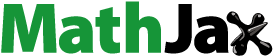
ABSTRACT
In the green Knoevenagel reaction of benzaldehyde and malonic acid, the carbon–carbon formation reaction is catalyzed by a double Schiff base formed by benzaldehyde and ammonia. A mechanism for this green Knoevenagel has been proposed. A closed catalytic cycle explains why various ammonium salts, including ammonium bicarbonate, have been correctly reported as catalysts in Knoevenagel-like reactions. Various catalytically active intermediates from derivatives of benzaldehyde were isolated and characterized. Furthermore, these in situ formed double Schiff bases play another catalytic role in the consecutive decarboxylation reaction to various cinnamic acids.
GRAPHICAL ABSTRACT
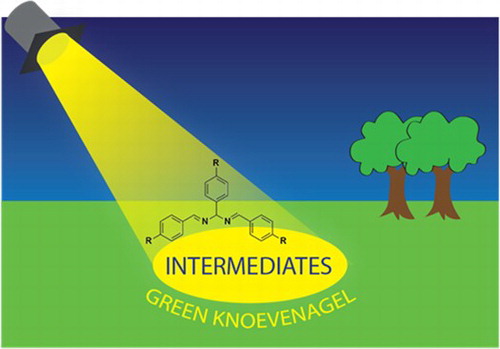
1. Introduction
Catalysis is an vital specialism of chemistry and this is emphasized by the chemical industry where 85–90% of all products are produced by catalytic processes (Citation1). The start of industrial catalysis was by the end of the nineteenth century and the beginning of the twentieth century (Citation2). One of the most important catalytic processes developed industrially is the Haber–Bosch process which uses a simple iron catalyst to make ammonia and other nitrogen-containing compounds from nitrogen and hydrogen (Citation3). The significant influence of the Haber–Bosch process on sustainability is that ammonia production made inexpensive fertilizers available to the world, allowing many people to grow a steady abundance of food for the first time (Citation4).
Catalytically promoted aldol condensations are important in organic synthesis as they provide an efficient way to form carbon–carbon bonds, which are the basis of organic chemistry (Citation5). The aldol condensation can either be acid-catalyzed or base-catalyzed. A base-catalyzed aldol condensation in the presence of an amine is called a Knoevenagel condensation. In 1898 Emil Knoevenagel was the first who realized that amines were truly catalytic (“Contactsubstanz”). He isolated catalytic intermediates and as a result of this laid the fundamentals of “organocatalysis” (Citation6,Citation7). Unfortunately, the impact of his research has not yet been valued by everyone (Citation8–11). Organocatalysis is the catalysis of reactions with small organic molecules and generally seen as a more environmentally friendly form of catalysis opposed to for example (toxic) transition metal catalysts (Citation12,Citation13).
It is well known for a long time that benzaldehyde and ammonia react together to form hydrobenzamide which is a double Schiff base (Citation14–19). Schiff bases have been playing vital roles in e.g. pharmaceuticals, as a protective group, and in liquid crystals (Citation20–23). In combination with various metals but never alone, it has further been shown that hydrobenzamide exhibits catalytic activity in the field of interactions with biogenic macromolecules such as DNA, RNA, and peptides (Citation24).
In the pursuit to develop eco-friendly Knoevenagel condensation reactions to produce ligno-phytochemicals, we reported earlier about a green Knoevenagel method with ammonium bicarbonate as a catalyst and source of ammonia (Citation25). This method provides good to an excellent conversion of various benzaldehydes and high yields of the corresponding cinnamic acids in an efficient, rapid, scalable, and environmentally friendly way. The question arose to what extent hydrobenzamide-like intermediates could be isolated and whether these intermediates could be identified as catalytically active (Citation25,Citation26). This study investigates the organocatalytic activity of various “in situ formed” hydrobenzamides in the green Knoevenagel reaction.
