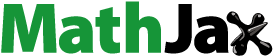
ABSTRACT
Green synthesis of nanoparticles is an emerging field of nanotechnology, preferred over physical and chemical synthesis owing to their safety, cost-effectiveness nature, bio-compatibility, eco-friendly and scalable properties. The present study includes Seripheidium quettense aqueous extract mediated green synthesis, optimization of silver nanoparticles (Sq-AgNPs) and their pharmacological evaluations. Synthesis was done considering various optimization parameters including concentrations of AgNO3, pH of the reaction mixture, extract to precursor ratio and temperature. Biosynthesis was carried out using our already optimized conditions. UV–visible spectrophotometer, FTIR, XRD and SEM analysis were used for characterization of Sq-AgNPs. The synthesized Sq-AgNPs (49.96–54.36 nm) were evaluated for their antibacterial, antifungal, anticancer and hemolytic potentials. The maximum antibacterial activity was found against Escherichia coli, Klebsiella pneumonia and Bacillus subtilis with their MICs of 11.1, 33.3 and 33.3 μg/mL, respectively. Aspergillus nigar was found as the most susceptible fungal strain with the highest zone of inhibition (13.2 ± 0.72 mm). Sq-AgNPs inhibited proliferation of human liver cancer cell lines (HepG2) with median lethal concentration (IC50s) of 62.5 µg/mL. Results of the hemolytic assay showed that SqNPs are bio-compatible and have less effect on erythrocytes even at high concentration of 100 µg/mL.
GRAPHICAL ABSTRACT
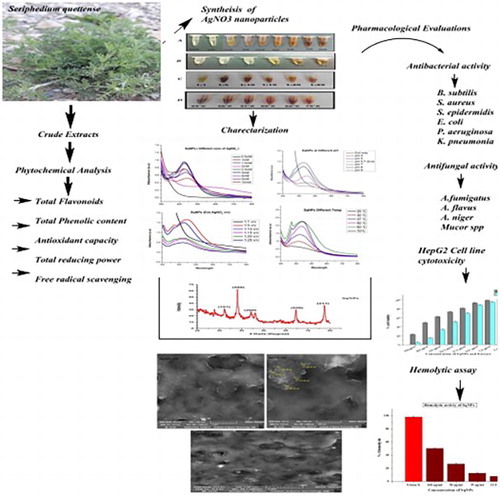
1. Introduction
Nanotechnology is an emerging arena with extensive impending medicinal potentials and applications. Due to their distinctive surface chemistry and morphological aspects, it has been exploited in many fields of science such as electronics, medicine, nutrition, designing, optics and microscopy (Citation 1, Citation 2) Among the metallic nanoparticles, AgNPs have got substantial focus due to their better stability, exceptional catalytic potentials and better electrical conductivity (Citation 3). AgNPs have wide applications from drug and gene delivery for sensing of nucleic acids and pathogens (Citation 4). Moreover, AgNPs can be used as an alternative therapeutic remedy against microbes, tumors and other infectious diseases (Citation 5). Several physical and chemical methods are reported to synthesize these AgNPs (Citation 6). Using these approaches, the precursor silver nitrate salt is reduced via reducing agents such as borohydride, citric acid or other hydrocarbons (Citation 7). But these approaches are expensive and are associated with safety issues. Subsequently, focus has been directed to the formulation of more safe methods for the synthesis of metallic nanoparticles.
The green synthesis approach is rapid, scalable, eco-friendly and inexpensive approach (Citation 5). Moreover, stable and biocompatible nano particles (NPs) can be produced using the green approach (Citation 2). As particle shape and size are very crucial in many bio-medical applications, the biogenic synthesis provides well control over particle size and morphology as compared to chemical or physical methods of NP synthesis. This method utilizes various plants, Algae, Fungi or microbial metabolites for the capping, reduction, and stabilization of respective NPs (Citation 8). In comparison to microbes, plants provides many advantages (Citation 6). Despite their multiple advantages, the biogenic synthesis has few limitations which can be controlled via optimization of extract-metal salt solution concentrations, pH and temperature of reaction mixture (Citation 9).
Seriphidium quettense, family Asteraceae, is traditionally used as vermifuge to relieve fever, diabetes and joints pain. Seriphidium herba-alba are used for treatment of diarrhea and abdominal cramps (Citation 10). Methanol extract of S. quettense (aerial parts) shows antimicrobial potentials against S. aureus, K. pneumoniae and P. aeruginosa (Citation 11). To best of our knowledge, no studies are reported on the S. quettense aqueous extract mediated green synthesis of Sq-AgNPs, their optimization and their biological evaluation. In this study, we have explored the potential application of Sq-AgNPs against microbes and cancer cells with the purpose of finding their applications in field of theranostics.
2. Materials and methods
2.1. Plant collection and extract preparation
Fresh aerial parts of S. quettense were collected in July 2016 from kawas village of District Ziarat, Baluchistan, Pakistan. Plant was identified by Professor Dr. Zabta Khan Shinwari, at Department of Plant sciences, Quaid-i-Azam University, Islamabad. A sample was deposited at herbarium, Department of Biotechnology, Quaid-e-Azam University Islamabad, Pakistan with voucher no: 350. The plant was shade dried for one week and crushed to powder (Citation 12).
