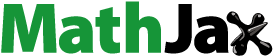
ABSTRACT
Cornstalk cellulose was liquefied in supercritical acetone at various temperature by a high-pressure autoclave, where the maximum yield of bio-oil was 43.79% and the highest conversion rate of cellulose was 72.13%. FT-TR spectrums showed that the C–O–C and C–C bonds in cellulose were cleaved under the attack of supercritical acetone, then active fragments were produced and transformed into liquefaction products. GC-MS results showed that the dominant components in bio-oil were ketones, esters, alkanes, etc. As the acetone dosage increased, ketones and glucosides increased while esters and alkanes decreased. A higher temperature was suitable for ketones and esters formation, which had an inhibition on alkanes formation. Finally, the formation of pathways and networks of dominant chemicals during cellulose liquefaction in supercritical acetone were developed. This investigation contributes to the knowledge of cellulose liquefaction in supercritical solvents for bio-oil and platform chemicals, which can provide an alternative method for biomass resources utility.
GRAPHICAL ABSTRACT
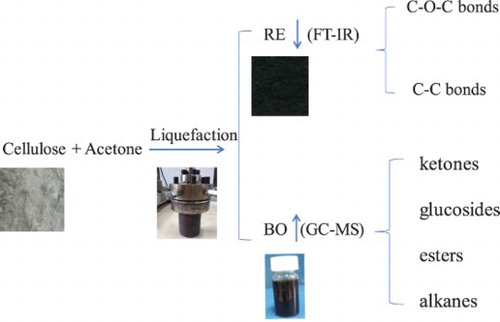
1. Introduction
Cellulosic biomass is the most widely distributed renewable resources on the earth (Citation1), it has gained much interest in producing clean biomass energy and green platform chemicals, such as biomass fuel and gas products (Citation2,Citation3). Thermo-chemical liquefaction has been employed as an effective method to convert biomass resources into valuable bio-oil products with various solvents (Citation4–6). Presently, various supercritical organic solvents (such as cyclohexane, water, methanol and ethanol) were extensively employed as thermo-chemical liquefaction media for biomass liquefaction (Citation7–10), supercritical liquefaction was proved to be more efficient in liquid products yield and feedstock conversion owing to the excellent advantages of heat and mass transfer (Citation11–13). For example, both the liquid product yield and cellulose conversion rate were higher when cellulose was liquefied in supercritical ethanol (Citation14–16), and the supercritical water also had a contribution on the cellulose depolymerization and conversion (Citation17–19). The reason was presumed that supercritical solvents can produce hydroxyl radicals, hydrogen radicals and alkyl radicals, then the C–O–C, C–C, C–H and –OH bonds were cleave under the attack of radicals which had a contribution on cellulose converting to liquid products (Citation8, Citation20,Citation21). Additionally, it was found that ketones, esters, alkanes and aromatics were in high content in the liquid product of cellulose liquefaction in supercritical solvents (Citation22–25), these green platform chemicals were widely used as important chemical materials. Therefore, supercritical liquefaction of biomass was also proved to be a promising method for producing platform chemicals.
On consider that acetone has a higher solubility to bio-oil components, and it has a relative lower supercritical point (supercritical temperature 235.5°C, supercritical pressure 4.72 MPa) in comparison with alcohols and other solvents. It has been found that more chemical materials can be obtained in supercritical acetone than in supercritical ethanol under the same reaction condition. Meanwhile, the solvent could affect the formation of various compounds in the bio-oil (Citation26,Citation27). To best of our knowledge, there have been few studies about the mechanism of cellulose liquefaction in supercritical acetone, and the selectivity of acetone on platform chemicals formation in bio-oil is also not clarified. In the present study, cornstalk cellulose liquefaction in supercritical acetone was carried out by a high-pressure autoclave under various reaction conditions. The FT-IR and GC-MS methods were employed in the liquefaction productions identification. The effects of acetone dosage, temperature, reaction time on cellulose liquefaction and the chemicals distribution in liquid product were investigated, the aim was to clarify the liquefaction mechanism and the chemicals transformation pathways. This study would provide meaningful knowledge about the selectivity of biomass liquefaction in supercritical solvents.