2. Results and discussion
2.1. Catalytical activity of hydrobenzamide
The double Schiff base hydrobenzamide 2a (1-[(phenyl)]-N,N'-bis [(phenyl)methylene] methanediamine) synthesized from benzaldehyde and ammonium bicarbonate was isolated with a high yield (>95%) as reported before (Citation27). To demonstrate that hydrobenzamide 2a is catalytically active and therefore part of a catalytic mechanism, various amounts of hydrobenzamide 2a were added to a mixture of benzaldehyde and malonic acid at 60°C. shows cinnamic dicarboxylic acid 4a formed as a function of time. Under these conditions, benzaldehyde 1a is both reagent to the product cinnamic dicarboxylic acid 4a (Scheme 1) and also a continuously incorporated part of the catalytic intermediate 2a. In the design of the experiment, it was therefore decided that the reported mol percentages of hydrobenzamide 2a were calculated relative to malonic acid and the total molar amount of benzaldehyde (free and incorporated in 2a) was kept equal to the molar amount of malonic acid 3.
Figure 1. Concentration-time history of cinnamic dicarboxylic acid 4a formed using various amounts of hydrobenzamide 2a in a mixture with an equal total amount of benzaldehyde 1a and malonic acid 3 at 60°C. Reported conversions are based on the ratio of peaks of the HPLC chromatogram measured with a UV detector operating at 300 nm.
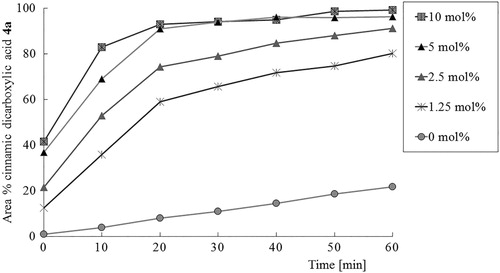
Scheme 1. Knoevenagel condensation of benzaldehyde 1a with malonic acid 3 with hydrobenzamide 2a as a catalyst towards cinnamic dicarboxylic acid 4a.
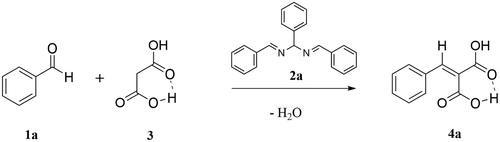
clearly shows that hydrobenzamide 2a accelerates the conversion of benzaldehyde 1a towards its corresponding cinnamic dicarboxylic acid 4a. Using 10 mol% hydrobenzamide 2a full conversion towards cinnamic dicarboxylic acid is reached within 1 h. To emphasize the impact of the catalytic intermediate hydrobenzamide: with a catalytic amount of only 1.25 mol% of hydrobenzamide 2a, complete conversion of benzaldehyde 1a towards cinnamic dicarboxylic acid 4a is reached within 2 h! Note that without the addition of hydrobenzamide 2a, the product cinnamic dicarboxylic acid 4a is also formed because of the carboxylic groups of malonic acid 3 also have acid catalytic activity in the condensation reaction. However, the results collected in reveals that this contribution is much smaller in comparison to the catalytic activity of hydrobenzamide 2a.
2.2. Mechanism condensation step
Now hydrobenzamide 2a has been demonstrated to play a catalytic role in the reaction from benzaldehyde 1a to cinnamic dicarboxylic acid 4a, a mechanism is proposed in Scheme 2 with hydrobenzamide 2a as a catalytic intermediate. On top of Scheme 2, the build-up is shown: precursor ammonia originated from and formed after decomposition of NH4HCO3, reacts with benzaldehyde 1a and results in benzyl imine I (step i). Benzyl imine I could not be isolated, due to its high reactivity but could be identified in the reaction mixture using MS (Supporting Information S35). Two molecules of benzyl imine I can subsequently combine into intermediate II, which has been identified in the reaction mixture using MS (step ii). Finally, a third benzaldehyde 1a is incorporated into intermediate II, resulting in the production of hydrobenzamide 2a which forms the basis of the catalytic cycle marked by the green circle at the lower part of Scheme 2 (step iii).
Scheme 2. Proposed mechanism of the reaction between the reactants benzaldehyde 1a and malonic acid 3 towards the product cinnamic dicarboxylic acid 4a catalyzed by in situ formed hydrobenzamide 2a.