2.2. Extract preparation
Plant powder (10 g) was taken in 200 ml of distilled water, boiled for 10 min and double filtered by using Whatman filter paper No.1. The extract was stored at 4°C in the refrigerator and further used for phytochemical analysis and synthesis of Sq-AgNPs (Citation 13).
3. Quantitative phytochemical analysis
Total reducing power of extract was estimated using potassium ferricyanide colorimetric assay (Citation 14). Estimation of Total Phenolic Content (TPC), DPPH free radical scavenging potentials and Total Antioxidant Capacity (TAC) (Phosphomolybdenum method) were evaluated according to already reported procedures (Citation 15). Total Flavonoid contents (TFC) was estimated as we reported previously (Citation 16).
4. Optimization and biosynthesis of SqNPs
To optimize AgNO3 concentration, SqNPs synthesis was checked at increasing concentrations of 0.5, 1, 1.5, 2, 2.5,3, 4, 5 and 10 mM. Briefly, 100 µL of S. quettense extract was added to 1000 µL of each concentration of AgNO3 solution in separate Eppendorf tubes. For pH optimization, same procedure was followed but using plant extract at increasing pH of 4, 5, 5.7 8 and 9. Ratio based optimizations were done according to the previously reported protocol (Citation 17). Different ratios (Ext: AgNO3) of (1:1, 1:5, 1:10, 1:15, 1:20 and 1:25 v/v) were used. For temperature optimization, synthesis was done at increasing temperatures of 25°C, 30°C, 37°C, 50°C, 60°C and 70°C. In order to check the stability of synthesized Sq-AgNPs, UV absorbance was monitored for the duration of two months. The stability of Sq-AgNPs was checked at 15, 30 and 60 days post synthesis.
5. Characterization of Sq-AgNPs
UV-3000 double beam spectrophotometer was used to check the formation of Sq-AgNPs. The UV-spectra was obtained by scanning the samples in the range between 200 and 800 nm. The functional characterization of biomolecules present in the Sq-AgNPs was performed by FTIR spectrometry. The characteristic infrared (IR) absorption spectra of the different functional groups were noted in the frequency range of 4000–5000 cm−1. Crystallographic structures of Sq-AgNPs were determined using X-ray diffraction (XRD) analysis. The morphological analysis of Sq-AgNPs was done via high-resolution SEM analysis (MIRA3 TESCAN).
6. Biological activities
6.1. Antibacterial assay
Antibacterial potentials of Sq-AgNPs were evaluated following standard well diffusion method previously reported (Citation 18, Citation 19). The number of colony forming units (CFU) was determined by adjusting the turbidity of each bacterial inoculums to 0.5 McFarland standard solution (Citation 20). Fresh inoculum of each strain (100 μL) was swabbed onto previously sterilized tryptic soy agar plates and wells of 6 mm diameter were formed using sterile cork borer. A volume of 30 μL of Sq-AgNPs solution (100 μg/ml DMSO) was subsequently added to wells under laminar flow hood. Cefixime and DMSO were used Positive and negative control, respectively. The plates were incubated for 24 h at 37°C and the zone of inhibition was measured. Those bacterial strains having zone of inhibition of ≥10 mm were evaluated at lower concentration (1.1 μg/mL to 100 μg/mL) of Sq-AgNPs for determination of Minimum Inhibitory Concentration (MICs) using three-fold micro broth dilution method (Citation 21). Bacterial suspension of 50 μL of each strain was added to a sterile 96-well microplate containing increasing concentrations (1.11–100 μg/ml) of Sq-AgNPs. Bacterial suspension without Sq-AgNPs was used as control. The plates were incubated for 24 h at 37°C and were analyzed visually to evaluate the growth of bacteria. The concentration of Sq-AgNPs at which no viable bacterial growth was observed was MIC value.
6.2. Antifungal assay
Antifungal activity of Sq-AgNPs was evaluated for four pathogenic fungal stains following previously reported protocol (Citation 22). The fungal strains were grown on previously sterilized SDA (Sabouraud Dextrose agar) media. The spores of each fungal strain were suspended in Tween 20 (0.02%) solution and their turbidity was set according to standard (0.5) McFarland solution. SDA plates were prepared and swabbed with 100 μL of harvested spores. Sterile discs impregnated with 100 μg/disc of Sq-AgNPs were placed equidistantly on seeded SDA plates. Antifungal drug amphotericin B (250 µg/ml) and DMSO were used as positive and negative control, respectively. Plates were incubated at 28°C and growth inhibition zones were measured after 24–48 h with the help of vernier caliper. All experiments were performed in triplicates.