2. Experimental
2.1. Materials and reagents
The fresh cornstalk was cut into pieces and collected from the farm which located in South China Agricultural University, Guangzhou, China. The fresh cornstalk was pre-dried at 80°C for 12 h and milled to obtain the fine powder, and the powder that passed through a 40-mesh sieve was used in the experiments. The fine powder was dried at 105°C for 12 h to remove the moisture before use. Sodium chlorite was of industrial grade. Acetone, benzene, ethanol, sodium hydroxide and other solvents used were all of analytical grade.
2.2. Cellulose preparation
Water-soluble components in the cornstalk powder were removed according to the GB/T 2677.1-93 and GB/T 2677.10-95. Then, the powder was treated by the sodium chlorite solution to remove lignin and obtain holocellulose. An insoluble residue (cornstalk cellulose) was then prepared by treating the holocellulose by sodium hydroxide. The cornstalk cellulose was dried at 105°C for 12 h and kept in the desiccator at room temperature for experiments. According to GB/T 744-04 and GB/T 2677.3-93, the cellulose content and ash content of the cornstalk cellulose were 97.51 ± 1.04% and 1.23 ± 0.19%, respectively.
2.3. Experimental procedures
The dosage of powdered cornstalk cellulose was 8.0 g, the acetone dosage range was 0∼200 mL. The cellulose and acetone were loaded into the high-pressure autoclave (CJF-0.5, China). The liquefaction experiments were conducted in the autoclave at 240∼320°C for 0∼120 min. The heating rate of the high-pressure autoclave was 4∼6°C/min. The autoclave used was rated up to a working pressure of 30 MPa and a working temperature of 450°C. The liquefaction products were divided into Gas products (GAS), Bio-oil (BO), Cellulose residue (RE) and Volatile compounds (VO), which can be removed with the helping of the rotary evaporation apparatus.
The supercritical point of acetone are 235.5°C and 4.72 MPa, respectively. The experimental procedures are shown in . The volatile compounds (VO) was classified for mass balance with the feedstock. The experiment results obtained in this study were reported using the following parameters,
where is the yield of the bio-oil,
is the yield of the solid residue,
is the yield of the gas products,
is the yield of volatile compounds.
is the weight of the bio-oil,
is the weight of the cellulose residue,
is the cornstalk cellulose conversion rate,
is the weight of the gas products, and
is the weight of the cornstalk cellulose.
2.4. Chemical analysis
The BO product was sampled and analyzed with a gas chromatograph equipped with a mass selective detector (GC-MS, Finnigan Co, USA). Both the injector and detector were kept at 250°C, the velocity of the carrier gas (He) was 1.0 mL·min−1. An HP-1 column (30 mm × 0.25 mm) was also used. The oven program was 10 min isothermal at 40°C, followed by a heating rate of 10°C·min−1–120°C, where it was held for 1 min. The oven temperature then increased to 250°C at a heating rate of 5°C·min−1 and was held for 10 min. The injected volume of the sample was 0.5 μL. The mass range scanned was from 35 to 335 amu in electron-impact (70 eV) mode. The GC-MS data were acquired and processed using Chemstation software (Finnigan Co, USA). The compounds in the samples were identified by comparing the mass spectra results with those in NIST library data.
The RE was analyzed by a Fourier Transform Infrared Spectroscopy (FT-IR, Bruker EQUIVOX55, Germany) to investigate the various reaction parameters on the clave of functional group in cellulose.
The RE was sputtered with 150 Å thick layer of gold in Bio-Rad sputter apparatus. The images were taken by scanning electron microscope (SEM, Hitachi S-4800, Japan). Then, the structure of RE was observed by SEM.
3. Results and discussion
3.1. Effect of acetone dosage on cornstalk cellulose liquefaction
Cornstalk cellulose was liquefied in various dosages of supercritical acetone (0∼200 mL) at 280°C for 60 min, the pressure in the autoclave was increased to 6.0∼8.5 MPa. The temperature and pressure were rated up to supercritical state of acetone. The effects of acetone dosage on cellulose conversion rate and the yields of GAS, BO, VO and RE isolated from cellulose liquefaction are shown in .
Figure 2. The yield of cellulose liquefaction products with various dosages of acetone at 280°C for 60 min.
