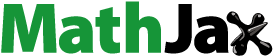
ABSTRACT
The synthesized ZnO NPs using durian rind in solution has shown maximum absorption at 355.5 nm with the bandgap of 3.33 eV, spectrophotometrically. SEM and TEM studies revealed that the shape of the synthesized ZnO NPs was spherical with an average size of 280 and 283 nm, respectively. However, DLS analysis of ZnO NPs revealed the average particle size of 456 d.nm. The presence of [100], [002], [101], [102], [110], [103], [200], [112] and [201] planes in XRD corroborate the formation of pure wurtzite structure of ZnO NPs. Synthesized ZnO NPs showed remarkable photocatalytic activity on degradation of methylene blue and sulfanilamide, antioxidant activity, considerable antimicrobial activity against Escherichia coli and Staphylococcus aureus, and considerable cytotoxic activity against brine shrimp. The sulfanilamide degradation was found to be 96.70%, under natural sunlight and in the presence of 0.1% ZnO NPs at pH 10 with a time of 3 h. The dye degradation was found to be 84% under sunlight in the presence of 0.01% ZnO NPs at pH 10 with a time of 40 min. The synthesized ZnO NPs may be explored furthermore in the fields of wastewater treatment, biomedicine, biosensor, and nanotechnology.
GRAPHICAL ABSTRACT
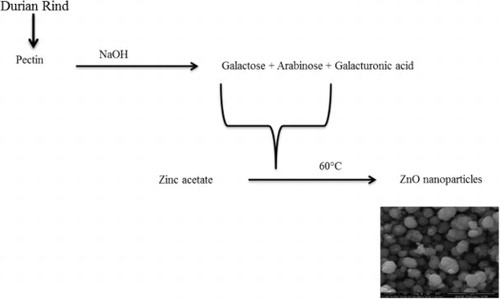
Introduction
Nanotechnology has transcended challenges of existing therapeutic strategies and transpired itself as a pioneer in the field, recently. Due to the exclusive chemical and physical properties of nanoparticles, these have been extensively explored in different domains viz. biology, catalysis, optics, medical and especially pharmaceutical sciences.
Numerous approaches, using chemicals, physical and electrochemical are used in the preparation of nanoparticles. Generally, the majority of the strategies face complications during purification stage since the utilized chemicals or the by-products formed are hazardous and do necessitate high energy for the preparation ( Citation1,Citation2). Apart from the toxicity problem, researchers have also encountered additional leading complications such as attainment of monodispersity and control over the size/shape during the preparation of nanoparticles. To mask all these problems, plant extracts were tremendously explored and gradually these have emerged as salient encouraging alternatives of nanoparticles synthesis. The plants containing constituents comprise protective as well as reduction properties which are essential for the reduction of metal ions to their corresponding nanoparticles ( Citation3,Citation4).
The current enigma of environmental pollution is indeed immense, especially when pesticides, dyes, industrial effluents, pharmaceuticals, and heavy metals contaminate the water streams continuously. The pollutants can be degraded by photocatalytic active nanostructural semiconductor metal oxides like TiO2 or ZnO under UV irradiation. ZnO is acclaimed as one of the best nanostructural semiconductor photocatalysts owing to its high photosensitivity, high catalytic activity, suitable bandgap, low cost and eco-friendliness ( Citation5).
Generally, zinc oxide nanoparticles are synthesized through wet chemical route ( Citation6), vapor phase process ( Citation7), hydrothermal ( Citation8), sonochemical and precipitation methods ( Citation9,Citation10). However, the requirements of expensive instruments, tedious process controlling and toxic reagents highly necessitate an uncomplicated green approach to synthesize zinc oxide nanoparticles. Several studies have reported successful biosynthesis of zinc oxide nanoparticles based on plants ( Citation11–15), fungi ( Citation16,Citation17) and bacteria ( Citation18–20). Zinc oxide nanoparticles possess several advantages including low cost, good gas sensing properties, photocatalytic and antimicrobial activity, possibility to prepare structure with proper optical properties (such as photonic crystals and catalytic materials) and non-toxicity in small quantity. Zinc oxide nanoparticles are significantly utilized in diversified industries pertaining to their specific characteristics such as anti-corrosion, anti-bacterial, photocatalytic, antioxidant, low electron conductivity and efficient heat resistance ( Citation21). These can also be used in numerous domains including laser and optoelectronic devices, surface acoustic wave and gas sensor devices. At room temperature, zinc oxide nanoparticles act as semiconductors with wide direct bandgap and exhibit large excitron binding energy (60 meV) ( Citation22). However, metal oxides and magnetic nanoparticles play an important role in the catalytic oxidation of organic wastes ( Citation23–26).