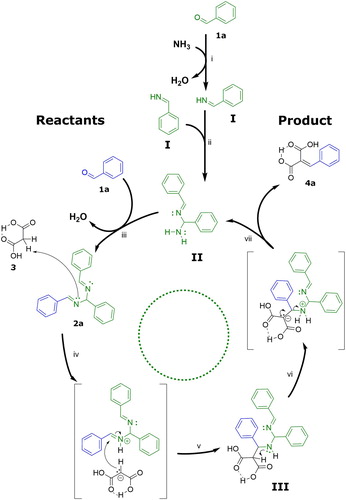
First, a proton from the α-carbon of the reactant malonic acid 3 is transferred to an imine in hydrobenzamide 2a (step iv). The ion pair created, reacts to form a carbon–carbon bond (step v). Intermediate III formed, could also be identified with MS measurements (Supporting Information S35). A second proton from the α-carbon of the malonic acid moiety is removed by the former imine nitrogen atom (step vi) and the desired product cinnamic dicarboxylic acid 4a is formed in step vii. Intermediate II is formed as an accompanying product and regenerates hydrobenzamide 2a again after coupling with benzaldehyde 1a (step iii) closing the catalytic cycle.
2.3. Catalytically active hydrobenzamide analogs
Also, other catalytically active hydrobenzamide analogs without a phenolic group were isolated successfully, and to the best of our knowledge, some of these catalytic intermediates are characterized for the first time as demonstrated by the results collected in . Many other catalytic intermediates still are to be explored because each benzaldehyde derivative generates its catalytic intermediate. Purification of the double Schiff bases of the non-phenolic derivatives was possible by suspending the obtained precipitate in 30% ammonia solution and subsequently removing the liquid by filtration. It was further noticed that the synthesized and purified hydrobenzamides were not only catalytically active for the corresponding benzaldehyde but were often to the same extent catalytically active for a different type of benzaldehyde. For instance, with equal amounts of benzaldehyde 1a and malonic acid 3 and 10 mol% of the catalytically active hydrobenzamide 2a at 60°C, cinnamic dicarboxylic acid 4a was formed. However, after addition of equal amounts of 4-methyl benzaldehyde 1b and malonic acid 3 to the former reaction mixture also a 4-methyl cinnamic dicarboxylic acid 4b was produced. Other combinations of catalysts and benzaldehydes derivatives could be carried out interchangeably. The catalytic intermediates of non-phenolic benzaldehydes derivatives were synthesized, purified, characterized by 1H-NMR, 13C-NMR, MS, TGA, and they all showed the expected catalytic activity in the condensation reaction with their corresponding benzaldehyde 1 and malonic acid 3. The range of melting points is broad and is of no importance in the condensation reaction because all these intermediates are formed in situ during the green Knoevenagel reaction and do not show up isolated.
Table 1. Various hydrobenzamides 2 obtained from benzaldehyde derivatives 1
Unfortunately, we were not able to isolate and characterize the putative catalytic intermediates from benzaldehydes with a phenolic group at the para position e.g. 4-hydroxy benzaldehyde 1j, vanillin 1k, and syringaldehyde 1l. The results of the indirect analysis of the fragments in the reaction mixture using MS (Supporting Information S36–S38) points to the presence of the in situ formed catalytic double Schiff bases. Due to the absence of the phenolic group on the para position, the acetylated intermediates are more stable, and the melting points of all three acetylated benzaldehydes could be determined and presented in . However, only the double Schiff base of 4-acetoxy-syringaldehyde 2o could be characterized by 1H-NMR. Characterization of the double Schiff base of 4-acetoxy-benzaldehyde 2m and 4-acetoxy-vanillin 2n with NMR proved to be impossible due to instability in various solvents. All three of these derivatized hydrobenzamides showed catalytic activity in the Knoevenagel condensation reaction with the corresponding benzaldehyde 1 and malonic acid 3. Also, the same catalytic activity was shown with benzaldehyde 1a and malonic acid 3 underlying that the catalytic intermediates can be used interchangeably.
