Vis, FTIR, TGA, HRTEM, XRD and XPS. It has been shown that Ag nanoparticles with uniform size and distribution were anchored successfully on the surface of ND. The ND supported Ag nanoparticles displayed highly water dispersibility due to the hydrophilicity of TPs, as is very important in heterogeneous catalysis. The catalytic performance of this nanohybrid was evaluated comprehensively by catalyzing 4-nitrophenol reduction by sodium borohydride, which is often used as a model reaction, showing excellent catalytic activity and recycling stability.
GRAPHICAL ABSTRACT
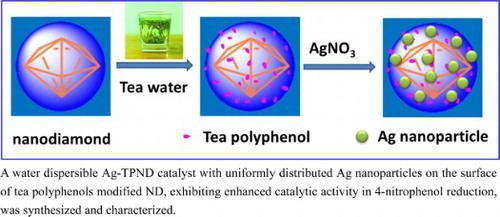
1. Introduction
Over the past few years, the use of supported metal nanoparticles (MNPs) for heterogeneous catalysis in various of chemical transformations has always been a research hotspot in the field of chemical catalysis (Citation1–7). The selective of appropriate carrier materials is the key to obtain highly efficient heterogeneous catalysts (Citation8–14). In the many types of carrier materials appeared in catalytic process, carbon-based structures are undoubtedly the most popular ones due to their specific characteristics such as excellent chemical stability in acid or base media, high surface area, possibility to control of the porosity and surface chemistry, and so on (Citation15–19). However, the MNPs catalysts supported by traditional carbon materials, including activated carbon, graphite nanofiber or nanofilaments, often appear aggregation or leaching of MNPs during the catalytic process due to the lack of functional groups on the surface of supports to stabilize the MNPs (Citation20). New types of carbon nanostructures, such as 1D nanotubes, 2D graphene sheet or its derivatives, and diamond nanoparticles as well as cellulose nanofiber or nanocrystals have recently received tremendous attention as alternative carbon supports due to their distinctive structural and physico-chemical properties as well as their synergistic catalytic effect with MNPs (Citation21–23). Nanodiamond (ND), a new developed carbon nanomaterial, can be prepared on a large scale with low cost using detonation technique. Detonation ND is rich in a variety of oxygen-containing groups such as hydroxyl and carboxylic groups on its surface which help to stabilize the MNPs to prepare the ND supported MNPs catalysts (Citation24–26). Nevertheless, conventional approaches, i.e. impregnation followed by chemical reduction, always resulted in the reduction of oxygen-containing groups simultaneously which will reduce its hydrophilicity and re-dispersibility (Citation27). In order to increase the interaction between the MNPs and the supports, it has been proved that introducing some functional groups with metal complexing ability on the supports surface is an effective method (Citation28, Citation29). However, how to efficiently modify this functional functional function is challenging because it often involves complex preparation procedures, long reaction times, and the application of expensive organic reagents (Citation30). In addition, traditional chemical reduction of metal precursors to preparing MNPs often involves the use of toxic reducing agents. From an ecological point of view, it is not suitable for long-term use. In this respect, adoping simpler and more environmentally friendly approaches to rapid synthesis of solution-processable, supported MNPs catalysts is highly anticipated (Citation31–40).
Plant polyphenols represent a large family of compounds consisted of epigallocatechin gallate (EGCG), epicatechin gallate (ECG), epigallocatechin (EGC), and tannic acid (TA), and so on. Inspired by their natural bonding and antioxidant properties, plant polyphenols have recently received extensive attention and research interest in different fields including surface modification, nano-synthesis, composites, to name a few (Citation31, Citation38, Citation41–48). Among many plant polyphenols, green tea polyphenols (TPs) is the most widely studied one because it is the most readily available (Citation49). TPs are rich in multiple pyrogallol and catechol structures which endow it powerful bonding and excellent antioxidant properties (Citation50). Many studies have reported that TPs can be used to modify and stabilize nanomaterials such as carbon nanotubes and graphene due to its powerful surface bonding capacity (Citation51–55). In addition, TPs is also used as a green reducing agent for synthesis of metal nanoparticles, and many metal nanomaterials such as gold, silver, palladium, copper and iron have been reported successively (Citation56–58). Recently, we reported a graphene-supported silver nanoparticle catalyst in a completely green way by combining dual roles of TPs in surface bonding and reducing activity (Citation59). Compared to graphene, ND can be prepared on a large scale and does not involve complex chemical synthesis procedures, which make it more suitable for use as support of heterogeneous catalyst. Although ND has been widely used as catalyst carriers, modification by TPs and for further reduction and stability of MNPs has not been reported.
In this paper, we demonstrate the feasibility of silver nanoparticles are generated and stabilized in situ on TPs-modified ND surface to prepare Ag-TPND heterogenuous catalyst. In contrast to previous reported chemical reduction method, our strategy is to pre-form a multifunctional TPs coating on the surface of ND utilizing the surface bonding properties of TPs. Then the TPs coating act as a green reducing agent to reduce Ag precursor in situ and stabilizing the formed Ag nanoparticles, obtaining well-distributed Ag-TPND hybrid. Because TPs molecules are pre-modified on the ND surface, the amount of silver nanoparticles can be controlled, which can effectively avoid the aggregation of silver nanoparticles on the ND surface during the preparation process. In addition, due to the super hydrophilicity of TPs molecules, the prepared Ag-TPND catalyst has good water dispersion properties, which is undoubtedly a very competitive feature in heterogeneous catalysis. Next, the reduction of 4-nitrophenol (4-NP) to 4-aminophenol (4-AP) by NaBH4 is adopted as a catalytic model reaction to evaluate the catalytic properties of the synthesized Ag-TPND. The results demonstrate that the as-prepared Ag-TPND hybrid exhibited excellent catalytic performance and cyclic stability toward the reduction of 4-NP by NaBH4 in the aqueous phase.
2. Experimental
2.1. Materials
AgNO3, 4-Nitrophenol (reagent grade) and NaBH4 were received from Sinopharm Chemical Reagent Co., Ltd. (Shanghai, China) and used without further purification. The nanodiamond gray powders was obtained from Henan Union Abrasives Corp, China. Green tea used in this work is Xinyang Maojian tea purchased from local market.
2.2. Surface modification of ND by TPs (TPND)
A transparent TPs water solution was prepared by extraction of green tea according to the method reported in the literature. Green tea (5 g) was extracted with 250 mL of deionized (DI) water at while being continuously stirred for 30 min. After extraction, removing green tea leaves by filtration, and centrifuging the filter solution at 8000 rpm for 30 min to completely remove the insoluble matter, transparent tea polyphenols solution was obtained. Then, ND was modified through the following procedures. 100 mL of new prepared TPs solution with 50 mg of ND powder was added in a 250 mL stand-up flask, sonicating the mixture for 30 min. Then The flask was transferred to a 80°C oil bath and reacted for two more hours with continuously stirring under nitrogen atmosphere. After the reaction was completed, the TPND hybrid was obtained by centrifugal separation. The target product was washed with deionized water in multiple cleaning-centrifugation cycles to remove excessive unmodified TPs and then dried by lyophilization.