6.3. HepG2 cytotoxicity studies
6.3.1. Cell culture
Cytotoxic potential of Sq-AgNPs against HepG2 live cancer cells was evaluated using MTT assay (Citation 23). Cells were cultured in DMEM (pH = 7.2) containing 10% FBS (Fetal Bovine Serum) in a humidified atmosphere of CO2 (5%) at 37°C temperature. Cells were added to 96-wells plates (1 × 104 in 100 µl of medium/well) and allowed to settle for 24 h. A volume of 100 µL of Sq-AgNPs suspension and extract solution of different target concentrations (7.8, 15.62, 31.25, 62.5 125, 250 and 500 µg/ml), were added to each well and left for 24 h. After incubation, 10 μL of sterilized 1 mg/mL MTT solution in distilled H2O was added and the plate was incubated again for 4 h in humidified CO2 (5%) incubator. Thereafter, DMSO (100 µl) was added to each well and mixed with the cells systematically for the thorough suspension of formazan crystals. Cells without Sq-AgNPs and extract were used as a negative control. The absorbance was measured at 570 nm using a microplate ELISA spectrophotometer reader. The cell sustainability was articulated as a percentage of the sustainability of the control cells.
6.4. Hemolytic assay
Hemolytic assay was performed to check the effect of aqueous extract of S. quettense and synthesized Sq-AgNPs. One micro liter (1 ml) of fresh human blood was taken in 1.5 ml Eppendorf and centrifuged at 14,000 rpm to obtain erythrocytes (RBCs). After centrifugation, the supernatant was discarded and 200 µL from pellet (blood) was taken in a falcon tube. Subsequently, 9.8 ml of phosphate buffer saline (PBS) was added to it and centrifuged (10 min/2000 rpm) for washing. After centrifugation, the supernatant was castoff. This washing step was repeated three times. A volume of 100 µL of red blood cell suspension in PBS was placed in 96 well plate and different concentrations of Sq-AgNPs dissolved in D-water were added to each well. The plates were then incubated (1 h/35°C). Triton X-100 (0.5%) was used as a positive control, while PBS and water were used as a negative control. Percentage of hemolysis was estimated using the following formula;
7. Data analysis
All the experiments were performed in triplicate. The data were presented as mean ± standard deviation (SD). The data was graphically presented using Origin Pro 8 and GraphPad Prism software’s.
8. Results and discussion
8.1. Phytochemical analysis and antioxidant capacity
The preliminary phytochemical screening of aqueous extract S. quettense aerial parts confirmed the presence of phenols and Flavonoids. In crude extracts, TFC of 56 ± 1.02 µg/mg extract and TPC of 40 ± 0.78 µg/mg extract were observed (). Flavonoids and phenolic compounds are known to have different biological properties, such as scavenging of free radicals and inhibition of lipid peroxidation. Certain flavonoids such as quercitin and luteolin are involved as bio-reductants during the synthesis of metal nanoparticles (Citation 24). The observed total antioxidant activity (TAA) and reducing power were 10.71 ± 0.65, 73.4 ± 0.11 µg/mg extract, respectively, whereas free radical scavenging activity was 30.6 ± 0.14%.
Table 1. Phytochemical analysis of Seriphedium quettense extract.
8.2. Optimization of Sq-AgNPs
Optimization is very cardinal step during the synthesis of metallic NPs (Citation 25). Optimization of various parameters such as concentration of the plant extract and silver salt solutions, pH of the reaction mixture, temperature at which the reaction is carried out and incubation period for the reaction can greatly affect the quantity, size, shape and biomedical applications of synthesized Sq-AgNPs (Citation 26, Citation 27).
8.3. Characterization of SqNPs
8.4. AgNO3 concentration-based optimization
Synthesis was performed at increasing concentrations of 0.5, 1, 1.5, 2, 2.5, 3, 4, 5 and 10 mM of AgNO3 solution. Color change was observed which confirms the synthesis of Sq-AgNPs as shown in (A). UV–visible spectrum of Sq-AgNPs is shown in . Maximum absorbance was recorded at 428 nm for 4 mM of AgNO3.
Figure 1. Color change of reaction mixture of synthesized Sq-AgNPs. (A). Color change of Sq-AgNPs at different concentration of AgNO3. (B). color change of Sq-AgNPs at different at pH, (C). Color change of Sq-AgNPs at different ratio of plant extract and AgNO3 solution, (D). Color change of Sq-AgNPs synthesized at variable temperatures.
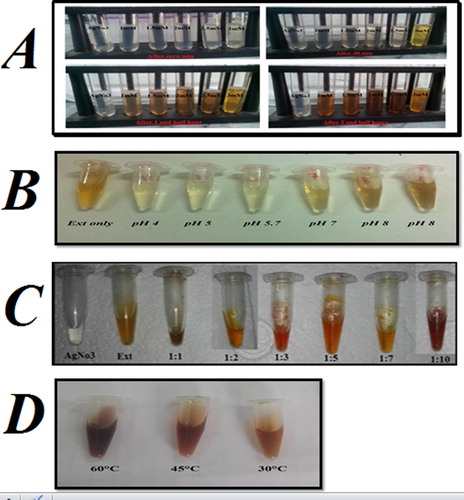
8.5. pH optimization of Sq-AgNPs
shows the synthesis of Sq-AgNPs at different pH of the extract. The color of the reaction mixture became yellowish dark as the pH increased (B). The extract reduced solution from colorless to light yellow due to the formation of Sq-AgNPs. The UV spectra show that maximum surface plasmonance (430 nm) was observed at alkaline pH (pH 8). It was observed that as the pH was increased the rate of Sq-AgNPs formation was also increased up to pH = 8 and then decreased. It might be owed to the ionization of phenolic composites in the extract of S. quettense (Citation 28). According to literature, acidic pH results in the formation of larger size particles while alkaline pH favors the formation of smaller size particles (Citation 29). High Sq-AgNPs formation was observed at basic pH than acidic pH. Our results are in agreement with previously published data (Citation 30), that basic pH results in the synthesis of stable NPs whereas at acid pH, NPs tend to aggregate.