Durio zibethinus (Durian), member of Malvaceae family, has been acknowledged for antioxidant, anti-diabetic, anti-hyperlipidemic, anti-proliferative and antimicrobial activities ( Citation27). Moreover, studies also suggest about nutritional and salubrious properties of durian ( Citation28). Bioactive compounds including polyphenols, tannins, flavonoids, proteins, carbohydrates, vitamins B and C, calcium, potassium, iron, manganese, sodium, magnesium and copper which are omnipresent in the fruit, have attributed to medicinal properties of Durian ( Citation29). Moreover, the rind contains lignin, hemicelluloses, celluloses, and pectin. Pectin has been considered as an essential element for the biosynthesis and capping of ZnO NPs ( Citation30).
Thus, with the background information and a continuation of our previous studies ( Citation31,Citation32); the present study was particularly focused on aqueous extract of Durio zibethinus fruit waste (rind) based green synthesis of ZnO NPs along with the determination of biological and photocatalytic properties. Different influencing parameters such as zinc acetate concentration, reaction time, temperature and extract concentration were also studied. Moreover, antibacterial potential against Escherichia coli, Bacillus subtilis, and Staphylococcus aureus followed by antioxidant potential using 2,2-diphenyl-1-picrylhydrazyl (DPPH) radical scavenging method, photocatalytic activity against methylene blue and sulfanilamide under solar irradiation, and cytotoxicity potential against brine shrimp were evaluated for synthesized ZnO NPs.
Materials and methods
Materials
Zinc acetate dihydrate, Muller Hinton Agar, Nutrient broth, methylene blue, and DPPH were attained from Himedia Laboratories Pvt. Ltd., Mumbai, India. The cultures of bacterial strains were procured from the Faculty of Applied Sciences, AIMST University, Malaysia.
Preparation of durian seed extracts
Fresh and healthy durian rinds were collected from local (Sungai Petani, Kedah) market. Impurities or dust present over the rinds were washed away with distilled water followed by incising the rinds into smaller pieces. The rinds were soaked and rinsed with 70% methanol and air-dried at room temperature. About 20 grams of finely cut durian rinds were placed in a beaker comprising 200 mL of distilled water. The content was mixed thoroughly and heated at a constant temperature of 60°C for 10 min in a water bath, with occasional stirring. The solution was later quickly filtered through Buchner funnel which was equipped with two layers of Whatman No.1 double filter paper and connected to a vacuum pump. The collected filtrates were centrifuged at 5300 rpm for 15 min. The supernatant was mustered upon completion of centrifugation and the same was stored in an amber-colored bottle at 4°C ( Citation32).
Biosynthesis of ZnO NPs
Fifty milliliters of zinc acetate dihydrate solution (0.01 M) was measured and poured into a 100 mL beaker. 5 mL of rind extract (light yellow) was incorporated into zinc acetate dihydrate solution a drop at a time, at room temperature. The solution's pH was acclimatized to 12 by adding a sufficient quantity of 2M NaOH solution and the solution was stirred for 2 h to obtain a pale white aqueous solution followed by filtration. Meanwhile, color change of the aqueous solution from light yellow to pale white indicated the reduction of zinc acetate dihydrate to ZnO NPs which was confirmed by UV-Vis spectra. The resulting filtrate was allowed to dry at 60°C in an oven for a day to obtain ZnO NPs.
Optimization of reaction parameters
Specific parameters, viz. reaction time, extract volume, zinc acetate concentration, pH and reaction temperature are crucial for biosynthesis of ZnO NPs. Such parameters are advantageous for qualitative determination of yield, shape, size and agglomeration state of ZnO NPs. Therefore, optimization of the specified parameters for sustainable synthesis of ZnO NPs was carried out.
The optimization of zinc acetate concentration was accomplished by altering zinc acetate concentration from 0.01, 0.02 M to 0.05 M while keeping other parameters fixed. Reaction mixtures were stirred for 2 h at room temperature upon drop by drop addition of 2.5 mL of rind extract to 0.03 M solution of zinc acetate dihydrate in a beaker. The absorbance of the solution was measured spectrophotometrically and the obtained UV-Visible scan data were contemplated as a base for subsequent optimization study. Moreover, pH, extract volume and reaction temperature were also optimized similarly while maintaining pH at (10, 11 and 12), the extract volume to (1, 2.5 and 5 mL) and reaction temperature at (25°C, 40°C, 60°C and 80°C).
Colloidal stability of ZnO NPs
UV–visible spectroscopy was utilized to ascertain the long-term stability of ZnO NPs for which an aqueous dispersion of ZnO NPs was prepared and stored in a 50 mL volumetric flask (aluminum foil-covered) at ambient temperature. The sample aliquots were retracted every week for 120 days to measure UV–Visible spectra changes.