2.3. Preparation of Ag-TPND catalyst
The Ag-TPND catalyst was prepared as follows. At first, 5 mg of new prepared TPND powder was dipersed into 10 mL DI water by sonification. Then, 0.5 mL of AgNO3 solution (0.1 M) was added to the dispersion dropwise. The reaction mixture continuously reacted for 12 h at room temperature with mild stirring under nitrogen atmosphere. After that, the Ag-TPND catalyst was obtained by centrifugal separation. The product was washed with DI water repeatedly and dried by lyophilization before use. The content of silver nanoparticles in catalyst determined by Atomic Absorption Spectrometry (AAS) is about 0.15%, which is consistant with the result of XPS. The preparation process of the Ag-TPND catalyst is schematic illuminated in Scheme 1.
2.4. Instruments characterization
FTIR spectral characteristics of the samples grinded with the KBr were performed in reflectance mode with Nicolet Avator 230 spectrometer. Absorption spectra were meseared by a Shimadzu UV-1601PC spectrophotometer in the range of 200–800 nm. The XRD patterns were recorded in a Bruker D8 ADVANCE X-ray diffractometer with Cu Kα (1.541 Å) radiation (40 kV, 30 mA). TEM were performed on a FEI Tecnai G2 F20 microscope with a field-emission gun operating at 200 kV. The sample was prepared by placing a drop of fresh water solution of Ag-TPND on Cu grids precoated with Formvar and carbon films. Thermal dagradation beheviors of pristine ND and TPND in the range of 25–800°C at a rate of 10°C/min were recorded on a NETZSCH 4TGA instrument under 50 mL/min of nitrogen flow. XPS measurement was made using VG Multilab 2000 electron spectrometer from Thermo Scientific using 300 W Al Kα radiation. The Ag contents of the fresh and the recycled catalysts were determined by dissoving the Ag nanoparticles to Ag+ ions using aqua regia and detecting the amount of Ag in supernatant by ICP-AES.
2.5. Catalytic reduction of 4-NP
The model reaction of 4-NP reduction by NaBH4 was performed in aqueous solution in a standard quartz cell. In a typical run, 0.1 mL of aqueous NaBH4 solution was added to 2.9 mL of aqueous solution of 4-NP. After mixing these solutions with nitrogen purging for 10 min to remove the dissolved oxygen, 2 mL of aqueous Ag-TPND suspensions purged with nitrogen was added to the reaction mixture under constant magnetic stirring. The final concentrations of 4-NP, NaBH4 and Ag-TPND catalyst are 0.1 mM, 10 mM and 0.2 mg mL−1. Without delay, the reaction mixture was transferred to a standard quartz cell, and the reduction process was monitored continuously by UV–vis absorption spectra with a120 s of time interval in the range of 200–800 nm.
After finishing the reaction, the Ag-TPND was collected by centrifugation and washed several times with DI water, ethyl acetate and then ether in consecutive cycles. Sonification dispersion was conducted in each cycle for removing the hard-to-wash adsorbents. After that, the catalyst was dried under a nitrogen flow for subsequent cycle experiment. At the same time, the supernatant after the reaction was collected to detect the leaching of silver by AAS.
3. Results and discussion
3.1. Surface modification of ND by TPs
Detonantion ND was modified by TPs in a completely green way. The modification process was testified by using FTIR spectroscopy to monitor the change of surface chemical compostions of ND before and after TPs modification. Industrial-grade detonation ND needs to be purified through oxidization with perchloric acid to remove the non-diamond carbon components. The oxidation process produces a large number of oxygen-containing groups on its surface. For the pristine ND (shown in a), strong absorption peaks at about 1716 cm−1, 3200–3500 cm−1 and 1620–1630 cm−1 were observed. These peaks can be attributed to the absorption vibrations of oxygen-containing groups such as carbonyl (C=O), hydroxyl groups (O–H) stretch and bend respectively. As expected, the vibration signals C–O and C–O–C were also observed in the region of 1000–1250 cm−1 and centered at 1128 cm−1 with a wide weak absorption. In addition, the pristine ND exhibits obvious signals of C–H (2970–2856 cm−1 stretch and 1403 cm−1 bend) and C=C stretching (1617 cm−1), indicating the characteristic structure of graphite on the surface of the ND. Compared to the pristine ND, TPND shows very different spectral characteristics as indicated in (b). The peak of C=O groups, at about 1716 cm−1 weakened obviously, while the absoption peak of C=C stretching at 1617 cm−1 enhanced markedly, indicating that TPs can partially reduce the oxygen-containing groups of ND. At the same time, the absorption peaks attributing to most oxygen-containing groups are still preserved although their intensities reduced to a certain extent, demonstrating that there were much of oxygen containing groups still existed on the surface of TPND. Meanwhile, there are a lot of new absorption peaks originated from the adsorbed TPs appeared over the whole scanning range, testifying the successful modification of TPs. From these evidences, we decuce that the residual oxygen-containing groups of ND and the adsorbed hydroxyl-rich TPs on the surface of nanodiamond contribute commonly to the outstanding water dispersibility of TPND.
The successful modification of ND by TPs was further examined through thermal degradation analysis as shown in . Compared to the prestine ND, about 5.3% of weight loss was observed for the TPND sample with the increase of temperature to 200°C. This weight loss can be attributed to the loss of water adsorbed by hydrophilic groups of TPs. As the temperature continues to rise, a quick weight loss begins to appear until 400°C. This thermal degradation process, which is clearly different from ND, should be from the decomposition of the TPs adsorbed on the surface of ND. As shown in the arrows in the figure, the amount of tea polyphenols adsorbed on the ND surface is about 15.4%. As the temperature continues to rise to 800°C, TPND shows almost the same thermal degradation trajectory as that of ND, indicating that Tea polyphenols have been completely thermally degraded. The results fully demonstrate the successful modification of TPs on the ND surface, and also show that TPs modification does not affect the surface structure and chemical composition of ND, so its thermal degradation behavior is not affected.