8.6. Ext to AgNO3 ratio optimization
Like pH, the ratio of plant extract and AgNO3 also affect the size and morphology of synthesized NPs. Different ratios (Ext: AgNO3) of 1:1, 1:5, 1:10, 1:15, 1:20 and 1:25 v/v were used to determine the optimized ratio of extract and AgNO3. The color change was observed for each ratio (C). Maximum absorbance was recorded at 448 nm for 1:5 v/v as shown in . The absorbance shows that the quantity of Sq-AgNPs formed due to the reduction of silver ions.
8.7. Temperature-based optimization of Sq-AgNPs
Temperature plays a significant role in the production of Sq-AgNPs (Citation 28). NPs were synthesized at variable temperatures of 25°C, 30°C, 37°C, 50°C, 60°C and 70°C. D shows the color change of the reaction mixture. The highest intensity peak (439) was observed at 60°C as shown in . The absorbance increased with increasing temperature (Citation 26). It shows that the rate of NPs synthesis at room temperature can be boosted by increasing the temperature of the particular mixture. While on the other hand the particles tend to be poly-dispersed at high temperature.
8.8. Stability of Sq-AgNPs
The stability of Sq-AgNPs was checked at 15, 30 and 60 days of synthesis as shown in . It was observed that there are no vibrational changes at the surface plasmon resonance of the NPs. The formation of monodisperse nanoparticles was shown by the appearance of sharp peak. In a study, AgNPs synthesized from Cassia roxburghii aqueous extract also showed stability for the duration of two months (Citation 31).
8.9. Fourier transform infrared Spectroscopy (FTIR)
Bio-molecules involved in the capping and reducing of Sq-AgNPs were analyzed by FTIR . FTIR study of Sq-AgNPs showed absorption peaks located at 3409, 3340, 2915, 2870, 2365, 1611, 1315, 1200, 1070 and 1020 which characterizes several functional groups like amine N–H stretching, alcoholic O–H stretching, C O of carboxylic anions, –C–H stretching, C≡N, nitriles, of –C=C– designated to the amide 1 bonds of proteins, amine groups, (C–O), (eOCH) stretching and (C–N) receptivity.
8.10. X-rays diffraction
XRD spectra of Sq-AgNPs are given as . XRD pattern of Sq-AgNPs shows the five main peaks at 2θ values of 32.29, 38.15, 44.55, 64.61, 77.67 corresponding to 101, 200, 200, 220 and 311 planes for silver indicated that these NPs were crystalline in nature. Scherrer’s equation was used to calculate the mean particle size of silver nanoparticles (Sq-AgNPs); (D = Kλ/β cosθ),
where D represents the size of Sq-AgNPs, λ represents the wavelength of the X-ray source (1.540) used in XRD, β is the FWHM (full width at half maximum of the diffraction peak), K is the Scherrer constant (values ranges from 0.9 to 1), and θ is the Bragg angle. It was found that the average grain/crystallite size of Sq-AgNPs from XRD data is 29.16 nm.
8.11. Scanning electron microscopy (SEM) analysis
The size and surface morphology of the synthesized Sq-AgNPs was characterized by SEM analysis. shows the SEM results of synthesized Sq-AgNPs. The micrograph shows that particles are of spherical shape and having various sizes ranging from 48.40 to 55.35 nm. Most of the NPs were aggregated in the form of clusters, and only few of them were scattered, as observed under SEM. The average size was found to be of 53.3 nm.
9. Biological assays
9.1. Antibacterial potentials of Sq-AgNPs
Antibiotics resistance is becoming a global issue and search for effective antibacterial drugs is the need of current time (Citation 32, Citation 33). The antibacterial activity of synthesized Sq-AgNPs was studied against Gram-positive and Gram-negative pathogenic bacterial strains. Sq-AgNPs were most active against E. coli and B. subtilis with MICs of 11.1 and 33.3 μg/mL, respectively. Sq-AgNPs were moderately active against S. epidermidis, P. aeruginosa and was inactive against S. aureus ( ). According to previous reports, AgNPs synthesized from Chrysanthemum indicum flowers showed good activity against E. coli and K. pneumoniae (Citation 34). The exact bactericidal mechanism of AgNPs is not very well understood yet. Some researchers believe that AgNPs might interfere with bacterial cell wall synthesis, inhibit the synthesis of vital proteins implicated in cell division, inhibit the synthesis of nucleic acids and interfere metabolic pathways (Citation 35). It is also reported that AgNPs induce plasmolysis and disturb the permeability of cell membrane which effect the respiration process (Citation 36, Citation 37).