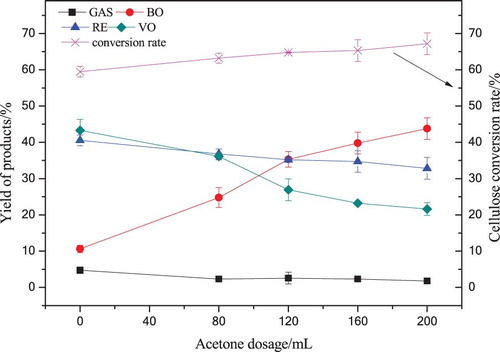
It can be seen from that BO yield strongly depended on the acetone dosage in the range of 80∼200 mL. With acetone dosage increased to 200 mL, the yield of BO increased from 10.63% to 43.79% fastly (almost four times that without acetone), the VO yield decreased from 43.29% to 21.59% rapidly, the yield of GAS (such as CO, CO2, H2, CH4, etc.) drop from 4.74% to 1.79%. Due to the C–O–C, C–C bonds in cellulose were clave under the attack of supercritical acetone molecular, on the other hand, more active fragments were trapped by supercritical acetone led to the formation pathways of VO and GAS were inhibited while a large number of small fragments were transformed to BO (Citation28,Citation29). With the depolymerization and liquefaction, the cellulose conversion rate increased from 59.46% to 67.17% gradually, therefore the RE yield decreased from 40.54% to 32.83%. The experimental results showed that supercritical acetone has significant effects on the distribution and transformation of the product during cellulose liquefaction, it has a promotion on BO yield and cellulose conversion rate while showed an inhibition on VO and GAS formation.
3.2. Effect of reaction temperature on cellulose liquefaction
Cornstalk cellulose was liquefied in 120 mL supercritical acetone under various reaction temperatures (240∼320°C) for 60 min, and the reaction pressure in the autoclave was 5.4∼8.9 MPa. The temperature and pressure were rated up to supercritical state of acetone. The effects of reaction temperature on the yields of GAS, BO, VO and RE from cellulose liquefaction are shown in .
Figure 3. The yield of cellulose liquefaction products with various dosages reaction temperatures in 120 mL acetone for 60 min.
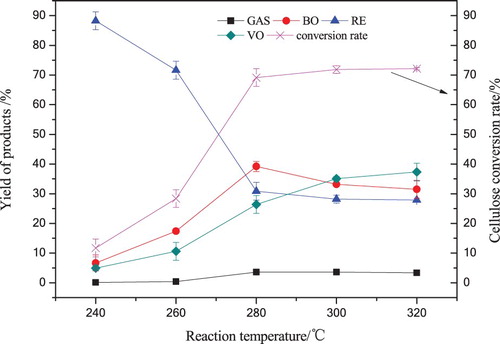
shows clearly that over the whole range of temperatures tested, cellulose conversion rate and VO yield increased continuously with the increasing temperature. With the temperature increased from 240°C to 280°C, the yield of BO reached the maximum 39.19%, as reaction temperature further reached to 320°C, BO yield decreased to 31.48% gradually. The reason for this behavior was that cellulose was decomposed to BO components in the initial liquefaction stage under the effect of supercritical acetone. However, the secondary reactions (such as decomposition, etc.) among BO components were favored when temperature was above 280°C (Citation30), which led to the formation of RE, VO and GAS. It can be presumed that 280°C would be an effective reaction temperature for the liquefaction cellulose for BO in supercritical acetone. As the temperature increased to 320°C, the yield of GAS increased by 3.46% and the VO yield improved considerably to 37.32%, which was due to the more lower molecular weight fragments were produced from cellulose liquefaction at a higher temperature (Citation31), then the more volatile compounds and gaseous products were produced via addition, decomposition, hydrogenation among these active fragments. From 240°C to 280°C, both cellulose conversion rate and BO yield improved sharper than that of 280∼320°C, the yield of RE dropped sharper at 240∼280°C than 280∼320°C. Experimental data indicated that 280°C was an appropriate liquefaction temperature to gain a maximum BO yield and a higher cellulose conversion rate, the conversion rate improved slowly over 280°C.
3.3. Effect of reaction time on cellulose liquefaction
Cornstalk cellulose was liquefied in 120 mL supercritical acetone under various reaction times (0∼120 min) at 280°C, the reaction pressure in the autoclave increased to 5.6∼7.4 MPa. The temperature and pressure were rated up to supercritical state of acetone. The effects of reaction time on the yields of GAS, BO, VO and RE obtained from cellulose liquefaction are shown in . The reaction time was valid once the temperature reached to the reaction temperature.
Figure 4. The yield of liquefaction products with various reaction times in 120 mL acetone at 280°C.