3.2. Synthesis and characterization of Ag-TPND catalyst
Despite the presence of a large number of oxygen-containing functional groups, ND is difficult to exist in a dispersed state in different solvents. As shown in , the ultrasonic dispersed ND in the water is completely precipitated after 1 h (inset (a) in ). However, after TPs modification, the TPND can be dispersed in water to form an almost transparent light yellow solution that can stabilize for more than one months (inset (b) in ). Not only that, this TPs stablized ND can reduce the silver precursor in situ to silver nanoparticles on the surface of ND. Importantly, this Ag-TPND can still be well dispersed in the water as shown in inset (c) of . Silver nanoparticles have characteristic surface plasma resonance (SPR) absorption, so the successful preparation of the Ag-TPND hybrid can be proved by UV-Vis absorption spectroscopy. As shown in , the spectrum of Ag–TPND showed a wide weak absorption peak centered at ca. 450 nm ascribing to the SPR absorption of Ag nanoparticles compared with the spectra of ND and TPND, confirming the formation of Ag–TPND nanohybrid.
Figure 3. UV–Vis spectra of ND (▴), TPND (●) and Ag-TPND hybrid (▪) in water. The inset shows the water dispersibility of (a) pristine ND, (b) TPND and (c) Ag-TPND hybrid.
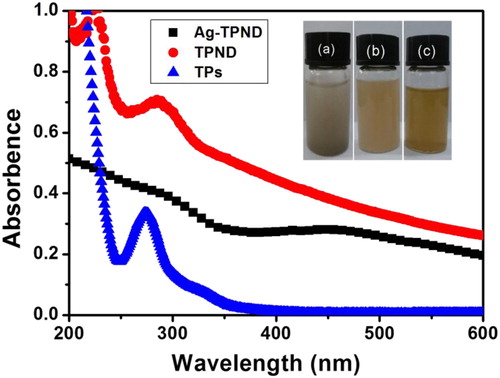
In order to illustrate the formation of silver nanoparticles and their crystal structure, XRD was used to characterize the obtained samples. shows the results of XRD comparison of TPND and Ag-TPND, and it can be seen from the two graphs that there are four characteristic peaks in Ag-TPND different from TPND, located at 2θ values of about 38°, 46°, 67°, and 77°, respectively. The absorption peaks of these locations should be attributed to the characteristic lattice planes of (111), (200), (220) and (311) resulting from the face centered cubic crystalline Ag (JCPDS, Card no. 05-0681). The average size of the Ag NPs are estimated from the half-widths of (111) peaks by using the Scherrer equation (Citation2, Citation60),Where D is the average particle size,
is the shape factor (constant 0.9),
is the X-ray wavelength (1.5406 Å), β is the full width at half maximum of the peak (0.449), and θ is the diffraction angle. The mean crystallite size of Ag nanoparticles calculated according to the Scherrer equation is about 3.92 nm. The XRD results fully demonstrate the generation of silver crystals and further confirm the successful preparation of Ag–TPND nanohybrid.
TPs molecules of TPND were utilized as green reducing agents for in situ preparing Ag nanoparticles and stabilizing them. The Ag nanoparticles are anchored to the surface of the ND through the bonding and stabilizing effect of TPs. The detailed morphology and Ag nanoparticles distribution of Ag-TPND nanohybrid was examined by TEM. A typical TEM image of Ag-TPND nanohybrid is recorded in (a). It can be seen that a large number of very small silver nanoparticles (black dots) are evenly distributed on the surface of the ND. The average particle size of Ag nanoparticles, obtained by randomly statistic analysis of 100 particles, is approximately 5 nm with size ranging from 2 to 10 nm, which is in good agreement with but slightly larger than the average size calculated by XRD. This difference also offen appeared in other reported documents. The magnified image shown in (b) reveals that the Ag nanoparticles with clear monocrystalline structure are strongly adhered on the surface of ND forming a stable binding state between them. The identified lattice spacing of 0.234 nm correspond to (111) lattice plane of Ag nanoparticles further testified the successful preparation of Ag-TPND hybrid.
In order to verify the surface modification of ND and subsequently in situ reduction of silver precursor to Ag-TPND hybrid, the surface element characterization of samples was analyzed by XPS. The spectra of XPS element surveys are shown in . As anticipated, XPS analyses of the pristine ND and TPND showed the presence of carbon and oxygen. However, XPS spectrum of Ag-TPND revealed additional peaks at 368.4 and 374.4 eV corresponding to the binding energy of Ag crystals. In order to elucidate the oxidation state of the Ag element, the Ag 3d regions of the Ag-TPND hybrid was further carried out and showed in inset. As expected, two characteristic peaks at binding energies of 368.4 and 374.4 eV appeared, corresponding to the Ag 3d5/2 and Ag 3d3/2 of metallic Ag (Ag0), respectively (Citation61). displays the detailed elemental compositions of pristine ND, TPND and Ag-TPND. A careful analysis of the data in shows that after TPs modification, the proportion of carbon decreased from 93.67% to 78.43%, while the proportion of oxygen increased from 6.33% to 18.79%, respectively, indicating the succesful modification of ND by TPs. Comparing elemental surveys of Ag-TPND with that of TPND, it is worthy noting that the formation of silver nanoparticles had almost no significant effect on surface carbon and oxygen composition. These evidences fully indicate that the oxygen groups on the surface give excellent water dispersibility of TPND and Ag-TPND. Moreover, the Ag content in Ag-TPND estimated from the peak area is about 0.15%, which is almost consistant with the result from ICP-AES detection (0.13%). This slight difference may be attributed to the incomoplete dissolusion of supported Ag and Ag+ ions adsorption on the surface of ND during the sampling process for ICP-AES.
Figure 6. XPS survey of pristine ND, TPND and Ag-TPND hybrid. The inset shows the XPS survey of Ag 3d regions of Ag-TPND hybrid.
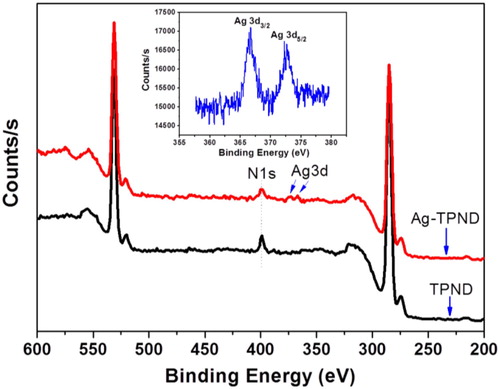
Table 1. XPS data of ND, TPND and Ag-TPND.