Table 2. Antibacterial and antifungal activities of synthesis Sq-AgNPs from aqueous extract of Seriphedium quettense plant.
9.2. Antifungal potentials of Sq-AgNPs
Due to the emergence of resistant fungal infections, researchers are looking for more effective antifungal drugs from alternative sources. The antifungal potentials of green synthesized Sq-AgNPs were evaluated against four pathogenic fungal strains. High antifungal activity was observed against A. niger and A. fumigatus with inhibitory zones of 13.2 ± 0.72 and 12 ± 0.33 mm, respectively (). AgNPs synthesized via various plants extracts are reported to have considerable antibacterial and antifungal potentials (Citation 26, Citation 38).
9.3. HepG2 cytotoxicity studies
In HepG2 liver cancer cell lines cytotoxicity assay, percent cell viability declined with an increase in the concentration of Sq-AgNPs (). Median Inhibitory concentrations (IC50) were 62.5, 251 µg/ml for Sq-AgNPs and extract, respectively. Sq-AgNPs were comparatively more active against HepG2 cells than crude extract. Previous studies also reported that AgNPs possess more cytotoxicity than plant extract (Citation 27). AgNPs synthesized using leaf extract of Andrographis echioides exhibited IC50 value of 31.5 µg/mL against MCF-7 cells (Citation 39). The proposed mechanism of AgNPs-mediated cancerous cells toxicity is exhibited via induction of apoptosis (Citation 40, Citation 41), generation of reactive oxygen species (ROS) leading too DNA damage and cell death (Citation 42, Citation 43).
9.4. Hemolytic activity of Sq-AgNPs
The toxicity of synthesized Sq-AgNPs was evaluated against freshly isolated human RBC’s. shows the percent hemolysis at increasing concentrations of Sq-AgNPs. Percent hemolysis of RBCs was observed to be dependent of concentration of Sq-AgNPs. The observed hemolysis at concentration of 12.5 and100 μg/mL were 3.6 and 27.3%, respectively. The hemolytic potentials of NPs are highly dependent on its size and size of 0.2 μm can enter RBCs causing its rupturing. Moreover, it is reported that NPs may bind to the thiol groups of the proteins or phospholipids of membrane cause their denaturation (Citation 44, Citation 45).
10. Conclusions
Green synthesis of silver nanoparticles using plant extract is inexpensive, eco-friendly and cost effective approach. Silver nanoparticles were synthesized via an aqueous extract of S. quettense extract. Different optimization parameters like concentrating of AgNO3 salt, extract pH, ratio of AgNO3 and plant extract salt and temperature were optimized to synthesize AgNPs with desire size, shape and biological properties. The Sq-AgNPs (49.96–54.36 nm) were characterized by UV–visible spectrophotometer, FTIR, XRD and SEM. The Sq-AgNPs were found effective against E. coli, K. pneumonia a, B. subtilis and A. nigar. Sq-AgNPs showed considerable cytotoxic effects against HepG2 cells with IC50 of 62.5 µg/mL. Further, Sq-AgNPs were bio-compatible with less cytotoxicity against RBCs. Further in-vivo studies on the Sq-AgNPs are recommended for more convincing results.
Acknowledgements
We are grateful for the Department of Biotechnology, Quaid-i-Azam University Islamabad for their Lab facilities to conduct this study. We are also thankful to Institute of space technology Islamabad and National center for physics for their help in characterization of nanoparticles.
Disclosure statement
No potential conflict of interest was reported by the authors.
Notes on contributors
Muhammad Qasim Nasar received BS-Biotechnology degree from Forman Christian College Lahore. He is Master of Philosophy student of Biotechnology at Quaid-i-Azam University Islamabad Pakistan. His main area of Research is green nano-biotechnology and evaluation of Bio-active phytochemicals from medicinal plant for biological activities.
Tanzeel Zohra received her MSc Botany from Arid Agriculture University Rawalpindi Pakistan. She has done her M. Phil Biotechnology from Quaid-i-Azam University. Her M.Phil research was about pharmacological evaluation of medicinal plants.
Ali Talha Khalil received his PhD Biotechnology degree from Quaid-i-Azam University Islamabad Pakistan. His research area includes green synthesis of metal nanoparticles and evaluation of their biological potential. He is fellow of UNESCO-UNISA Africa Chair in Nanosciences and Nanotechnology and fellow of NANOAFNET.
Sadam Saqib received his M.Phil degree in Plant Sciences from Quaid-i-Azam University. His research area includes synthesis of nanoparticles and evaluation of their effect against plant pathogens.
Muhammad Ayaz got his Doctor of Pharmacy (Phrm-D) degree from University of Malakand with a gold medal. Thereafter, he completed his M.PhiL degree from University of Peshawar in Pharmaceutical Sciences (Pharmacology) and PhD from University of Malakand in Pharmaceutical Sciences (Pharmacology). His research area is ethnopharmacology, Alzheimer's disease, Phytochemistry and nanotechnology. He is Associate Editor for Frontiers in Pharmacology (Ethnopharmacology), BMC Complementary and Alternative Medicine, International Journal of Pharmacology, Pharmacologia, Trends in Medical Research and Digital Chinese Medicine. He has Published more than 50 research and review articles with impact factor of 120+ and 700 Plus citations.