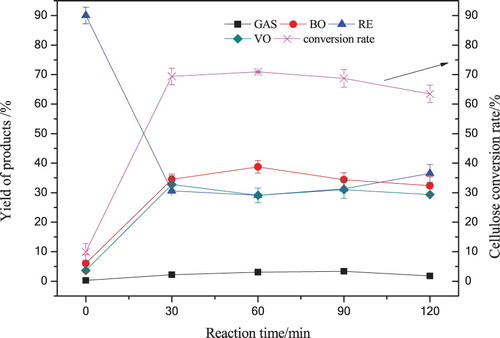
As shown in , when the reaction time prolonged from 0 to 30 min, the yield of BO, VO and conversion rate increased sharply to 34.48%, 32.75% and 69.40%, respectively. The reason was that cellulose was depolymerized and liquefied to active fragments to form liquid products and volatile compounds and gas products under the effects of supercritical acetone radicals at 280°C. The maximum conversion rate was observed to be 63.46% at 60 min, and BO yield improved less dramatically from 30 min to 60 min, however, the reaction time further prolonged to 120 min, the yield of BO and VO decreased gradually, it was presumed that the secondary reactions to form RE were favored as time prolonged, therefore the RE yield increased from 29.10% to 36.54%, and the conversion rate decreased by 7.44%. For example, the BO and VO yields drop as part of components transformed into RE through repolymerization, addition, decomposition, cyclization and redox. During the whole liquefaction process the yield of GAS was below 3.33%. The results indicated that 30 min was an effective time for cellulose liquefaction to produce a higher yield of BO and a better conversion rate in supercritical acetone.
3.4. FT-IR analysis for RE under various reaction conditions
The functional groups of residues produced at various reaction conditions during cornstalk cellulose liquefaction were characterized by FT-IR. The results are shown in . According to the literatures (Citation30–32), the absorption around 1060∼1160 cm−1 corresponds to the vibration of C–O–C bonds, the absorption at 1680∼1715 and 1710∼1770 cm−1 corresponds to C = O and –COOH groups, respectively. The absorption at 2900∼3100 cm−1 and 3300∼3600 cm−1 corresponds to –C–H and –OH groups, respectively.
Figure 5. The FT-IR analysis of RE under various reaction conditions and SEM pictures of RE. (a) FT-IR analysis for RE with various dosages of acetone at 280°C for 60 min. (b) SEM pictures of RE. (c) FT-IR analysis for RE with various reaction temperatures with 120 mL acetone for 60 min. (d) FT-IR analysis for RE with various reaction times with 120 mL acetone at 280°C.
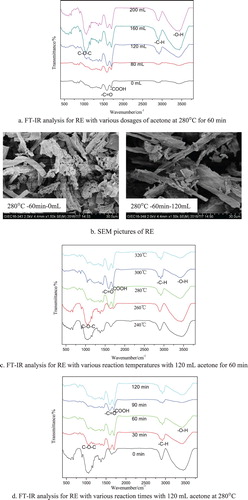
The RE was composed of cellulose and other solid products. (a) shows that with the increasing of acetone dosage, the absorption of –OH, C–O–C and –C–H groups became stronger gradually, which was due to cellulose was not liquefied completely at 280°C. A higher acetone dosage had an unsignificant promotion on cellulose conversion. The absorption of –C = O and –COOH groups became obvious owing to the formation of RE by secondary reactions (such as re-polymerization, condensation, coking, redox, etc.) among ketones, esters and other carbonyl compounds in BO. By comparing the results in (b), cornstalk cellulose was cleave more thoroughly and decomposed into smaller fragments with the effects of supercritical acetone.
It can be seen from (c) that over the whole range of temperatures tested, the absorption peak of –OH, C–O–C and –C–H groups became weaker as the temperature increased, which was due to a higher temperature had an enhancement on the cellulose de-polymerization and liquefaction for liquid products (Citation33), the –OH, C–O–C and –C–H bonds were cleave under the attack of supercritical acetone at a higher temperature. The maximum cellulose conversion rate was 72.13% at 320°C. However, the secondary reactions in BO were also enhanced which led to the absorption of –C = O and –COOH groups became stronger.
As shown from (d), the absorption of –OH, C–O–C and –C–H groups became weaker gradually as reaction time prolonged to 90 min, the reason was that cellulose was decomposed to liquefaction products under the effects of supercritical acetone as time prolonged. However, the absorption peak of –OH, C–O–C and –C–H groups were obviously observed again as time further prolonged to 120 min, due to the secondary reactions of hydroxyl compounds and other bio-oil components. With the reaction time prolonged, the secondary reactions among bio-oil chemicals were enhanced, resulted in the absorption peak of –C = O and –COOH groups obviously observed. A moderate reaction time was preferred to get a higher cellulose conversion rate.