Characterization of ZnO NPs
Surface Plasmon Resonance (SPR) shift and plasmon intensity of colloidal ZnO NPs were determined by UV-Visible spectra (Shimadzu UV-Visible. spectrophotometer, model AV-1800) at the wavelength range of 300–700 nm. Morphology and size of ZnO NPs were confirmed by FESEM, an elemental composition by EDX analysis (FESEM-EDX, Oxford-Instrument INCA400), surface capping functional groups by FT-IR (FTIR-JASCO 4100 Spectrometer at 4000 cm−1–400 cm−1). The zeta potential and hydrodynamic particle size of ZnO NPs were evaluated with Zetasizer Ver. 7.03 (Malvern Instruments Ltd., Worcestershire, UK). The crystallinity of ZnO NPs was confirmed by XRD pattern of nanoparticles (Bruker AXS D-8 powder X-ray diffractometer (Shimadzu, Japan) operated at 40 kV voltage and 15 mA current using CuKα radiation (λ = 1.5406 A°)) ( Citation32).
Minimum inhibitory concentration (MIC)
MIC was ascertained by 1:2 serial dilution methods. Two Gram-positive (B. subtilis (ATCC 6633) and S. aureus (ATCC 43300)) and one Gram-negative (E. coli, (ATCC 25922)) bacterial strains were used. For determination of MIC, 6 sterilized test-tubes including the two test tubes as control were used. Ten milligrams of zinc oxide nanoparticles were dissolved in distilled water and volume was made up to 10 mL to attain 1000 μg/mL test solution. 2 mL of MH broth was transferred into each of the six test tubes whereby the 5th and 6th tubes were maintained as positive and negative controls, respectively. 2 mL solution of ZnO NPs was incorporated into test tube 1 (500 μg/mL). Serial dilution was performed to attain 250 and 62.5 μg/mL. Finally, 0.2 mL of standardized inoculums was incorporated into test tubes 1–5 and all the tubes were incubated at 37°C for 24 h. After incubation, the MIC value was ascertained by observing bacterial growth in test tubes.
Screening of antimicrobial activity
The well diffusion method was executed to determine the antibacterial activity of biosynthesized ZnO NPs against S. aureus and E. coli. With the help of a sterile cotton swab, the suspensions of bacterial strains comprising 105–106 CFU/ml final concentrations were swabbed onto sterile Mueller-Hinton Agar (MHA) plates. Four wells (∼6 mm diameters) were created in each agar plate with the help of sterile gel puncture. Standard ampicillin solution (1 mg/mL), zinc acetate dihydrate solution (1 mg/mL), durian rind extract and ZnO nanoparticles (1 mg/mL) + ampicillin (1 mg/mL), were used to fill the wells, individually in all the plates. Plates were incubated at 37°C for 24 hrs and upon incubation; zones of inhibition were measured in the plates, using a ruler ( Citation32).
In vitro antioxidant assay
DPPH method was utilized for determining free radical scavenging activity of ZnO NPs. DPPH (0.3 mM) solution in methanol was prepared and 250 µL of the solution was added to 3 ml of ZnO NPs solution in water at different concentrations (5, 10, 25 and 50 μg/ mL). Control was prepared by adding 3 mL of water to 250 μL of DPPH. The assay was repeated for three times to obtain an average value. “The decrease in absorbance on the addition of test samples was used to calculate antiradical activity, as expressed by percentage inhibition (% PI) of DPPH radical. The capability to scavenge the DPPH radical was calculated using the following equation (1):(1)
(1) where, Ac and As are the absorbances of the control and test samples, respectively, after 30 min measuring at 517 nm” (
Citation33). The IC50 value of the sample was assessed from the constructed linear regression plot.
Photocatalytic degradation of methylene blue using ZnO NPs
Photocatalytic property of ZnO NPs was determined by methylene blue (MB) degradation in the presence of ZnO NPs under solar exposure (
Citation34). Ten milligrams of ZnO NPs in 100 mL of distilled water was sonicated for 2 h. 1 mg MB powder was incorporated to the above aqueous ZnO NPs solution, stirred magnetically for 30 min in shadow, and the colloidal suspension was exposed to solar irradiation. The average atmosphere temperature during the experiment was maintained at 30°C with 40 min means shine duration. 5 mL of suspension was retracted from the colloidal mixer at every 5 min. Spectrophotometric scanning (200–800 nm) of collected suspension was performed to analyze the degradation of MB. The same procedure was followed for the solution containing ZnO NPs, MB with pH 10 and MB alone with pH 10 for comparison purpose. The dye degradation (%) was calculated by using the following equation (2):(2)
(2) where C0 is the initial concentration of MB solution and Ct is dye solution concentration after “t” hours in sunlight irradiation. The dye concentration absorption peaks at 662 nm were measured by UV–Visible spectroscopy (
Citation34).
Photocatalytic degradation of sulfanilamide using ZnO NPs
Photodegradation of sulfanilamide under solar exposure was performed by using 4 μg/mL of sulfanilamide solution. The solution was stirred magnetically for 30 min in shadow followed by exposure to solar irradiation. The average atmosphere temperature during the experiment was found to be 30°C with 5 h mean shine duration. At every 1 h, the solution from respective glass beaker was collected and scanned spectrophotometrically from 200 to 800 nm to study the degradation of sulfanilamide. The same procedure was followed for a solution containing 4 μg/mL of sulfanilamide and 0.1% ZnO NPs as photocatalyst for comparison purpose. The different parameters such as pH and time were optimized for both photodegradation and photocatalytic degradation reaction, and the concentration of ZnO NPs was optimized for photocatalytic degradation reaction of sulphanilamide. The degradation of sulphanilamide (%) was calculated by using the following equation (3):(3)
(3)
Where C0 is the initial concentration of sulfanilamide in solution and Ct is the concentration of the sulfanilamide in solution after “t” hours in the sunlight irradiation.