Ashfaq Ahmad is currently an assistant Professor at Pharmacy Department Sarhad University of Science and Information Technology (SUIT). He got his M.PhiL degree from Kohat University of Science and Technology (KUST)and PhD from University of Malakand. He has published in renowned journals of high impact factors.
Zabta Khan Shinwari is professor of Biotechnology at Quaid-i-Azam University Islamabad Paksitan. He got his Ph.D degree from Kyoto University, (Japan) in 1994. He is (UNESCO Laureate), President – National Council for Tibb Treasurer, the Association of Academies and Societies of Sciences in Asia (AASSA), Fellow, Islamic World Academy of Sciences and Secretary General at Pakistan Academy of Sciences,
ORCID
Muhammad Ayaz http://orcid.org/0000-0002-4299-2445
References
- Abou El-Nour, K.M.M.; Eftaiha, A.; Al-Warthan, A.; Ammar, R.A.A. Synthesis and Applications of Silver Nanoparticles. Arabian Journal of Chemistry 2010, 3 (3), 135–140.
- Iravani, S. Green Synthesis of Metal Nanoparticles Using Plants. Green Chem. 2011, 13 (10), 2638–2650.
- Chaloupka, K.; Malam, Y.; Seifalian, A.M. Nanosilver as a New Generation of Nanoproduct in Biomedical Applications. Trends Biotechnol. 2010, 28 (11), 580–588.
- Prow, T.W.; Grice, J.E.; Lin, L.L.; Faye, R.; Butler, M.; Becker, W.; Wurm, E.M.T.; Yoong, C.; Robertson, T.A.; Soyer, H.P.; Roberts, M.S. Nanoparticles and Microparticles for Skin Drug Delivery. Adv. Drug Delivery Rev. 2011, 63 (6), 470–491.
- Ovais, M.; Ahmad, I.; Khalil, A.T.; Mukherjee, S.; Javed, R.; Ayaz, M.; Raza, A.; Shinwari, Z.K. Wound Healing Applications of Biogenic Colloidal Silver and Gold Nanoparticles: Recent Trends and Future Prospects. Appl. Microbiol. Biotechnol. 2018, 102 (10), 4305–4318.
- Nadeem, M.; Abbasi, B.H.; Younas, M.; Ahmad, W.; Khan, T. A Review of the Green Syntheses and Anti-microbial Applications of Gold Nanoparticles. Green Chem. Lett. Rev. 2017, 10 (4), 216–227.
- Kemp, M.M.; Kumar, A.; Mousa, S.; Park, T.-J.; Ajayan, P.; Kubotera, N.; Mousa, S.A.; Linhardt, R.J. Synthesis of Gold and Silver Nanoparticles Stabilized with Glycosaminoglycans Having Distinctive Biological Activities. Biomacromolecules 2009, 10 (3), 589–595.
- Mittal, J.; Batra, A.; Singh, A.; Sharma, M.M. Phytofabrication of Nanoparticles through Plant as Nanofactories. Adv. Nat. Sci.: Nanosci. Nanotechnol. 2014, 5 (4), 043002.
- Narayanan, K.B.; Sakthivel, N. Biological Synthesis of Metal Nanoparticles by Microbes. Adv. Colloid Interface Sci. 2010, 156 (1-2), 1–13.
- Segal, R.; Feuerstein, I.; Danin, A. Chemotypes of Artemisia Herba-Alba in Israel Based on their Sesquiterpene Lactone and Essential oil Constitution. Biochem. Syst. Ecol. 1987, 15 (4), 411–416.
- Kakar, S.A.; Tareen, R.B.; Kakar, M.A.; Jabeen, H.; Kakar, S.U.R.; Al-Kahraman, Y.M.S.A.; Shafee, M. Screening of Antibacterial Activity of Four Medicinal Plants of Balochistan-Pakistan. Pak. J. Bot 2012, 44, 245–250.
- Ayaz, M.; Junaid, M.; Ullah, F.; Subhan, F.; Sadiq, A.; Ali, G.; Ovais, M.; Shahid, M.; Ahmad, A.; Wadood, A.; El-Shazly, M.; Ahmad, N.; Ahmad, S. Anti-Alzheimer’s Studies on β-Sitosterol Isolated from Polygonum hydropiper L. Front. Pharmacol. 2017, 8, 697.
- Ovais, M.; Khalil, A.T.; Raza, A.; Islam, N.U.; Ayaz, M.; Saravanan, M.; Ali, M.; Ahmad, I.; Shahid, M.; Shinwari, Z.K. Multifunctional Theranostic Applications of Biocompatible Green-Synthesized Colloidal Nanoparticles. Appl. Microbiol. Biotechnol. 2018, 102 (10), 4393–4408.
- Zohra, T.; Ovais, M.; Khalil, A.T.; Qasim, M.; Ayaz, M.; Shinwari, Z.K.; Ahmad, S.; Zahoor, M. Bio-guided Profiling and HPLC-DAD Finger Printing of Atriplex Lasiantha Boiss. BMC Complement. Altern. Med. 2019, 19, 4.