3.5. Platform chemicals formation during cellulose liquefaction in supercritical acetone
3.5.1. GC-MS analysis for BO obtained from various acetone dosages
The bio-oil obtained from cellulose liquefaction in various dosages of acetone (0, 80, 120, 160 mL) at 280°C for 60 min were characterized by GC-MS to investigate the effect of acetone dosages on dominant chemicals formation. The platform chemicals were divided into ketones, esters, alkanes, alcohols, etc. The contents of dominant chemicals identified in/with the bio-oil are presented in .
Table 1. The GC-MS analysis for BO with various dosages of acetone at 280°C for 60 min.
shows that ketones (Carbon numbers C5∼C20), esters (Carbon numbers C7∼C30), alkanes (Carbon numbers C10∼C30) have a higher content in BO, and alcohols, acids, aldehydes and phenols were lower. Acetates and methyl esters were the dominant compounds of esters, this indicated that CH3· and CH3C = O· radicals were produced from supercritical acetone, the addition and condensation among these methyl and acetyl radicals and cellulose active fragments produced a lot of esters, on the other hand, the esterification between acids and alcohols also have a contribution on esters formation (Citation34). Ketones were composed of aliphatic ketones (4-Hydroxy-4-methyl-2-pentanone, etc.), alicyclic ketones (cyclopentanone, furanone, etc.) and aromatic ketones (cyclopentenone, etc.). In the initial stage, cellulose underwent dehydration, isomerization to form alicyclic ketones, aliphatic ketones were produced from active fragments via ring-cleavage, addition, aromatic ketones were formed from aliphatic ketones and alicyclic ketones underwent cyclization, aromatization and dehydration (Citation25). The carbon numbers of alkanes were between C10∼C30, such as eicosane and heneicosane. Alkanes were formed from cellulose via ring-cleavage, addition, dehydration, hydrogenation, deoxygenation, decarboxylation and decarbonylation (Citation35,Citation36).
3.5.2. Chemicals distribution in BO and the formation pathways
According to , the overall relative contents of ketones, esters, alkanes and alcohols in BO were calculated. The formation pathways of these platform chemicals were also developed. Platform chemicals distribution in BO under various acetone dosages, the results are shown in .
It can be seen from that supercritical acetone had a significant contribution on the formation and transformation of BO compounds. The content of ketones, esters and alkanes were 13.45%, 23.81% and 43.84% without acetone, respectively. With the increasing of acetone to 80 mL, the content of ketones reached 39.12%, owing to that the cellulose fragments were formed to a lot of ketones under the attack of supercritical acetone at 280°C. However, as the dosage of acetone further increased to 160 mL, ketones content drop to 18.08%, which was due to the formation of esters and alkanes under a higher concentration of acetone radicals. Over the whole range of acetone dosages tested, esters content was decreased from 23.81% to 11.67%, which was due to the decomposition of esters to alkanes, acids and alcohols. The content of alkanes decreased to 33.93% as acetone dosage increased to 200 mL, it may be that alkanes transform into other types of chemical compounds through the cyclization, condensation, addition and decomposition reaction. However, the content of glucosides was reached to 17.27% sharply while acetone dosage was over 120 mL, it was consumed that the further decomposition of glucosides from cellulose liquefaction was inhibited by a higher dosage of acetone, resulted in the decreased content of ketones, esters and alkanes. These platform chemicals are a promising choice to be used in chemical synthesis and cosmetics, etc. The results indicated that 80 mL is a preferred acetone dosage to pursue a higher content of ketones and esters.
The BO obtained from cellulose liquefaction in supercritical acetone under various reaction temperatures were analyzed by GC-MS. The overall relative contents of ketones, esters and alkanes in BO were calculated from the GC-MS results. The content of dominant platform chemicals is shown in .
Figure 7. The content of platform chemicals in BO under various reaction temperatures with 120 mL acetone for 60 min.