Cytotoxicity of DSAgNPs against Artemia salina
Artemia cytotoxicity assay is the simplest screening assays to analyze the toxicity of bioactive materials ( Citation35). Using capillary glass tube, ten healthy and live larvae of A. salina were transferred from brighter part of hatching chamber and placed into 24 well plates containing different concentrations (5–150 µg/mL) of ZnO NPs in 1 mL of sterilized seawater. Sterilized seawater devoid of ZnO NPs was used as negative control and was kept at 25°C for 24 h (16 h light-filled and 8 h dark-filled). After incubation, the larval mortality percentage was calculated from the number of larvae surviving in each test concentration. The assay was executed in triplicate and the LC50 was determined through regression plot.
Data analyses
Microsoft Excel 2010 package was utilized for the analysis of data.
Results and discussion
The sustainable (green) synthesis of zinc oxide nanoparticle was achieved by bio-reduction of zinc acetate dihydrate using Durio zibethinus rind aqueous extract as reducing and capping agent. Durian rinds were selected for the study as these are not only considered as waste material but these also cause environmental pollution upon discarding in open places. Moreover, easy and abundant availability of durian around the geographical region of research was another selection criterion to choose durian rinds for the study. Pectin, one of the major phytochemical constituents of durian rind, is an excellent antioxidizing agent ( Citation36) which is considered to be responsible for the reduction of Zn2+ in the reaction solution as well as to stabilize the formulated nanoparticles.
Mechanism involved in ZnO NPs formation
The present study dealt with the waste mediated ZnO NPs synthesis using durian rind aqueous extract, which was initiated by pectin present in the extract. Nadezhda et al. reported that the interaction of pectin with alkaline medium gives galacturonic acid and monosaccharides. Various monosaccharides produced in the reaction of pectin with the alkaline medium are rhamnose, arabinose, glucose, mannose, galactose and a minor quantity of xylose () ( Citation37). Galactose and arabinose are the dominating monosaccharides in the ratio of 2.7: 1 as Nadezhda et al. mentioned, “the -COO-, –OH and O existing in the side chains of pectin fragments were involved in the development of silver nanoparticles” ( Citation37). Capping is also facilitated by proteins and enzymes present in the rind extract.
Figure 1. Proposed mechanism for durian rind aqueous extract mediated formation of zinc oxide nanoparticles.
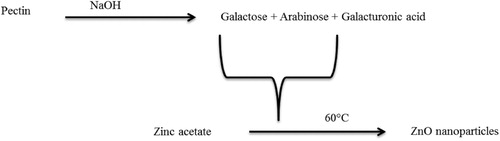
Synthesis of ZnO NPs: process optimization and UV characterization
During exposure to rind extracts, reduction of zinc ions into ZnO NPs was scrutinized as a result of the color change from light yellow to a pale white aqueous solution which occurred due to the SPR phenomenon. The UV-Visible absorption measurements validated the visual observations of the reaction mixture. A characteristic absorption peak of ZnO NPs at 355.5 nm was observed. The current wavelength value is comparable with the values testified by Lingaraju et al. at 355 nm ( Citation38).
As zinc acetate functions as zinc oxide nanoparticles generator in the reaction medium, the zinc acetate concentration in the reaction system indeed affects Zn2+ reduction rate. The UV-Visible spectroscopy () demonstrates the effect of zinc acetate concentration on Zn2+ reduction whereby other reaction parameters were kept unchanged viz. rind extract volume 2.5 mL, stirring time 2 h, pH 12 and temperature 25°C. The intensity of absorption peak was observed to increase with an increase in zinc acetate concentration from 0.01 to 0.03 M, with a decline upon a further increase in zinc acetate concentration from 0.03 to 0.05 M. Moreover, the absorption maximum shifted to shorter wavelength (blue shift) upon increase in zinc acetate concentration from 0.01 to 0.03 M which further shifted to longer wavelength (redshift) upon subsequent increase in zinc acetate concentration from 0.03 to 0.05 M. The observation indicated that the mean diameter of ZnO NPs, decreased upon an increase in zinc acetate concentration up to ≤ 0.03 M and increased when zinc acetate concentration was > 0.03 M. At a higher concentration, the ZnO NPs begin to aggregate and form large particles. According to Nadezhda et al. ( Citation37), a certain part of resulting ZnO does not involve in nanobiocomposites formation and is utilized for the formation of well-formed crystalline structure. In addition, lack of monosaccharides in the reaction medium for reducing zinc acetate to ZnO could also be the reason for the decrease in the intensity of absorbance.