2.4. Decarboxylation
The original Knoevenagel reaction involves a condensation step in which piperidine is often used as an organocatalyst. We demonstrated that piperidine could be replaced successfully with precursor ammonia, released from ammonium salts. In our green Knoevenagel reaction, the in situ produced double Schiff base plays a role as a catalytic intermediate in this condensation step where cinnamic dicarboxylic acids are produced (Citation25).
This condensation step is followed in the Doebner modification by a decarboxylation step to cinnamic acids, often using pyridine as a solvent and organocatalyst (Citation13,Citation28). Since cinnamic acids are produced from the cinnamic dicarboxylic acids after raising the original reaction mixture to a temperature of 110°C there is an indication that hydrobenzamide 2a or degradation products of hydrobenzamide 2a also participate in this subsequent decarboxylation step (Citation29–31). This was also based on previous observations where purified cinnamic dicarboxylic acids and so without the presence of the double Schiff base, did not show any decarboxylation at a temperature of 110°C (Citation25).
shows the decarboxylation of purified cinnamic dicarboxylic acid 4a performed at a temperature of 110°C. Hydrobenzamide 2a accelerates the decarboxylation of cinnamic dicarboxylic acid 4a towards cinnamic acid 5a. Without the addition of a hydrobenzamide 2a, no significant decarboxylation occurs. Using 10 mol% hydrobenzamide 2a almost full conversion towards cinnamic acid 5a is reached within 2 h. However, the consecutive decarboxylation towards styrene is visible for approximately 10%, which reduces the yield of cinnamic acid 5a. With lower amounts or hydrobenzamide 2a, virtually no styrene formation is visible. Our data reveal that the decarboxylation of cinnamic dicarboxylic acid 4a obeys first-order kinetics which follows from a linear relationship between and time (Supporting Information S47).
Figure 2. Decarboxylation of cinnamic dicarboxylic acid 4a using various amounts of hydrobenzamide 2a (HBA) at 110°C. Cinnamic dicarboxylic acid 4a and hydrobenzamide 2a were mixed with a minimum amount (e.g. 0.5 mL) of ethyl acetate after which ethyl acetate was removed by distillation under reduced pressure at 40°C. Reported conversions are based on the ratio of peaks of the HPLC chromatogram measured with a UV detector operating at 300 nm.
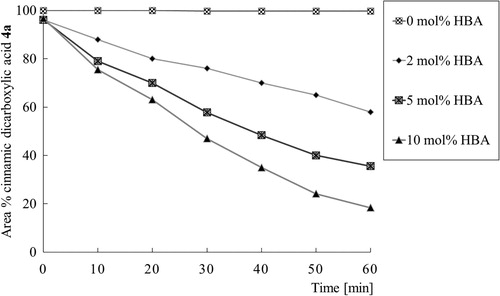
A possible explanation of the organocatalysis of the decarboxylation by hydrobenzamide 2a can be found in the suggestion that tertiary amines can serve as a Lewis base (Citation32,Citation33). As shown in Scheme 3, hydrobenzamide 2a deprotonates cinnamic dicarboxylic acid 4a and creates a carboxylate anion. Increasing the temperature leads to decarboxylation and finally, cinnamic acid 5a is formed.
2.5. Thermal stability hydrobenzamides
Because the decarboxylation is performed at an elevated temperature (i.e. 110°C), the thermal stability of hydrobenzamide 2a is essential. The thermal stability of hydrobenzamide 2a was therefore investigated with thermogravimetric analysis (TGA). The feature of this analysis is to determine the initial decomposition temperature (onset), the temperature when 50 wt.% of hydrobenzamide is decomposed (D½), and the final residue in a nitrogen atmosphere (Citation34). As demonstrated by the results given in , the TGA curve of hydrobenzamide 2a has an onset at a temperature of 254°C, a D½ at a temperature of 281°C and above a temperature of 320°C there is no residue left. Above a temperature of 320°C, the in situ formed catalytic intermediate has entirely disappeared. In our opinion, at a decarboxylation temperature of 110°C hydrobenzamide 2a is stable to act as a Lewis base in the decarboxylation of dicarboxylic cinnamic acid 4a. Thermographs of other hydrobenzamides are shown in Supporting Information (S39–S46).