3.3. Catalytic reduction of 4-NP
Nitro phenol compounds are found in many pesticides and are harmful to the environment and human body. Since 4-aminophenol (4-NP) is an important pharmaceutical intermediate, catalytic conversion of 4-NP to 4-aminophenol (4-NP) can not only reduce its environmental impact, but also achieve its recycling. Therefore, 4-NP reduction reaction is often used as an important model reaction to evaluate catalyst activity. In this work, to probe the catalytic activity of our newly synthesized Ag-TPND catalylst, catalytic conversion of 4-NP was also chosen as an exemplary reaction and traced by UV–vis absortion spectroscopy. It could be seen from that the 4-NP exhibited a typical absorption peak at about 400 nm after adding NBH4 aqueous solution, testifing the formation of 4-nitrophenolate ion in the alkali medium. In the absence of Ag-TPND catalyst or in the presence of TPND only, the absorption peak at 400 nm remain constant for a long time, indicating that the NaBH4 itself or TPND cannot catalyize the reduction 4-nitrophenolate ion. When the Ag-TPND is added, the catalytic reaction begins immediately. As shown in , the intensity of the absorption peak at 400 nm continues to decrease, while a new absorption peak appears at 301 nm, and the intensity increases gradually. This growing new absorption peak is the characteristic absorption peak of the newly generated 4-AP. More importantly, no induction period was observed, and it can be completed in a very short period of time (16 min) with no additional absorption peaks appearing.
Figure 7. (A) Tracing the reduction process of 4-NP to 4-AP by UV-Vis absorption catalyzed by Ag-TPND; (B) Determination of reaction rate constant by the plot of ln(Ct/C0) against the reaction time.
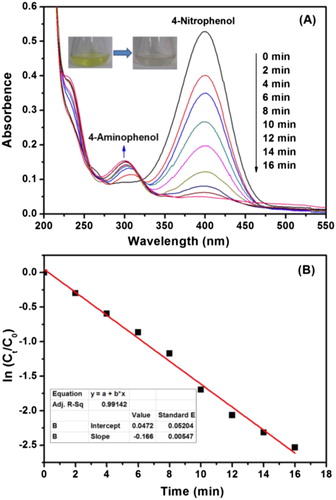
Because NaBH4 is greatly excessive (NaBH4/4-NP = 100:1) during the whole reduction process, the reduction reaction rate can be considered to be a pseudo-first-order kinetic relationship with 4-NP concentration, but not related to the concentration of NaBH4. As anticipated, a good linear correlation of ln (Ct/C0) versus time was obtained as shown in (B). The rate constant (k) could be determined from the linear plot of ln (Ct/C0) versus reduction time to be 27.7 × 10−3 s−1. To evaluate and compare the catalytic activity of different catalysts, the standardized catalyst activity constant A = k/m was used to evaluate the catalytic performance of the current catalyst, where k is rate constant, m is the catalyst mass of 1 mL reaction solution (). As compared in , the current Ag-TPND catalyst is better than most of the Ag-based catalysts reported in the literature (Citation43, Citation62–65). To futher evaluate the intrinsic catalytic activity of the catalyst, initial turnover frequency (TOF) values in the first two minutes, that is, the number of 4-NP molecules reacted per mol Ag per hour, were calculated to be 256.2 h−1. This value is slight lower than the recently reported TOF value (420 h−1) of the Ag@CNC catalyst prepared in a green way (Citation14). Nevertheless, compared to Ag@CNC, the silmpler synthesis procedure and cost effectiveness of ND make Ag-ND catalyst to be economically attractive.
Table 2. Comparison of the catalytic activity towards 4-NP reduction for various Ag-based catalysts.
Recycling is an important index to investigate the stability of catalyst activity. Next, the cycle stability of the Ag-TPND catalyst was carried out for same reaction time by tracing the absorption of 4-NP at 400 nm. As can be seen from , even after five recycles, the activity of the catalyst is basically stable compared with that of the fresh catalyst. Nevertheless, with the increase of recycle times, the 4-NP conversion rates decreased slightly continuously from 92.1% to about 83.6% within 16 min of reaction time. In order to explore the reasons for the decrease of catalyst activity, the leaching of silver nanoparticles was traced by employing a hot filtration test.8 Leaving the reduction reaction of 4-NP with NaBH4 in the presence Ag-TPND catalyst in aqueous solution performed for 2 min, then the dispersed catalyst was removed by high-speed centrifugation. The catalyst-free reacting system was continued to react for 14 min under the same conditions with sampling test every 2 min. The absorption of 4-NP at 400 nm remain unchanged after removing the Ag-TPND catalyst, indicating that negligible amount of silver nanoparticles was leached out from the ND support. Moreover, AAS detection was also performed to monitor the leaching amount of silver in the centrifuge supernatant after each recycle. The results showed that traces of Ag were detected with below 0.03 ppm for the first recycle and 0.35 ppm for the fifth recycle, indicating that as the number of cycles increases, the amount of silver lost to the solution increases gradually. In addition, the Ag content of the recycled catalysts are treated with aqua regia to measure the concentration of Ag+ by ICP-AES. The result showed that the Ag content of the catalyst after the first recycle remained almost 0.13%, but after five recycles, the Ag content declined to about 0.11%. We infer that the small loss of silver from the ND surface is due to the coordination interaction between the amino groups of the reduction product and silver nanoparticles (as shown in ). In addition, another likely cause of the decline in catalyst activity may be from the change of catalyst dispersion and aggregation. To this end, we studied the dispersion and aggregation of the catalyst on the ND surface after five recycles through TEM observation. As can be seen from inset, after five cycles, Ag is mostly retained on the ND surface, but the particles have a slight aggregation phenomenon compared to the newly prepared catalyst. This aggregation behevior of Ag nanoparticles should be another reason for the decline in recycling activity.
Figure 9. The catalytic reduction process of 4-NP to 4-AP and the proposed deactivition mechanism of the Ag-TPND catalyst after multiple recycle.
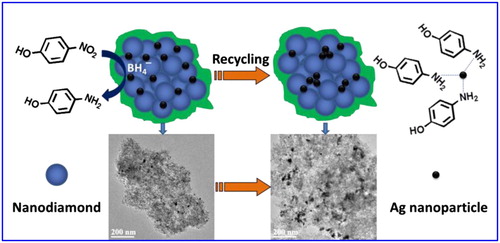
The reduction mechanism of 4-nitrophenol to 4-aminophenol by NaBH4 in the presence of Ag-TPND catalyst is proposed in terms of the Langmuir–Hinshelwood (LH) model (Citation66, Citation67) and illustrated in . The catalytic reduction proceeds on the surface of the Ag nanoparticles. ions first transfer a surface-hydrogen species to the surface of the Ag nanoparticles through reversible adsorption process. Concomitantly, 4-NP molecules can also adsorb on the surface of the Ag nanoparticles reversibly. The reversible adsorption/desorption of both reagents on the Ag surface is fast and can be modeled in terms of a Langmuir isotherm process. In the catalytic reduction of 4-NP, the contact between 4-NP and silver nanoparticles is one of the most critical steps. At the same time, the surface-hydrogen species formed by the decomposition of surface adsorbed
ions participate in the reduction reaction which is the rate-determining step. Adsorption and desorption of reactant molecules on catalyst surface are the key factors affecting the efficiency of heterogeneous catalytic reaction. Moreover, the large specific surface area and SP2 carbon structure of ND facilitate the adsorption of the 4-NP through electrostatic interactions. This adsorption increases the effective concentration of the 4-NP near the Ag nanoparticles, making the transfer of hydrogen species to 4-NP on the surface of silver nanoparticles more efficient.