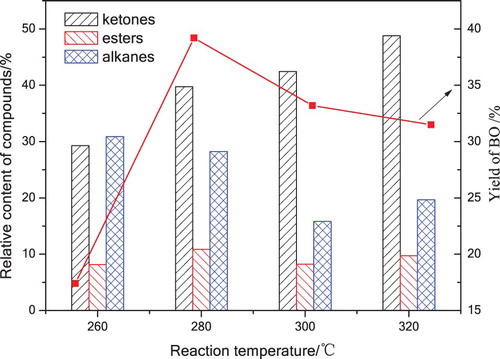
Ketones, esters and alkanes were the dominant chemicals in BO as shown in . The content of ketones increased from 29.29% to 48.74% dramatically as the reaction temperature increased to 320°C, which was due to cellulose was decomposed under the attack of supercritical acetone radicals and underwent dehydration, isomerization and addition to form ketones. The content of esters was at 8.13%∼10.84%, the esterification between acids and alcohols had a contribution on methyl esters formation, the acetyl (CH3–C = O·) produced from acetone had an obvious enhancement on the formation of acetates. The content of alkanes dropped from 30.91% to 15.84% with the increasing temperature, alkanes were formed from cellulose underwent ring-cleavage, addition, dehydration, hydrogenation, deoxygenation, decarboxylation and decarbonylation. Additionally, there were lower content of alcohols, acids, aldehydes and benzenes, phenols identified in BO. The GC-MS results indicated that a higher temperature was suitable for ketones and esters formation, which had an inhibition on alkanes formation. Experimental data proved that 320°C is a preferred reaction temperature to pursue a higher content of ketones and esters.
According to 3.5.1 and 3.5.2(above), the formation pathways and networks of dominant chemicals (ketones, esters, acids, alcohols, alkanes, aldehydes, glucosides, etc.) during cellulose liquefaction in supercritical acetone were developed (Citation21, Citation29, Citation34, Citation37,Citation38). It is shown in .
4. Conclusions
The influences of acetone dosage, temperature, reaction time on the cornstalk cellulose liquefaction in supercritical acetone, the FT-IR analysis for RE and GC-MS analysis of BO were investigated. A moderate reaction temperature, a short reaction time and a high acetone dosage were usually suitable for the production of BO (43.79%) and a higher conversion rate of cellulose (72.13%). Supercritical acetone had a significant enhancement on cellulose depolymerization and liquefaction. The maximum contents of ketones, esters, alkanes and glucosides in bio-oil were 48.74%, 23.81%, 43.84% and 17.27%, respectively. The content of ketones and glucosides in BO were improved with supercritical acetone. However, the content of esters and alkanes were decreased. Moreover, a higher temperature was suitable for ketones and esters formation, which had an inhibition on alkanes formation. Finally, the formation networks of dominant chemicals during cellulose liquefaction in supercritical acetone were developed. The synergistic effect between supercritical acetone, temperature and reaction time has a regulation on cellulose liquefaction and platform chemicals formation, which showed a difference with other supercritical solvents.
Disclosure statement
No potential conflict of interest was reported by the authors.
Notes on contributors
Wei Li is a graduate student at College of Food, South China Agricultural University, China. His research interests focus on biomass chemistry.
Xin-an Xie is currently a professor at College of Food, South China Agricultural University, China. His research interests focus on biomass conversion and simulation optimization research.
Jiao Sun is a graduate student at College of Food, South China Agricultural University, China. Her research interests focus on biomass utilization.
Di Fan is a graduate student at College of Food, South China Agricultural University, China. Her research interests focus on biomass conversion.
Xing Wei is a graduate student at College of Food, South China Agricultural University, China. His research interests focus on biomass chemistry.
Additional information
Funding
References
- Song, C.C.; Wang, G.; Hu, H.Q. Progress in Thermochemical Liquefaction of Biomass. Energiae Solaris Sinica 2004, 25 (2), 242–247.
- Machovina, B.; Feeley, K.J. Restoring Low-Input High-Diversity Grasslands as a Potential Global Resource for Biofuels. Sci. Total Environ. 2017, 609, 205.
- Simon, D.; Tyner, W.E.; Jacquet, F.; Vermerris, W. Economic Analysis of the Potential of Cellulosic Biomass Available in France From Agricultural Residue and Energy Crops. Bioenerg. Res. 2010, 3 (2), 183–193.
- Skouta, R. Selective Chemical Reactions in Supercritical Carbon Dioxide, Water, and Ionic Liquids. Green Chem. Lett. Rev. 2009, 2 (3), 121–156.