Figure 3. TGA thermograph of hydrobenzamide 2a. TGA analysis was performed with a 20°C/min heating rate in nitrogen.
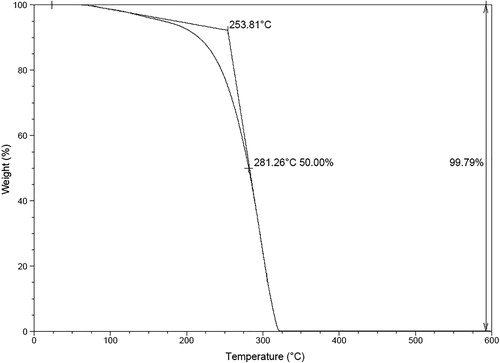
In summary, it can be concluded that in situ formed catalytic intermediates have a dual function in the green Knoevenagel. First, they are a catalytic intermediate in the condensation reaction of coupling benzaldehyde derivatives with malonic acid and play another catalytic role in the consecutive decarboxylation step. More research needs to be done on aspects of formation, stability, and catalytic activity of these intermediates in the green Knoevenagel reaction.
3. Conclusion
It has been demonstrated that in the green Knoevenagel reaction of benzaldehyde 1a and malonic acid 3, the carbon–carbon formation reaction is catalyzed by an in situ formed double Schiff base. A mechanism for this green Knoevenagel reaction has been proposed. In the proposed mechanism benzaldehyde react with the precursor ammonia, released from ammonium salts to form a catalytically active double Schiff base. A closed catalytic cycle explains why various ammonium salts, including ammonium bicarbonate, have been correctly reported as catalysts in Knoevenagel-like reactions. Several catalytically active intermediates from derivatives of benzaldehyde were isolated and characterized. Unfortunately, it was not possible to isolate or characterize the benzaldehyde derivatives with a phenolic-group at the para position of benzaldehyde. However, the results of mass spectrometry points to the existence of these putative in situ formed catalytic intermediates. Furthermore, these in situ formed double Schiff bases play another catalytic role in the consecutive decarboxylation reaction to various cinnamic acids.
4. Materials and methods
4.1. Methods
1H-NMR measurements were performed on an Agilent 400-MHz NMR system with DMSO-d6 as a solvent. Data was acquired using VnmrJ3 software. Chemical shifts are reported in ppm, relative to tetramethylsilane (TMS).
HPLC analysis was carried out using a reversed phase liquid chromatographic system (Agilent 1100 series) equipped with a diode array detector operating at 300 nm (Agilent 1100 series) and an autosampler injector with a 20 µL loop (Agilent 1100 series G1316A). The system was equipped with a Grace Alltima C18 5 µm column (250 × 4.6 mm). The determination of the concentration of the components in the reaction mixtures was carried out by the external standard method and was based on peak areas. HPLC measurements were made with the following settings: Flowrate 1.0 mL/min at a temperature of 20°C, eluens A = methanol, eluens B = acetic acid buffer (99% milliQ, 1% acetic acid). The samples were eluted using the following gradient: 40% A and 60% B set for 6 min, gradient to 100% A and 0% B in subsequent 10 min, 100% A and 0% B set for 5 min, followed by 40% A and 60% B set for 8 min.
LC-MS analysis was performed using a liquid chromatographic system (Agilent 1200 series) equipped with a Luna 3 µm C18 column (200 × 2,0 mm) and an ion trap (Agilent 6300 series).
Thermogravimetric analysis (TGA) was performed on a Q50 TA Instruments TGA, using nitrogen as purge gas, at a heating rate of 20°C/min in the range of 0–600°C.
Melting points were determined with Hanon Automatic Video Melting Point apparatus MP450.