The effect of durian rind extract volume on the reduction of zinc acetate to ZnO nanoparticles revealed that colloidal solution containing 2.5 mL, exhibited substantially higher absorbance values as compared to others, which indicates higher productivity of the method at specified extract volume. However, the absorbance value declines upon a further increase in rind extract concentration probably due to high production of galacturonic acid ( Citation37), which cannot contribute in a redox reaction with zinc acetate to produce ZnO. Therefore, 2.5 mL durian rind extract was selected as the optimum concentration for ZnO NPs synthesis. Our findings are supported by Nadezhda et al. reports, suggesting that the efficiency of nanoparticles synthesis is contingent on reducing sugar concentration produced from pectin by alkali hydrolysis ( Citation37).
Similarly, time, pH and reaction temperature were optimized as per the procedure stated in the volume of rind extract and concentration of zinc optimization. In the present study, the optimized conditions for the ZnO NPs synthesis were 2.5 mL extract of 10% durian rind in deionized water, 0.03 M zinc acetate concentration, 2 h reaction time, pH 12 and temperature 60°C.
shows the characteristic absorption peak of ZnO at 355.5 nm wavelength assigned to intrinsic bandgap absorption of ZnO, owing to electron transitions from the valence band to the conduction band. The bandgap of ZnO NPs was calculated by using Planck's quantum theory and Tauc equation (4) (
Citation39):(4)
(4) where h is the energy of a photon, D is a constant and Eg is bandgap of the material. The transition data offers the best linear fit in the band edge region for n1/2. The observed bandgap of 3.33 eV was slightly lower than the bulk ZnO (3.37) which attributes to the possibility of defects in ZnO crystal lattice. Research (
Citation40) revealed that such defects may induce visible-light absorption pertaining to the isolated states in the forbidden gap of ZnO. The bandgap values difference, 3.0 eV to 3.3 eV, is contemplated as one of the peculiar aspects of ZnO material. This demonstrates purity and good optical properties of synthesized ZnO particles.
Colloidal stability of ZnO NPs in aqueous dispersion
Stability of synthesized ZnO NPs in aqueous dispersion was estimated on the basis of changes in absorption maxima of SPR band for a period of 120 days. The SPR bands persisted essentially symmetrical during the test period. Moreover, surface plasmon absorption peak did not shift significantly, suggesting the absence of aggregation in biosynthesized ZnO NPs during the storage period under ambient conditions.
Characterization of ZnO NPs
The physicochemical characterization, specifically SEM data showed a size range of 122–356 nm with an average size of 280 nm for the synthesized nanoparticles ( (A)). (B) shows the TEM image of ZnO NPs with an average size of 283 nm. SEM and TEM result distinctly confirmed that the majority of nanoparticles were spherical and polydispersed even though the synthesized ZnO particles was bit higher than the accepted range of nanoparticles. (C) displays the EDX spectrum of biosynthesized ZnO NPs, confirming the presence of elemental oxygen and zinc signals. Few tiny weak peaks of P, Ca, Cu and Pt were also observed along with high intense peak due to bounded biomolecules on to the biosynthesized ZnO NPs. Furthermore, the estimated weight percentage of oxygen and zinc was found to be of 22.70% and 60.04%, respectively.
Figure 4. (A) SEM image of ZnO NPs at 400 nm resolution, (B) TEM image of ZnO NPs, (C) XRD pattern of ZnO NPs, (D) EDX spectra of ZnO NPs.
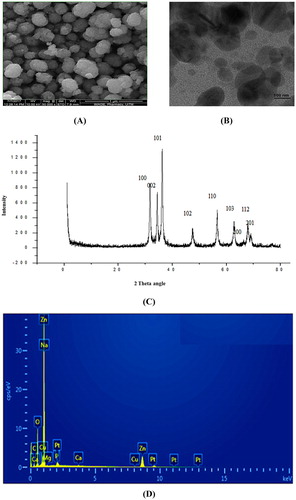
Phase and crystallographic structure of durian rind extract-based ZnO NPs were determined and confirmed with XRD technique whereby ten intense diffraction peaks were evident at 2θ values of 31.12°, 34.42°, 36.22°, 47.44°, 56.62°, 62.78°, 65.44°, 67.94° and 69.42°. The peaks were verified with JCPDS card (No. 5-0664) for a typical wurtzite type polycrystals ( (D)). The presence of [100], [002], [101], [102], [110], [103], [200], [112] and [201] planes in XRD, corroborates formation of pure wurtzite structure of ZnO NPs. Absence of any additional peaks pertaining to impurities indicated high purity of synthesized ZnO NPs.