- Liu, H.M.; Li, M.F.; Yang, S.; Sun, R.C. Understanding the Mechanism of Cypress Liquefaction in hot-Compressed Water Through Characterization of Solid Residues. Energies 2013, 6 (3), 1590–1603.
- Li, R.; Xie, Y.; Yang, T.; Li, B.; Zhang, Y.; Kai, X. Characteristics of the Products of Hydrothermal Liquefaction Combined with Cellulosic bio-Ethanol Process. Energy 2016, 114, 862–867.
- Peng, J.; Chen, P.; Lou, H.; Zheng, X. Catalytic Upgrading of bio-oil by HZSM-5 in sub- and Super-Critical Ethanol. Bioresour. Technol. 2009, 100 (13), 3415–3418.
- de Caprariis, B.; De Filippis, P.; Petrullo, A. Hydrothermal Liquefaction of Biomass: Influence of Temperature and Biomass Composition on the bio-oil Production. Fuel 2017, 208, 618–625.
- Yang, T.; Wang, W.; Kai, X.; Li, B.; Sun, Y.; Li, R. Studies of Distribution Characteristics of Inorganic Elements During the Liquefaction Process of Cornstalk. Energy Fuels 2016, 30 (5), 4009–4016.
- Phusunti, N.; Phetwarotai, W.; Tirapanampai, C.; Tekasakul, S. Subcritical Water Hydrolysis of Microalgal Biomass for Protein and Pyrolytic Bio-oil Recovery. Bioenerg Res. 2017, 10 (4), 1005–1017.
- Jun, Y.; Eiji, M.; Shiro, S. Liquefaction of Beech Wood in Various Supercritical Alcohols. Wood Sci. 2006, 52 (6), 527–532.
- Mazaheri, H.; Keatteong, L.; Bhatia, S.; Mohamed, A.R. Sub/Supercritical Liquefaction of oil Palm Fruit Press Fiber for the Production of bio-oil: Effect of Solvents. Bioresour. Technol. 2010, 101 (19), 7641–7647.
- Tao, H.X.; Xie, X.A.; Zheng, C.Y.; Zhan, X.Q. Liquefaction of Cornstalk Cellulose in sub/Super-Critical Ethanol. J. Northwest Univ. A&F 2014, 42 (1), 196–204.
- Li, H.; Yuan, X.; Zeng, G.; Huang, D.; Huang, H.; Tong, J.; You, Q.; Zhang, J.; Zhou, M. The Formation of bio-oil From Sludge by Deoxy-Liquefaction in Supercritical Ethanol. Bioresour. Technol. 2010, 101 (8), 2860–2866.
- Zheng, C.Y.; Xie, X.A.; Tao, H.X.; Zheng, L.S.; Li, Y. Depolymerization of Stalk Cellulose During its Liquefaction in sub-and Supercritical Ethanol. J. Fuel Chem. Technol. 2012, 40 (5), 526–532.
- Xu, F.; Geng, X.C.; Liu, C.F.; Ren, J.L.; Sun, J.X.; Sun, R.C. Structural Characterization of Residual Lignins Isolated with Cyanamide-Activated Hydrogen Peroxide From Various Organosolvs Pretreated Wheat Straw. J. Appl. Polym. Sci. 2008, 109 (1), 555–564.
- H.T. Cao. The Research on the Liquefaction of Biomass in Sub-and Supercritical Water. Master’s Thesis, Hunan University, Changsha, China. 2008.
- Yuan, X.; Cao, H.; Li, H.; Zeng, G.; Tong, J.; Wang, L. Quantitative and Qualitative Analysis of Products Formed During Co-Liquefaction of Biomass and Synthetic Polymer Mixtures in sub- and Supercritical Water. Fuel Process. Technol. 2009, 90 (3), 428–434.
- Xiao, X.; Bian, J.; Peng, X.P.; Xu, H.; Xiao, B.; Sun, R.C. Autohydrolysis of Bamboo (Dendrocalamus Giganteus Munro) Culm for the Production of Xylooligosaccharides. Bioresour. Technol. 2013, 138, 63–70.
- X.F. Chen. Analysis of the Products from Alkanolysis of the Rice-Stalk Powder and Related Mechanism Study, Master’s Thesis, Wuhan University of Science and Technology, Wuhan, China, 2008.
- Li, W.; Xie, X.A.; Tang, C.Z.; Li, Y.; Li, L.; Wang, Y.L.; Wei, X.; Fan, D. Effects of Hydroxyl and Hydrogen Free Radicals on the Liquefaction of Cellulose in sub/Supercritical Ethanol. J. Fuel Chem. Technol. 2016a, 44, 415–421.