4.2. Synthetic procedure
Optimized protocol for the synthesis of hydrobenzamide (2a):
A mixture of benzaldehyde (1a, 1.44 g, 15 mmol) and ammonium bicarbonate (0.79 g, 10 mmol) was brought to a temperature of 50°C, resulting in a solid white substance in 30 min. The solid was purified by suspending the obtained precipitate in 10 mL 30% (m/m) ammonia and subsequently removing the solvent by filtration.
Protocol for the green Knoevenagel condensation towards cinnamic dicarboxylic acid 4a with 10 mol% hydrobenzamide 2a:
Malonic acid 3 (1.0 g, 10 mmol) was dissolved in benzaldehyde (1a, 0.96 g, 10.0 mmol) and hydrobenzamide (2a, 0.26 g, 1.0 mmol) was subsequently added. The reaction mixture was stirred and kept at 60°C for 1 h to reach complete benzaldehyde conversion. Samples of 2 μl were taken for reversed phase HPLC analysis and diluted in 7.5 mL methanol, filtered, and analyzed using the method described in section 2.2. The calculated percentage of the peak area of cinnamic dicarboxylic acid (4a) is in relation to the total peak area.
Acknowledgement
The authors are grateful for the financial support from Netherlands Organization for Scientific Research (NWO) (grant 023.007.020 awarded to Jack van Schijndel). We further thank Sebastian van Schijndel for his help on the graphical abstract.
Disclosure statement
No potential conflict of interest was reported by the authors.
Notes on contributors
Jack van Schijndel studied chemistry at the State University of Utrecht with a major in the field of structural chemistry. He has been teaching Chemistry at the tertiary level for over 28 years and has supervised both undergraduate and postgraduate research projects in this field at the Avans University of Applied Sciences. His latest work focusses on the sustainable use of renewable resources for the polymer industry. The synthetic routes developed are furthermore designed along the lines of the Green Chemistry principles. He supervised as a senior scientist more than 60 projects with SME’s in the field of biopolymers. His main areas interest is green chemistry, polymer synthesis but also teaching and learning innovations.
Dennis Molendijk received the B.Sc. degree in Chemistry at Avans University of Applied Sciences and is working under the supervision of Jack van Schijndel. He is involved in all ongoing projects in the research laboratory and also guides younger students with their research projects. His current research is focused on the green synthesis of biobased blocks of biopolymers and polymerization of these.
Harmen Spakman is a student of the B.Sc. Degree in Chemistry at Avans University of Applied Sciences.
Edward Knaven is currently an academic technician in the Research group Analysis Techniques in Life Science of Avans University of Applied Sciences. His research interests lie in the study of mass spectrometry of organic compounds.
Luiz Alberto Canalle received the M.Sc. degree in chemistry from the Radboud University Nijmegen, The Netherlands, in 2006. He received his PhD. in bio-organic chemistry from the same university in 2010. Since 2012, he has been with Avans University of Applied Sciences, Breda, The Netherlands as a lecturer in organic chemistry and researcher in the field of biopolymers. His main areas interest are polymer synthesis; green chemistry and the research/teaching nexus.
Jan Meuldijk obtained his M.Sc. degree in chemistry at the State University of Utrecht in 1975. His PhD. work was in the field of inorganic and theoretical chemistry at the Free University of Amsterdam, graduation in 1979. After post-doctoral fellowships in solid state physics and chemical engineering at the State University of Groningen, he was appointed assistant professor in the field of Industrial Catalysis at the Eindhoven University of Technology in 1985. In 1988, he became associate professor in chemical reactor engineering. His work in the Chemical Reaction Engineering Group is focused on process development of complex chemical products. In the department of Chemical Engineering and Chemistry there is a close cooperation with the Polymer Chemistry Group and the Macromolecular and Organic Chemistry Group. As of September 1, 2010 he is full professor in Polymer Reaction Engineering and Scientific Director of the Post MSc. Program “Process and Product Design”.