- Zohra, T.; Ovais, M.; Khalil, A.T.; Qasim, M.; Ayaz, M.; Shinwari, Z.K. Extraction Optimization, Total Phenolic, Flavonoid Contents, HPLC-DAD Analysis and Diverse Pharmacological Evaluations of Dysphania Ambrosioides (L.) Mosyakin & Clemants. Nat. Prod. Res. 2018, 33 (1), 136–142.
- Ullah, F.; Ayaz, M.; Sadiq, A.; Hussain, A.; Ahmad, S.; Imran, M.; Zeb, A. Phenolic, Flavonoid Contents, Anticholinesterase and Antioxidant Evaluation of Iris Germanica var; Florentina. Nat. Prod. Res. 2016, 30 (12), 1440–1444.
- Anjum, S.; Abbasi, B.H. Biomimetic Synthesis of Antimicrobial Silver Nanoparticles Using in Vitro-Propagated Plantlets of a Medicinally Important Endangered Species: Phlomis Bracteosa. Int. J. Nanomed. 2016, 11, 1663.
- Ayaz, M.; Subhan, F.; Ahmed, J.; Khan, A.-U.; Ullah, F.; Ullah, I.; Ali, G.; Syed, N.-I.-H.; Hussain, S. Sertraline Enhances the Activity of Antimicrobial Agents Against Pathogens of Clinical Relevance. J. Biol. Res.-Thessaloniki 2015, 22 (1), 4.
- Ayaz, M.; Junaid, M.; Ullah, F.; Sadiq, A.; Ovais, M.; Ahmad, W.; Ahmad, S.; Zeb, A. Chemical Profiling, Antimicrobial and Insecticidal Evaluations of Polygonum hydropiper L. BMC Complement. Altern. Med. 2016, 16 (1), 502.
- Ayaz, M.; Subhan, F.; Ahmed, J.; Khan, A.-U.; Ullah, F.; Sadiq, A.; Syed, N.I.H.; Ullah, I.; Hussain, S. Citalopram and Venlafaxine Differentially Augments Antimicrobial Properties of Antibiotics. Acta Polon Pharm Drug Res 2015, 72 (6), 1269–1278.
- Tabassum, S.; Ahmed, M.; Mirza, B.; Naeem, M.; Zia, M.; Shanwari, Z.K.; Khan, G.M. Appraisal of Phytochemical and in Vitro Biological Attributes of an Unexplored Folklore: Rhus Punjabensis Stewart. BMC Complement. Altern. Med. 2017, 17 (1), 146.
- Ihsan-Ul-Haq, A.B.D.U.L.; Ahmed, I.B.R.A.R.; Hussain, I.Z.H.A.R.; Jamil, M.A.R.Y.A.M.; Mirza, B.U.S.H.R.A. Antibacterial Activity and Brine Shrimp Toxicity of Artemisia Dubia Extract. Pak. J. Bot. 2012, 44 (4), 1487–1490.
- Ayaz, M.; Sadiq, A.; Wadood, A.; Junaid, M.; Ullah, F.; Zaman Khan, N., Cytotoxicity and Molecular Docking Studies on Phytosterols Isolated from Polygonum hydropiper L. Steroids 2019, 141, 30–35.
- Makarov, V.V.; Love, A.J.; Sinitsyna, O.V.; Makarova, S.S.; Yaminsky, I.V. “Green” Nanotechnologies: Synthesis of Metal Nanoparticles using Plants. Acta Naturae. 2014, 6 (1), 35–44.
- Ovais, M.; Khalil, A.; Ayaz, M.; Ahmad, I.; Nethi, S.; Mukherjee, S. Biosynthesis of Metal Nanoparticles via Microbial Enzymes: A Mechanistic Approach. Int. J. Mol. Sci. 2018, 19 (12), 4100.
- Oluwaniyi, O.O.; Adegoke, H.I.; Adesuji, E.T.; Alabi, A.B.; Bodede, S.O.; Labulo, A.H.; Oseghale, C.O. Biosynthesis of Silver Nanoparticles using Aqueous Leaf Extract of Thevetia Peruviana Juss and its Antimicrobial Activities. Appl. Nanosci. 2016, 6 (6), 903–912.
- Arunachalam, K.D.; Arun, L.B.; Annamalai, S.K.; Arunachalam, A.M. Potential Anticancer Properties of Bioactive Compounds of Gymnema Sylvestre and its Biofunctionalized Silver Nanoparticles. Int. J. Nanomed. 2015, 10, 31–41.
- Nasar, M.Q.; Khalil, A.T.; Ali, M.; Shah, M.; Ayaz, M; Shinwari, Z.K. Phytochemical Analysis, Ephedra Procera CA Mey. Mediated Green Synthesis of Silver Nanoparticles, Their Cytotoxic and Antimicrobial Potentials. Medicina. 2019, 55 (7), 369.
- Mittal, A.K.; Kaler, A.; Banerjee, U.C. Free Radical Scavenging and Antioxidant Activity of Silver Nanoparticles Synthesized from Flower Extract of Rhododendron Dauricum. Nano. Biomed. Eng. 2012, 4 (3), 118–124.