4. Conclusions
In summary, surface modification of ND by TPs and subsequent green reduction have been conducted to prepare a water dispersible Ag-TPND hybrid. This hybrid was successfully used as an efficient catalyst to the reduction of 4-NP in the presence of NaBH4 with higher rate constants of 0.166 min−1 and TOF value of 260.2 h−1. Albeit with slight loss in activity with increase of the recycling times, reuse of the catalysts can be achieved through simple centrifugal separation with very low silver leaching and aggregation. This green procedure offers attractive alternatives for designing highly active heterogeneous catalysts with novel nanostructure and tailored catalytic performances by bio-based derivatives.
Acknowledgments
Financial support from University Science and Technology innovation Talent support plan of Henan Province (18HASTIT038) and Key projects of science and technology research of the Education Department of Henan Province (17A150028) is greatly acknowledged.
Disclosure statement
No potential conflict of interest was reported by the authors.
Notes on contributors
Zhimin Wang is an associate professor of Chemsitry in School of Science at Henan Agricultural University where he teaches Polymer Chemistry and Green Chemistry and Sustainability. Dr Wang’s research interests are mainly focused on polymer chemistry, nanoscience and technololy, catalysis and bio-based chemicals.
Yuan Huang is a postgraduate in School of Chemistry and College of life Sciences, Henan Agricultural University.
Dongcan Lv is a senior lecturer in School of Chemistry, Henan Agricultural University.
Guangce Jiang is a senior lecturer in School of Chemistry, Henan Agricultural University.
Fengjiao Zhang is a postgraduate in School of Chemistry and College of life Sciences, Henan Agricultural University.
Andong Song is a professor of microbiology and biochemistry in College of life Sciences at Henan Agricultural University where he teaches Microbiology, Biochemistry, and Enzymatic hydrolysis and fermentation. Dr Song’s research interests are the development of green and efficient methods for Biomass transformation and utilization.
Additional information
Funding
References
- Abbas Khakiani, B.; Pourshamsian, K.; Veisi, H. A Highly Stable and Efficient Magnetically Recoverable and Reusable Pd Nanocatalyst in Aqueous Media Heterogeneously Catalysed Suzuki C-C Cross-Coupling Reactions. Appl. Organomet. Chem. 2015, 29 (5), 259–265. doi:10.1002/aoc.3282.
- Liao, G.; Li, Q.; Zhao, W.; Pang, Q.; Gao, H.; Xu, Z. In-Situ Construction of Novel Silver Nanoparticle Decorated Polymeric Spheres as Highly Active and Stable Catalysts for Reduction of Methylene Blue Dye. Appl. Catal. A Gen. 2018, 549, 102–111. doi:10.1016/j.apcata.2017.09.034.
- Veisi, H.; Razeghi, S.; Mohammadi, P.; Hemmati, S. Silver Nanoparticles Decorated on Thiol-Modified Magnetite Nanoparticles (Fe3O4/SiO2-Pr-S-Ag) as a Recyclable Nanocatalyst for Degradation of Organic Dyes. Mater. Sci. Eng. C. 2019, 97 (Dec 2018), 624–631. doi:10.1016/j.msec.2018.12.076.
- Xue, W.; Huang, D.; Zeng, G.; Wan, J.; Zhang, C.; Xu, R.; Cheng, M.; Deng, R. Nanoscale Zero-Valent Iron Coated with Rhamnolipid as an Effective Stabilizer for Immobilization of Cd and Pb in River Sediments. J. Hazard. Mater. 2018, 341, 381–389. doi:10.1016/j.jhazmat.2017.06.028.
- Yang, H.; Bradley, S.J.; Wu, X.; Chan, A.; Waterhouse, G.I.N.; Nann, T.; Zhang, J.; Kruger, P.E.; Ma, S.; Telfer, S.G. General Synthetic Strategy for Libraries of Supported Multicomponent Metal Nanoparticles. ACS Nano. 2018, 12 (5), 4594–4604. doi:10.1021/acsnano.8b01022.
- Sudarsanam, P.; Zhong, R.; Van den Bosch, S.; Coman, S.M.; Parvulescu, V.I.; Sels, B.F. Functionalised Heterogeneous Catalysts for Sustainable Biomass Valorisation. Chem. Soc. Rev. 2018. doi:10.1039/C8CS00410B.
- Meirer, F.; Weckhuysen, B.M. Spatial and Temporal Exploration of Heterogeneous Catalysts with Synchrotron Radiation. Nat. Rev. Mater. 2018, 3 (9), 324–340. doi:10.1038/s41578-018-0044-5.
- Veisi, H.; Hamelian, M.; Hemmati, S. Palladium Anchored to SBA-15 Functionalized with Melamine-Pyridine Groups as a Novel and Efficient Heterogeneous Nanocatalyst for Suzuki–Miyaura Coupling Reactions. J. Mol. Catal. A Chem. 2014, 395, 25–33. doi:10.1016/j.molcata.2014.07.030.
- Liao, G.; Zhao, W.; Li, Q.; Pang, Q.; Xu, Z. Novel Poly(Acrylic Acid)-Modified Tourmaline/Silver Composites for Adsorption Removal of Cu(II) Ions and Catalytic Reduction of Methylene Blue in Water. Chem. Lett. 2017, 46 (11), 1631–1634. doi:10.1246/cl.170785.
- Veisi, H.; Najafi, S.; Hemmati, S. Pd(II)/Pd(0) Anchored to Magnetic Nanoparticles (Fe3O4) Modified with Biguanidine-Chitosan Polymer as a Novel Nanocatalyst for Suzuki-Miyaura Coupling Reactions. Int. J. Biol. Macromol. 2018, 113 (Ii), 186–194. doi:10.1016/j.ijbiomac.2018.02.120.
- Yazdankhah, M.; Veisi, H.; Hemmati, S. In Situ Immobilized Palladium Nanoparticles (Pd NPs) on Fritillaria Imperialis Flower Extract-Modified Graphene and Their Catalytic Activity for Reduction of 4-Nitrophenol. J. Taiwan Inst. Chem. Eng. 2018, 91, 38–46. doi:10.1016/j.jtice.2018.05.043.