ORCID
Jack van Schijndel http://orcid.org/0000-0002-2806-7536
Additional information
Funding
Notes
* The manuscript was written through contributions of all authors. All authors have given approval to the final version of the manuscript.
References
- Jacobsen, E.N.; Finney, N.S. Synthetic and Biological Catalysts in Chemical Synthesis: how to Assess Practical Utility. Chem. Biol. 1994, 1, 85–90.
- Heveling, J. Heterogeneous Catalytic Chemistry by Example of Industrial Applications. J. Chem. Educ. 2012, 89, 1530–1536.
- Wang, Q.; Guo, J.; Chen, P. Recent Progress Towards Mild-Condition Ammonia Synthesis. J. Energy Chem. 2019, 36, 25–36.
- Vu, M.; Sakar, M.; Do, T. Insights Into the Recent Progress and Advanced Materials for Photocatalytic Nitrogen Fixation for Ammonia (NH3) Production. Catalysts. 2018, 8, 621.
- Mandal, S.; Mandal, S.; Ghosh, S.K.; Ghosh, A.; Saha, R.; Banerjee, S.; Saha, B. Review of the Aldol Reaction. Synth. Commun. 2016, 46, 1327–1342.
- Knoevenagel, E. Condensation von Malonsäure mit Aromatischen Aldehyden Durch Ammoniak und Amine. Ber. Dtsch. Chem. Ges. 1898, 31, 2596–2619.
- Knoevenagel, E. Condensationen zwischen Malonester und Aldehyden unter dem Einfluss von Ammoniak und organischen Aminen. Ber. Dtsch. Chem. Ges. 1898, 31, 2585–2595.
- Davey, R.M.; Stamford, N.P.J. Catalytic Enamines from Dialkylamide-Dialkylacetals. Tetrahedron Lett. 2012, 53, 2537–2539.
- List, B. Emil Knoevenagel and the Roots of Aminocatalysis. Angewandte Chemie (International ed. in English) 2010, 49, 1730–1734.
- Wan, J.; Jing, Y.; Liu, Y.; Sheng, S. Metal-free Synthesis of Cyano Acrylates via Cyanuric Chloride-Mediated Three-Component Reactions Involving a Cascade Consists of Knoevenagel Condensation/Cyano Hydration/Esterification. RSC Adv. 2014, 4, 63997–64000.
- Joharian, M.; Morsali, A.; Azhdari Tehrani, A.; Carlucci, L.; Proserpio, D.M. Water-stable Fluorinated Metal–Organic Frameworks (F-MOFs) with Hydrophobic Properties as Efficient and Highly Active Heterogeneous Catalysts in Aqueous Solution. Green Chem. 2018, 20, 5336–5345.
- Raj, M.; Singh, V.K. Organocatalytic Reactions in Water. Chem. Commun. 2009, 44, 6687–6703.
- van Schijndel, J.; Canalle, L.A.; Smid, J.; Meuldijk, J. Conversion of Syringaldehyde to Sinapinic Acid Through Knoevenagel-Doebner Condensation. Open. J. Phys. Chem. 2016, 6, 101–108.
- Crowell, T.I.; McLeod, R.K. Kinetics of Hydrobenzamide Formation from p-Dimethylaminobenzaldehyde and Ammonia. Role of the Imine. J. Org Chem. 1967, 32, 4030–4033.
- Crowell, T.I.; Peck, D.W. Kinetic Evidence for a Schiff Base Intermediate in the Knoevenagel Condensation1. J. Am. Chem. Soc. 1953, 75, 1075–1077.
- Kamal, A.; Ahmad, A.; Qureshi, A.A. Syntheses of Some Substituted Hexamines in Aqueous Medium. Tetrahedron 1963, 19, 869–872.
- Nishiyama, K.; Saito, M.; Oba, M. Formation of N,N′-Disubstituted Methanediamine Derivatives From Hexamethyldisilazane and Aldehydes via Stepwise Reactions. Bull. Chem. Soc. Jpn. 1988, 61, 609–611.
- Sim, S.K.; Hunter, D.H. 2,4-Diazapentadienes. I. Prototropy, Cyclization, and Addition-Elimination. Can. J. Chem. 1972, 50, 669–677.