- Khalil, A.T.; Ayaz, M.; et al. In Vitro Cholinesterase Enzymes Inhibitory Potential and in Silico Molecular Docking Studies of Biogenic Metal Oxides Nanoparticles. Inorganic and Nano-Metal Chemistry. 2018, 48 (9), 441–448.
- Balashanmugam, P.; Kalaichelvan, P.T. Biosynthesis Characterization of Silver Nanoparticles using Cassia Roxburghii DC. Aqueous Extract, and Coated on Cotton Cloth for Effective Antibacterial Activity. Int. J. Nanomed. 2015, 10 (Suppl. 1), 87.
- Ayaz, M. Cellular Efflux Transporters and the Potential Role of Natural Products in Combating Efflux Mediated Drug Resistance. Front. Biosci. 2017, 22, 732–756.
- Ullah, F.; Malik, S.A.; Ahmed, J.; Shah, S.M.; Ayaz, M.; Hussain, S.; Khatoon, L. Investigation of the Genetic Basis of Tetracycline Resistance in Staphylococcus Aureus From Pakistan. Tropical Journal of Pharmaceutical Research 2012, 11 (6), 925–931.
- Arokiyaraj, S.; Valan Arasu, M.; Vincent, S.; Oh, Y.-K.; Kim, K.H.; Choi, K.-C.; Choi, S.H.; Prakash, N.U. Rapid Green Synthesis of Silver Nanoparticles From Chrysanthemum Indicum L and its Antibacterial and Cytotoxic Effects: an in Vitro Study. Int. J. Nanomed. 2014, 9, 379.
- Guzman, M.; Dille, J.; Godet, S. Synthesis and Antibacterial Activity of Silver Nanoparticles against Gram-Positive and Gram-Negative Bacteria. Nanomed. Nanotechnol. Biol. Med. 2012, 8 (1), 37–45.
- Song, H.Y.; Ko, K.K.; Oh, I.H.; Lee, B.T. Fabrication of Silver Nanoparticles and Their Antimicrobial Mechanisms. Eur Cells Mater 2006, 11 (Suppl 1), 58.
- Murray, R.; Steed, P.; Elson, H. The Location of the Mucopeptide in Sections of the Cell Wall of Escherichia Coli and Other Gram-Negative Bacteria. Can. J. Microbiol. 1965, 11 (3), 547–560.
- Ovais, M.; Hayat, S,; Fakhar-E-Alam, M,; Aslam, B.; Siddique, M.H.; Nisar, M.A.; Saqalein, M.; Atif, M.; Sarwar, A.; Khurshid, A.; Amin, N.; Wang, Z. Nanoantibiotics: Recent Developments and Future Prospects. Front. Clin. Drug Res. – Anti-Infect. 2018, 5, 30–54.
- Elangovan, K.; Elumalai, D.; Anupriya, S.; Shenbhagaraman, R.; Kaleena, P.K.; Murugesan, K. Phyto Mediated Biogenic Synthesis of Silver Nanoparticles Using Leaf Extract of Andrographis Echioides and its bio-Efficacy on Anticancer and Antibacterial Activities. J. Photochem. Photobiol., B 2015, 151, 118–124.
- Mata, R.; Nakkala, J.R.; Sadras, S.R. Biogenic Silver Nanoparticles from Abutilon Indicum: Their Antioxidant, Antibacterial and Cytotoxic Effects in Vitro. Colloids Surf., B 2015, 128, 276–286.
- Baharara, J.; Namvar, F.; Ramezani, T.; Mousavi, M.; Mohamad, R. Silver Nanoparticles Biosynthesized using Achillea Biebersteinii Flower Extract: Apoptosis Induction in MCF-7 Cells via Caspase Activation and Regulation of Bax and Bcl-2 Gene Expression. Molecules 2015, 20 (2), 2693–2706.
- Šljukić, B.; Banks, C.E.; Crossley, A.; Compton, R.G. Lead (IV) Oxide–Graphite Composite Electrodes: Application to Sensing of Ammonia, Nitrite and Phenols. Anal. Chim. Acta 2007, 587 (2), 240–246.
- Ovais, M.; Khalil, A.T.; Islam, N.U.; Ahmad, I.; Saravanan, M.; Shinwari, Z.K.; Mukherjee, S. Role of plant phytochemicals and microbial enzymes in biosynthesis of metallic nanoparticles. Appl. Microbiol. Biotechnol. 2018, 102 (16), 6799–6814.
- Chen, L.Q.; Fang, L.; Ling, J.; Ding, C.Z.; Kang, B.; Huang, C.Z. Nanotoxicity of Silver Nanoparticles to red Blood Cells: Size Dependent Adsorption, Uptake, and Hemolytic Activity. Chem. Res. Toxicol. 2015, 28 (3), 501–509.
- Rothen-Rutishauser, B.M.; Schürch, S.; Haenni, B.; Kapp, N.; Gehr, P. Interaction of Fine Particles and Nanoparticles with red Blood Cells Visualized with Advanced Microscopic Techniques. Environ. Sci. Technol. 2006, 40 (14), 4353–4359.