- Liao, G.; Gong, Y.; Yi, C.; Xu, Z. Soluble, Antibaterial, and Anticorrosion Studies of Sulfonated Polystyrene/Polyaniline/Silver Nanocomposites Prepared with the Sulfonated Polystyrene Template. Chinese J. Chem. 2017, 35 (7), 1157–1164. doi:10.1002/cjoc.201600816.
- Liu, J.; Plog, A.; Groszewicz, P.; Zhao, L.; Xu, Y.; Breitzke, H.; Stark, A.; Hoffmann, R.; Gutmann, T.; Zhang, K.; Buntkowsky, G. Design of a Heterogeneous Catalyst Based on Cellulose Nanocrystals for Cyclopropanation: Synthesis and Solid-State NMR Characterization. Chem. - A Eur. J. 2015, 21 (35), 12414–12420. doi:10.1002/chem.201501151.
- Eisa, W.H.; Abdelgawad, A.M.; Rojas, O.J. Solid-State Synthesis of Metal Nanoparticles Supported on Cellulose Nanocrystals and their Catalytic Activity. ACS Sustain. Chem. Eng. 2018, 6 (3), 3974–3983. doi:10.1021/acssuschemeng.7b04333.
- Veisi, H.; Kazemi, S.; Mohammadi, P.; Safarimehr, P.; Hemmati, S. Catalytic Reduction of 4-Nitrophenol Over Ag Nanoparticles Immobilized on Stachys Lavandulifolia Extract-Modified Multi Walled Carbon Nanotubes. Polyhedron. 2019, 157, 232–240. doi:10.1016/j.poly.2018.10.014.
- Pérez-Mayoral, E.; Calvino-Casilda, V.; Soriano, E. Metal-Supported Carbon-Based Materials: Opportunities and Challenges in the Synthesis of Valuable Products. Catal. Sci. Technol. 2016, 6 (5), 1265–1291. doi:10.1039/c5cy01437a.
- Chen, T.; Xiong, Y.; Qin, Y.; Yang, H.; Zhang, P.; Ye, F. Facile Synthesis of Low-Cost Biomass-Based γ-Fe2O3/C for Efficient Adsorption and Catalytic Degradation of Methylene Blue in Aqueous Solution. RSC Adv. 2017, 7 (1), 336–343. doi:10.1039/c6ra24900k.
- Deng, D.; Novoselov, K.S.; Fu, Q.; Zheng, N.; Tian, Z.; Bao, X. Catalysis with Two-Dimensional Materials and Their Heterostructures. Nat. Nanotechnol. 2016, 11 (3), 218–230. doi:10.1038/nnano.2015.340.
- Khan, M.; Tahir, M.N.; Adil, S.F.; Khan, H.U.; Siddiqui, M.R.H.; Al-Warthan, A.A.; Tremel, W. Graphene Based Metal and Metal Oxide Nanocomposites: Synthesis, Properties and Their Applications. J. Mater. Chem. A. 2015, 3 (37), 18753–18808. doi:10.1039/c5ta02240a.
- Nanda, J.; Biswas, A.; Adhikari, B.; Banerjee, A. A Gel-Based Trihybrid System Containing Nanofibers, Nanosheets, and Nanoparticles: Modulation of the Rheological Property and Catalysis. Angew. Chemie - Int. Ed. 2013, 52 (19), 5041–5045. doi:10.1002/anie.201301128.
- Huang, H.; Wang, X. Recent Progress on Carbon-Based Support Materials for Electrocatalysts of Direct Methanol Fuel Cells. J. Mater. Chem. A. 2014, 2 (18), 6266–6291. doi:10.1039/c3ta14754a.
- Yan, Y.; Miao, J.; Yang, Z.; Xiao, F.X.; Yang, H.B.; Liu, B.; Yang, Y. Carbon Nanotube Catalysts: Recent Advances in Synthesis, Characterization and Applications. Chem. Soc. Rev. 2015, 44 (10), 3295–3346. doi:10.1039/c4cs00492b.
- Mao, H.; Shen, Y.; Zhang, Q.; Ulaganathan, M.; Zhao, S.; Yang, Y.; Hng, H.H. Highly Active and Stable Heterogeneous Catalysts Based on the Entrapment of Noble Metal Nanoparticles in 3D Ordered Porous Carbon. Carbon N. Y. 2016, 96 (32), 75–82. doi:10.1016/j.carbon.2015.09.057.
- Navalon, S.; Martin, R.; Alvaro, M.; Garcia, H. Sunlight-Assisted Fenton Reaction Catalyzed by Gold Supported on Diamond Nanoparticles as Pretreatment for Biological Degradation of Aqueous Phenol Solutions. ChemSusChem. 2011, 4 (5), 650–657. doi:10.1002/cssc.201000453.
- Navalon, S.; de Miguel, M.; Martin, R.; Alvaro, M.; Garcia, H. Enhancement of the Catalytic Activity of Supported Gold Nanoparticles for the Fenton Reaction by Light. J. Am. Chem. Soc. 2011, 133 (7), 2218–2226. doi:10.1021/ja108816p.
- Manickam-Periyaraman, P.; Espinosa, S.M.; Espinosa, J.C.; Navalón, S.; Subramanian, S.; Álvaro, M.; García, H. Dyes Decolorization Using Silver Nanoparticles Supported on Nanometric Diamond as Highly Efficient Photocatalyst Under Natural Sunlight Irradiation. J. Environ. Chem. Eng. 2016, 4 (4), 4485–4493. doi:10.1016/j.jece.2016.10.011.
- Ismaili, H.; Workentin, M.S. Covalent Diamond-Gold Nanojewel Hybrids via Photochemically Generated Carbenes. Chem. Commun. (Camb). 2011, 47 (27), 7788–7790. doi:10.1039/c1cc12125a.
- Krueger, A.; Lang, D. Functionality Is Key: Recent Progress in the Surface Modification of Nanodiamond. Adv. Funct. Mater. 2012, 22 (5), 890–906. doi:10.1002/adfm.201102670.
- Meinhardt, T.; Lang, D.; Dill, H.; Krueger, A. Pushing the Functionality of Diamond Nanoparticles to New Horizons: Orthogonally Functionalized Nanodiamond Using Click Chemistry. Adv. Funct. Mater. 2011, 21 (3), 494–500. doi:10.1002/adfm.201001219.
- Dördelmann, G.; Meinhardt, T.; Sowik, T.; Krueger, A.; Schatzschneider, U. CuAAC Click Functionalization of Azide-Modified Nanodiamond with a Photoactivatable CO-Releasing Molecule (PhotoCORM) Based on [Mn(CO)3(Tpm)]+. Chem. Commun. (Camb). 2012, 48 (94), 11528–11530. doi:10.1039/c2cc36491c.