- Laurent, M.A. Ueber das Hydrobenzamid. Annalen der Pharmacie 1837, 21, 130–134.
- Rao, V.K.; Reddy, S.S.; Krishna, B.S.; Naidu, K.R.M.; Raju, C.N.; Ghosh, S.K. Synthesis of Schiff’s Bases in Aqueous Medium: A Green Alternative Approach with Effective Mass Yield and High Reaction Rates. Green Chem. Lett. Rev. 2010, 3, 217–223.
- Djemili, A.; Lakrout, S.; Cheraiet, Z.; Berredjem, M.; Aouf, N. A Simple and Highly Efficient Solvent- and Catalyst-Free Synthesis of Novel N-Sulfamoyl Imines. Green Chem. Lett. Rev. 2015, 8, 48–53.
- Lokhande, R.; Sonawane, J.; Roy, A.; Ravishankar, L. Solvent-free Reductive Amination of Aromatic Aldehydes Catalyzed by CeCl3·7H2O. Green Chem. Lett. Rev. 2011, 4, 69–72.
- Zhong, G.; Zhong, Q. Solid–Solid Synthesis, Characterization, Thermal Decomposition and Antibacterial Activities of Zinc(II) and Nickel(II) Complexes of Glycine–Vanillin Schiff Base Ligand. Green Chem. Lett. Rev. 2014, 7, 236–242.
- Gupta, K.C.; Sutar, A.K. Catalytic Activities of Schiff Base Transition Metal Complexes. Coord. Chem. Rev. 2008, 252, 1420–1450.
- van Schijndel, J.; Canalle, L.A.; Molendijk, D.; Meuldijk, J. The Green Knoevenagel Condensation: Solvent-Free Condensation of Benzaldehydes. Green Chem. Lett. Rev. 2017, 10, 404–411.
- Corey, E.J.; Kühnle, F.N.M. A Simplified Synthesis of (±)-1,2-Diphenyl-1,2-Diaminoethane (1) from Benzaldehyde and Ammonia. Revision of the Structures of the Long-Known Intermediates “Hydrobenzamide” and “Amarine”. Tetrahedron Lett. 1997, 38, 8631–8634.
- Francis, F. Notiz über die Einwirkung von Ammoniak auf Benzaldehyd und die Darstellung von Benzaldehyd-Ammoniak. Ber. Dtsch. Chem. Ges. 1909, 42, 2216–2218.
- Doebner, O. Synthese der Sorbinsäure. Ber. Dtsch. Chem. Ges. 1900, 33, 2140–2142.
- van Schijndel, J.; Molendijk, D.; Canalle, L.A.; Rump, E.T.; Meuldijk, J. Temperature Dependent Green Synthesis of 3-Carboxycoumarins and 3,4-Unsubstituted Coumarins. Curr. Org. Synth. 2019, 16, 130–135.
- Borah, A.J.; Yan, G. Decarboxylative Functionalization of Cinnamic Acids. Org. Biomol. Chem. 2015, 13, 8094–8115.
- Patra, T.; Maiti, D. Decarboxylation as the Key Step in C−C Bond-Forming Reactions. Chem. Eur. J. 2017, 23, 7382–7401.
- Oliveri, I.P.; Maccarrone, G.; Di Bella, S. A Lewis Basicity Scale in Dichloromethane for Amines and Common Nonprotogenic Solvents Using a Zinc(II) Schiff-Base Complex as Reference Lewis Acid. J. Org. Chem. 2011, 76, 8879–8884.
- Christian Laurence and Jean-François Gal Lewis Basicity and Affinity Scales: Data and Measurement; John Wiley & Sons Ltd: Chichester, U.K., 2010, pp 476.
- Tiwari, A.; Hihara, L.H. Thermal Stability and Thermokinetics Studies on Silicone Ceramer Coatings: Part 1-Inert Atmosphere Parameters. Polym. Degrad. Stab. 2009, 94, 1754–1771.