The hydrodynamic diameters of ZnO NPs were determined with the help of DLS measurement. The mean hydrodynamic diameter of ZnO NPs was observed to be, 456 d.nm, intercept 0.929 in DLS analysis with a low polydispersity index (PDI) of 0.347 ( (A)). The observation of superior average size values in DLS measurement as compared to TEM and SEM analyses could have been due to nanoparticles agglomeration and hydrodynamic radii measurement of NPs by DLS which includes solvent layer at interface and capping. The nanoparticles stability was substantiated by nanoparticles zeta potential which was found to be −14.7 mV ( (B)). The expression of negative potential value by biosynthesized ZnO NPs reflects the presence of bio-organic components in the extract as capping agents.
Figure 5. (A) Size distribution of ZnO NPs obtained from dynamic light scattering, (B) Zeta potential of ZnO NPs.
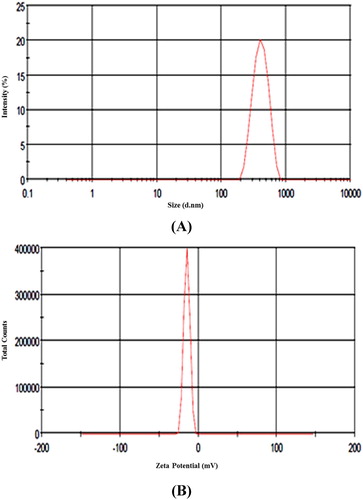
The specific mechanism for the involvement of biomolecules, incorporated in biological extracts, for the synthesis of nanoparticles is yet to be elucidated in detail. Nevertheless, plant-originated polysaccharides and phytochemicals including starch, alginic acid, cellulose, and dextran have been indicated to exhibit dual roles as capping and reducing agents in the synthesis of plant-based nanoparticles ( Citation41).
The confirmation of potential groups responsible for interaction between ZnO nanoparticles and the capping agent was ensured through FTIR (Figure S1). The FTIR spectrum of ZnO NPs expressed sharp intensive peaks at 3586 cm−1 (phenolic OH stretching), 3435 cm−1 (aromatic N-H stretching), 3313 cm−1 (OH stretching of phenol and alcohol), 3170 cm−1 (C-H stretching), 1635 cm−1 (amide I bond of proteins due to carbonyl stretch in proteins), 1513 cm−1 (aromatic ring stretching), 1377 cm−1 (C–C bond of aromatic ring or amide group-II), 1312–1297 cm−1 (aromatic secondary amine CN stretching), 1080 and 1297 cm−1 (stretching vibrations of C-N aromatic and aliphatic amines) and 657 cm−1 (C-H of alkynes). The results inferred that O-H, C-C and C-N vibration stretches in ZnO NPs are from pectin, amino acids and polyphenols of rind extract of durian which, as stabilizing and capping agents, may be involved in ZnO NPs formation.
Antimicrobial activity of ZnO NPs
The accelerating bacterial resistance against antimicrobial agents is considered as the earnest challenge for infectious diseases treatment. Newer bacterial strains with alarming resistance levels have emerged, recently. The necessities of preventive measures against the emergence and spread of multi-resistant bacterial strains and to develop newer effective antimicrobial agents to manage bacterial resistance are eminent than ever.
The current study analyzes antibacterial activities of ZnO NPs against gram-positive bacteria (S. aureus and B. subtilis) and gram-negative bacterium (E. coli) through minimum inhibitory concentration technique. Wherein, no activity against B. subtilis and activity against E. coli and S. aureus were observed. The tube with 500 μg/mL ZnO NPs concentration, with lack of bacterial growth, remained as clear as the negative control; denoting satisfactory inhibitory effectiveness of the concentration against S. aureus and E. coli.
The durian rind extracts based ZnO NPs did not show better antibacterial activities as compared to the ZnO NPs prepared from Emblica officinalis fresh fruit extract ( Citation42). Anbukkarasi et al. reported the highest antibacterial activity for ZnO NPs against E. coli with minimal MIC of 31.25 µg/mL ( Citation42). Moreover, our ZnO NPs did not express any antimicrobial activity against B. subtilis, whereas, the biosynthesized Brassica oleraceae leaves extract-based ZnO NPs have indicated MIC of 50 µg/mL ( Citation43).
Additionally, the synergistic effect of ZnO NPs (1 mg/mL) on the antibacterial activity of penicillin and ampicillin was also determined by zone of inhibition technique against S. aureus and E. coli, respectively. Thangham et al. reported the same results where the maximum zone of inhibition was formed at the highest concentration of ZnO NPs ( Citation44). A significant escalation in the zone of inhibition against S. aureus was also observed in the case of the combination of ZnO NPs and penicillin which was observed to be of 21 mm, even more than the zone of inhibition of penicillin alone i.e. 17 mm (). The synergistic effect, however, is considered strong as compared to the study of antimicrobial activity of zinc oxide, titanium dioxide and silver nanoparticles against S. aureus isolates ( Citation45).
Table 1. Antimicrobial activities of ZnO NPs.