- Veisi, H.; Nasrabadi, N.H.; Mohammadi, P. Biosynthesis of Palladium Nanoparticles as a Heterogeneous and Reusable Nanocatalyst for Reduction of Nitroarenes and Suzuki Coupling Reactions. Appl. Organomet. Chem. 2016, 30 (11), 890–896. doi:10.1002/aoc.3517.
- Veisi, H.; Safarimehr, P.; Hemmati, S. Oxo-Vanadium Immobilized on Polydopamine Coated-Magnetic Nanoparticles (Fe3O4): A Heterogeneous Nanocatalyst for Selective Oxidation of Sulfides and Benzylic Alcohols with H 2 O 2. J. Taiwan Inst. Chem. Eng. 2018, 88, 8–17. doi:10.1016/j.jtice.2018.03.051.
- Taheri, S.; Veisi, H.; Hekmati, M. Application of Polydopamine Sulfamic Acid-Functionalized Magnetic Fe3O4 Nanoparticles (Fe3O4 @PDA-SO 3 H) as a Heterogeneous and Recyclable Nanocatalyst for the Formylation of Alcohols and Amines Under Solvent-Free Conditions. New J. Chem. 2017, 41 (12), 5075–5081. doi:10.1039/C7NJ00417F.
- Farzad, E.; Veisi, H. Fe3O4/SiO2 Nanoparticles Coated with Polydopamine as a Novel Magnetite Reductant and Stabilizer Sorbent for Palladium Ions: Synthetic Application of Fe3O4 /SiO2 @PDA/Pd for Reduction of 4-Nitrophenol and Suzuki Reactions. J. Ind. Eng. Chem. 2018, 60, 114–124. doi:10.1016/j.jiec.2017.10.017.
- Veisi, H.; Ghorbani, M.; Hemmati, S. Sonochemical in Situ Immobilization of Pd Nanoparticles on Green Tea Extract Coated Fe3O4 Nanoparticles: An Efficient and Magnetically Recyclable Nanocatalyst for Synthesis of Biphenyl Compounds Under Ultrasound Irradiations. Mater. Sci. Eng. C. 2019, 98, 584–593. doi:10.1016/j.msec.2019.01.009.
- Veisi, H.; Pirhayati, M.; Kakanejadifard, A.; Mohammadi, P.; Abdi, M.R.; Gholami, J.; Hemmati, S. In Situ Green Synthesis of Pd Nanoparticles on Tannic Acid-Modified Magnetite Nanoparticles as a Green Reductant and Stabilizer Agent: Its Application as a Recyclable Nanocatalyst (Fe3O4 @TA/Pd) for Reduction of 4-Nitrophenol and Suzuki Reactions. ChemistrySelect. 2018, 3 (6), 1820–1826. doi:10.1002/slct.201702869.
- Shahriary, M.; Veisi, H.; Hekmati, M.; Hemmati, S. In Situ Green Synthesis of Ag Nanoparticles on Herbal Tea Extract (Stachys Lavandulifolia)-Modified Magnetic Iron Oxide Nanoparticles as Antibacterial Agent and Their 4-Nitrophenol Catalytic Reduction Activity. Mater. Sci. Eng. C. 2018, 90, 57–66. doi:10.1016/j.msec.2018.04.044.
- Das, S.; Chakraborty, J.; Chatterjee, S.; Kumar, H. Prospects of Biosynthesized Nanomaterials for the Remediation of Organic and Inorganic Environmental Contaminants. Environ. Sci. Nano. 2018, 180 (1–3), 98–105. doi:10.1039/C8EN00799C.
- Ismail, M.; Khan, M.I.; Bahadar, S.; Akhtar, K.; Ali, M.; Asiri, A.M. Catalytic Reduction of Picric Acid, Nitrophenols and Organic Azo Dyes via Green Synthesized Plant Supported Ag Nanoparticles. J. Mol. Liq. 2018, 268, 87–101. doi:10.1016/j.molliq.2018.07.030.
- Chouhan, N.; Ameta, R.; Kumar, R. Biogenic Silver Nanoparticles From Trachyspermum Ammi (Ajwain) Seeds Extract for Catalytic Reduction of p -Nitrophenol to p -Aminophenol in Excess of NaBH 4. J. Mol. Liq. 2017, 230, 74–84. doi:10.1016/j.molliq.2017.01.003.
- Hemmati, S.; Mehrazin, L.; Ghorban, H.; Garakani, S.H.; Mobaraki, T.H.; Mohammadi, P.; Veisi, H. Green Synthesis of Pd Nanoparticles Supported on Reduced Graphene Oxide, Using the Extract of Rosa Canina Fruit, and Their Use as Recyclable and Heterogeneous Nanocatalysts for the Degradation of Dye Pollutants in Water. RSC Adv. 2018, 8 (37), 21020–21028. doi:10.1039/C8RA03404D.
- Lebaschi, S.; Hekmati, M.; Veisi, H. Green Synthesis of Palladium Nanoparticles Mediated by Black Tea Leaves (Camellia Sinensis) Extract: Catalytic Activity in the Reduction of 4-Nitrophenol and Suzuki-Miyaura Coupling Reaction Under Ligand-Free Conditions. J. Colloid Interface Sci. 2017, 485, 223–231. doi:10.1016/j.jcis.2016.09.027.
- Veisi, H.; Ghorbani, F. Iron Oxide Nanoparticles Coated with Green Tea Extract as a Novel Magnetite Reductant and Stabilizer Sorbent for Silver Ions: Synthetic Application of Fe3O4@green Tea/Ag Nanoparticles as Magnetically Separable and Reusable Nanocatalyst for Reduction of 4-. Appl. Organomet. Chem. 2017, 31 (10), e3711. doi:10.1002/aoc.3711.
- Veisi, H.; Ghadermazi, M.; Naderi, A. Biguanidine-Functionalized Chitosan to Immobilize Palladium Nanoparticles as a Novel, Efficient and Recyclable Heterogeneous Nanocatalyst for Suzuki-Miyaura Coupling Reactions. Appl. Organomet. Chem. 2016, 30 (5), 341–345. doi:10.1002/aoc.3437.
- Khan, M.; Shaik, M.R.; Adil, S.F.; Khan, S.T.; Al-Warthan, A.; Siddiqui, M.R.H.; Tahir, M.N.; Tremel, W. Plant Extracts as Green Reductants for the Synthesis of Silver Nanoparticles: Lessons from Chemical Synthesis. Dalt. Trans. 2018, 47 (35), 11988–12010. doi:10.1039/C8DT01152D.