- Chang, S.; Zhao, Z.L.; Zhang, W.; Zheng, A.Q.; Wu, W.Q.; Li, H.B. Comparison of Chemical Composition and Structure of Different Kinds of Bio-Oils. J. Fuel Chem. Technol. 2011, 39 (10), 746–753.
- Demirbas, A. The Influence of Temperature on the Yields of Compounds Existing in bio-Oils Obtained From Biomass Samples via Pyrolysis. Fuel Process. Technol. 2007, 88 (6), 591–597.
- Li, W.; Xie, X.; Tang, C.; Li, Y.; Li, L.; Wang, Y.; Fan, D.; Wei, X. The Distribution of bio-oil Components with the Effects of sub/Supercritical Ethanol and Free Radicals During Cellulose Liquefaction. BioResour. 2016, 11 (4), 9771–9778.
- Zheng, C.Y.; Tao, H.X.; Xie, X.A. Distribution and Characterizations of Liquefaction of Celluloses in sub- and Super-Critical Ethanol. Bioresour. 2013, 8 (1), 648–662.
- Durak, H.; Aysu, T. Thermochemical Liquefaction of Algae for bio-oil Production in Supercritical Acetone/Ethanol/Isopropanol. J. Supercrit. Fluids 2016, 111, 179–198.
- Liu, H.-M.; Liu, Y. Effect of Different Solvents on Cypress Liquefaction to Fuels and Characterization of Produces. Bioresour. Technol. 2013, 8 (4), 6211–6219.
- Shao, Q.J.; Peng, J.X.; Xiu, S.D.; Wen, X.H. Analysis of oil Products by Pyrolysis of Bamboo in Supercritical Methanol. Acta Energ. Sol. Sin. 2007, 28 (9), 984–987.
- Tao, H.X.; Xie, X.A.; Tang, C.Z.; Tian, W.G. Mechanism of Ketones Formation From Cellulose Liquefaction in sub- and Supercritical Ethanol. J. Fuel Chem. Technol. 2013, 41 (1), 60–66.
- R, L.M.I.; Barros, B.D. Aggregation of Pseudoisocyanine Iodide in Cellulose Acetate Films: Structural Characterization by FTIR. Langmuir 2000, 16 (24), 9331–9337.
- Kacurakova, M.; Capek, P.; Sasinkova, V.; Wellner, N.; Ebringerova, A. FT-IR Study of Plant Cell Wall Model Compounds: Pectic Polysaccharides and Hemicelluloses. Carbohydr. Polym. 2000, 43 (2), 195–203.
- Li, M.F.; Sun, S.N.; Xu, F.; Sun, R.C. Formic Acid Based Organosolv Pulping of Bamboo (Phyllostachys Acuta): Comparative Characterization of the Dissolved Lignins with Milled Wood Lignin. Chem. Eng. J. 2012, 179 (4), 80–89.
- Soares, S.; Ricardo, N.M.P.S.; Jones, S.; Heatley, F. High Temperature Thermal Degradation of Cellulose in air Studied Using Ftir and 1H and 13C Solid-State NMR. Eur. Polym. J. 2011, 37 (4), 737–745.
- Tang, C.Z.; Tao, H.X.; Zhan, X.Q.; Xie, X.A. Mechanism of Esters Formation During Cellulose Liquefaction in Sub- and Supercritical Ethanol. Bioresour. 2014, 9, 4946–4957.
- Guo, G.Q.; Wang, H.J.; Chen, F.G. Thermochemical Liquefaction of Lignocellulosic Materious in Hydrogen-Donor Solvent. J. Cellulose Sci. Technol. 2003, 11 (2), 41–50.
- Mortensen, P.M.; Grunwaldt, J.D.; Jensen, P.A.; Knudsen, K.G.; Jensen, A.D. A Review of Catalytic Upgrading of bio-oil to Engine Fuels. Appl. Catal. A-Gen 2011, 407 (1), 1–19.
- J. B. Huang. Molecular Simulation Study of Pyrolysis Mechanism of Cellulose. Doctor’s Thesis, Chongqing University. Chongqing. China, 2010.
- Z.F. Geng. Theoretical and Experimental Study on Mechanism of Cellulose Pyrolysis, Doctor’s Thesis. Tianjin University, Tianjin, China, 2010.