The standard ampicillin exhibited a zone of inhibition of 20 mm () against E. coli indicating significant inhibitory activity of the molecule. However, the combined effect of ZnO NPs with ampicillin against E. coli was observed to be equal as compared to the antibiotic alone. Nazari et al. reported similar results of gold nanoparticles (Au NPs) combined with 14 β-lactam antibiotics against Staphylococcus aureus, Pseudomonas aeruginosa, and E. coli which highlighted no enhancement in antibacterial activities of antibiotics due to Au NPs at the tested concentrations ( Citation46).
The present study results indicated higher effectiveness of ZnO NPs against Gram-positive bacteria as compared to the Gram-negative bacteria. The probable cause of the variation might be due to cell wall dissimilarities between Gram-positive and Gram-negative bacteria which provide more resistance against antibacterial agents to the later.
Several studies have been conducted to ascertain the mechanism behind the antibacterial activity of ZnO NPs. Zhang et al. suggested direct contact of zinc oxide nanoparticles with bacterial cell walls leads to detrimental effects on bacterial cell integrity ( Citation47). Moreover, the liberation of antimicrobial ions mainly Zn2+ ions and formation of reactive oxygen species (ROS) such as hydrogen peroxide and superoxide ions have also been proposed as the mechanism of ZnO NPs antibacterial activities ( Citation48). However, the exact toxicity mechanism is yet to be fully understood and is still considered controversial. Peng et al. expressed that the larger surface area and higher concentration are also responsible for antibacterial activities of ZnO NPs ( Citation49).
Antioxidant activity
The DPPH assay indicated that the free radical scavenging activities of ZnO NPs on DPPH radicals increased with an increase in concentration and the maximum antioxidant activity of 78.19% was reported at 10 mg/mL, as displayed in . Moreover, the assay also demonstrated the potent radical scavenger property of ZnO NPs with an IC50 value of 6.39 mg/mL (). The functional groups (pectin) adhered to the ZnO NPs could have attributed to the exhibited antioxidant ability. The natural compound pectin has been recognized for its antioxidant activity ( Citation36). It has been reported that such extracts efficiently yield metal oxide or metal nanoparticles upon utilization for reduction of metal acetates or nitrates. However, Lingaraju et al. reported that the IC50 value of green synthesized zinc oxide nanoparticles from Ruta graveolens, was 9.34 mg/mL ( Citation38). Our studies results are found to be better than the above mentioned studies which could be due to the presence of capping agent on synthesized ZnO NPs. Interestingly, Nagajyothi et al. described that the green synthesized ZnO NPs using Polygala tenuifolia root extract, showed 45.47% free radical scavenging activity on DPPH radicals with 1 mg/mL concentration ( Citation50), better than our present study results. The reported superior antioxidant activity could have been due to the presence of quercetin, active constituent of Polygala tenuifolia root, over the surface of zinc oxide nanoparticles.
Photocatalytic degradation of methylene blue
The dye degradation of MB under sunlight irradiation evaluates the photocatalytic activities of biosynthesized ZnO NPs. The gradual change in solution color from deep blue to colorless indicates dye degradation. The characteristic absorption peak at 662 nm was observed for pure methylene blue dye solution which was utilized to trace the photocatalytic degradation process of MB. The dye degradation in the presence of biosynthesized ZnO NPs was observed visually and verified by the decrease of the peak intensity spectrophotometrically at 662 nm during 40 min exposure in solar light. The degradation of MB under solar irradiation was determined in different conditions such as in absence of ZnO NPs with pH 10, and in presence of ZnO NPs with pH 10 ( (A) and 7 (B)). The degradation of MB under solar irradiation in the presence of ZnO NPs at pH 7 was studied from 0 min to 180 min at an interval of 30 min. Since the degradation efficiency was less in the condition, hence pH 10 was selected as optimum pH for the degradation of MB. The photocatalytic degradation efficiency of ZnO NPs and dye degradation (%) variant with sunlight exposure time is graphically represented in (A) and 8 (B), respectively.
Figure 7. Degradation of methylene blue under solar irradiation: (A) in absence of ZnO NPs at pH 10, (B) in the presence of ZnO NPs at pH 10.
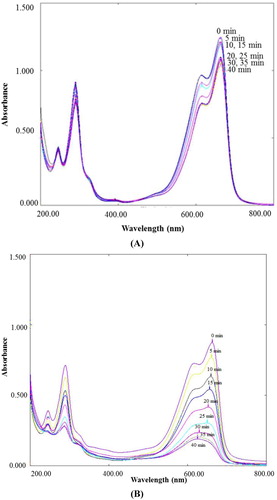
Figure 8. (A) Photocatalytic degradation efficiency of ZnO NPs, (B) Percentage degradation of methylene blue in the presence and absence of ZnO NPs under solar irradiation.