- Fierascu, I.; Georgiev, M.I.; Ortan, A.; Fierascu, R.C.; Avramescu, S.M.; Ionescu, D.; Sutan, A.; Brinzan, A.; Ditu, L.M. Phyto-Mediated Metallic Nano-Architectures via Melissa Officinalis L.: Synthesis, Characterization and Biological Properties. Sci. Rep. 2017, 7 (1), 1–3. doi:10.1038/s41598-017-12804-7.
- Dauthal, P.; Mukhopadhyay, M. Noble Metal Nanoparticles: Plant-Mediated Synthesis, Mechanistic Aspects of Synthesis, and Applications. Ind. Eng. Chem. Res. 2016, 55 (36), 9557–9577. doi:10.1021/acs.iecr.6b00861.
- Lee, D.W.; Jin, M.H.; Lee, Y.J.; Park, J.H.; Lee, C.B.; Park, J.S. Reducing-Agent-Free Instant Synthesis of Carbon-Supported Pd Catalysts in a Green Leidenfrost Droplet Reactor and Catalytic Activity in Formic Acid Dehydrogenation. Sci. Rep. 2016, 6 (April), 1–9. doi:10.1038/srep26474.
- Nakamura, G.; Narimatsu, K.; Niidome, Y.; Nakashima, N. Green Tea Solution Individually Solubilizes Single-Walled Carbon Nanotubes. Chem. Lett. 2007, 36, 1140–1141. doi:10.1246/cl.2007.1140.
- Wang, Y.; Ho, C.T. Polyphenols Chemistry of Tea and Coffee: A Century of Progress. J. Agric. Food Chem. 2009, 57 (18), 8109–8114. doi:10.1021/jf804025c.
- Nadagouda, M.N.; Varma, R.S. Green Synthesis of Silver and Palladium Nanoparticles at Room Temperature using Coffee and Tea Extract. Green Chem. 2008, 10 (8), 859. doi:10.1039/b804703k.
- Iravani, S. Green Synthesis of Metal Nanoparticles Using Plants. Green Chem. 2011, 13 (10), 2638. doi:10.1039/c1gc15386b.
- Akhtar, M.S.; Panwar, J.; Yun, Y.-S. Biogenic Synthesis of Metallic Nanoparticles by Plant Extracts. ACS Sustain. Chem. Eng. 2013, 1 (6), 591–602. doi:10.1021/sc300118u.
- Brumbaugh, A.D.; Cohen, K.A.; St. Angelo, S.K. Ultrasmall Copper Nanoparticles Synthesized with a Plant Tea Reducing Agent. ACS Sustain. Chem. Eng. 2014, 2 (8), 1933–1939. doi:10.1021/sc500393t.
- Nadagouda, M.N.; Iyanna, N.; Lalley, J.; Han, C.; Dionysiou, D.D.; Varma, R.S. Synthesis of Silver and Gold Nanoparticles Using Antioxidants from Blackberry, Blueberry, Pomegranate, and Turmeric Extracts. ACS Sustain. Chem. Eng. 2014, 2 (7), 1717–1723. doi:10.1021/sc500237k.
- Chen, Y.; Lee, Y.D.; Vedala, H.; Allen, B.L.; Star, A. Exploring the Chemical Sensitivity of a Carbon Nanotube/Green Tea Composite. ACS Nano. 2010, 4 (11), 6854–6862. doi:10.1021/nn100988t.
- Wang, Y.; Shi, Z.; Yin, J. Facile Synthesis of Soluble Graphene via a Green Reduction of Graphene Oxide in Tea Solution and Its Biocomposites. ACS Appl. Mater. Interfaces. 2011, 3 (4), 1127–1133. doi:10.1021/am1012613.
- Wang, Z.; Xu, C.; Gao, G.; Li, X. Facile Synthesis of Well-Dispersed Pd–graphene Nanohybrids and their Catalytic Properties in 4-Nitrophenol Reduction. RSC Adv. 2014, 4 (26), 13644. doi:10.1039/c3ra47721e.
- Wang, Z.; Xu, C.; Li, X.; Liu, Z. In Situ Green Synthesis of Ag Nanoparticles on Tea Polyphenols-Modified Graphene and Their Catalytic Reduction Activity of 4-Nitrophenol. Colloids Surfaces A Physicochem. Eng. Asp. 2015, 485, 102–110. doi:10.1016/j.colsurfa.2015.09.015.
- Patterson, A. The Scherrer Formula for X-Ray Particle Size Determination. Phys. Rev. 1939, 56, 978–982.
- Zhou, Y.; Yang, J.; He, T.; Shi, H.; Cheng, X.; Lu, Y. Highly Stable and Dispersive Silver Nanoparticle-Graphene Composites by a Simple and Low-Energy-Consuming Approach and Their Antimicrobial Activity. Small. 2013, 9 (20), 3445–3454. doi:10.1002/smll.201202455.
- Fujishima, A.; Honda, K. Synthesis and Catalytic Application of Nanostructured Silver Dendrites. J. Phys. Chem. C. 2008, 112 (4), 1304–1304. doi:10.1021/jp7116449.
- Tang, S.; Vongehr, S.; Meng, X. Carbon Spheres with Controllable Silver Nanoparticle Doping. J. Phys. Chem. C. 2010, 114 (2), 977–982. doi:10.1021/jp9102492.
- Chi, Y.; Yuan, Q.; Li, Y.; Tu, J.; Zhao, L.; Li, N.; Li, X. Synthesis of Fe3O4@SiO2–Ag Magnetic Nanocomposite Based on Small-Sized and Highly Dispersed Silver Nanoparticles for Catalytic Reduction of 4-Nitrophenol. J. Colloid Interface Sci. 2012, 383 (1), 96–102. doi:10.1016/j.jcis.2012.06.027.
- Wu, W.; Zhang, S.; Li, Y.; Li, J.; Liu, L.; Qin, Y.; Guo, Z.-X.; Dai, L.; Ye, C.; Zhu, D. PVK-Modified Single-Walled Carbon Nanotubes with Effective Photoinduced Electron Transfer. Macromolecules 2003, 36 (17), 6286–6288. doi:10.1021/acs.jpcc.6b09356.
- Gu, S.; Wunder, S.; Lu, Y.; Ballauff, M.; Fenger, R.; Rademann, K.; Jaquet, B.; Zaccone, A. Kinetic Analysis of the Catalytic Reduction of 4-Nitrophenol by Metallic Nanoparticles. J. Phys. Chem. C. 2014, 118 (32), 18618–18625. doi:10.1021/jp5060606.
- Wunder, S.; Polzer, F.; Lu, Y.; Mei, Y.; Ballauff, M. Kinetic Analysis of Catalytic Reduction of 4-Nitrophenol by Metallic Nanoparticles Immobilized in Spherical Polyelectrolyte Brushes. J. Phys. Chem. C. 2010, 114 (19), 8814–8820. doi:10.1021/jp101125j.