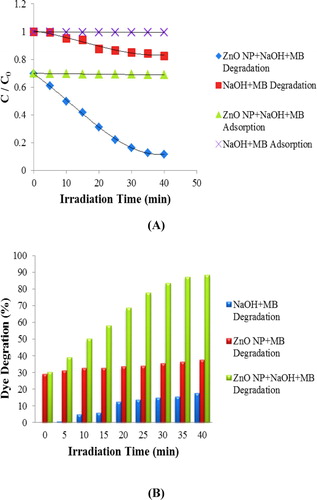
It is evident that the characteristic absorption peak of MB solution containing ZnO NPs with pH 10 at 662 nm, decreases rapidly with an increase in irradiation time ( (A)) with a dye degradation of 84% at 40 min, as shown in (B). In contrast, only 18% and 38% degradation of MB was observed for solar light irradiated solutions containing MB with pH 10 ( (B)), and MB and ZnO NPs with pH 7 ( (B)), respectively. The results revealed that the synthesized ZnO NPs are promising degrading agents of MB which may be used for water treatment.
Interestingly, our present study result is comparable with the conclusions of Anbuvannan et al. who have indicated a rapid decline in absorbance to about zero for MB solution in presence of Phyllanthus niruri leaves extract mediated zinc oxide nanoparticles at 664 nm within 30 min, under UV irradiation ( Citation51). However, our findings are comparatively better than the results reported by Parthiban and Sundaramurthy in which the MB dye degradation using Pyrus pyrifolia leaf extract based ZnO NPs showed declined peak intensity to zero at 657 nm within 210 min, under solar light exposure ( Citation52).
In the photocatalytic decomposition process, generally, the dye pollutant degradation occurs due to the photo-generation of electron-hole pair between valence bands and conduction. Either H2O or OH− reacts with photogenerated h+ in the valence band to produce the HO.. Whereas, e− in the conduction band, reacts with adsorbed O2 on ZnO surface to produce O2− and HO radicals (
Citation5). Consequently, the dye is decomposed by the produced radicals (
Citation53).(5)
(5) The other possible reason for the degradation of MB is that it accepts electrons and degrades into leucomethylene blue (LMB), which is colorless since MB is electroactive and is capable of accepting electrons (
Citation54).
To evaluate the photostability of the catalyst, recycling experiments for the photodegradation of MB were performed. We observed that 84% of the MB was degraded upon first ZnO NPs use. However, after three recycles its photocatalytic activity was decreased slightly (second run 82.34%, third run 81.45%). We also imply that the particle size of ZnO NPs could have an important role in the photocatalytic activity.
Photocatalytic degradation of sulfanilamide under natural sunlight
The calibration curve for sulphanilamide in tap water is shown in . The degradation of sulphanilamide in-room atmosphere was also executed and there was negligible degradation of sulfanilamide at pH 7 and 10. In the dark condition, there was only 3.02% and 1.69% of sulfanilamide degradation in 5 h at pH 7 and 10, respectively. Therefore, the degradation of sulfanilamide under natural sunlight was studied.
shows that at 5 h, there were 51.29%, 55.59%, 57.00%, 45.49%, and 37.18% degradation of sulfanilamide at pH 7, 9, 10, 11 and 12, respectively. The percentage degradation of sulfanilamide was observed to be the highest at pH 10 and lowest at pH 12. The percentage degradation of sulfanilamide in alkaline conditions followed the order of pH 10 > pH 9 > pH 7 > pH 11 > pH 12. However, the degradation of sulfanilamide in the presence of H2O2 and acetone under natural sunlight did not exhibit significant results.
Figure 10. Percentage degradation of sulfanilamide at pH 7, 9, 10, 11and 12 in different time interval under natural sunlight.
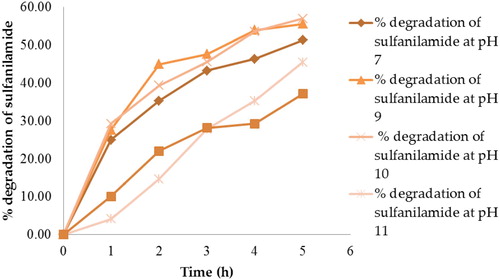
In our present study, it was found that 51.29% of sulfanilamide () was degraded under natural sunlight in 5 h. In comparison to other studies ( Citation55), a prominent result in the degradation of sulfanilamide under natural sunlight without any photocatalyst was observed. The Sun emitted around 43.00% visible light, 49.00% near-infrared region, 7.00% UV ray, and < 1.00% are x-ray, gamma wave and radio waves ( Citation56). Hence, it can be deduced that natural sunlight can degrade sulfanilamide as the sun emits UV rays, however, the light intensity may affect the degradation.
In the present study, we identified that ZnO NPs can act as photocatalyst for the degradation of sulfanilamide under natural sunlight. and indicate that ∼96.70% of sulfanilamide was degraded in the presence of 0.10% ZnO NPs in 3 h. The degradation of sulfanilamide with OH radical proceeds via breaking of -N-C-, -S-N- and -C-S- bonds with loss of NH4+, NO3− and SO42− ions and hydroxylation of the benzene ring forming aromatic intermediates ( Citation57).
Figure 11. UV-Vis spectra of degradation of sulfanilamide at different time interval in the presence of 0.10% ZnO NPs under natural sunlight.
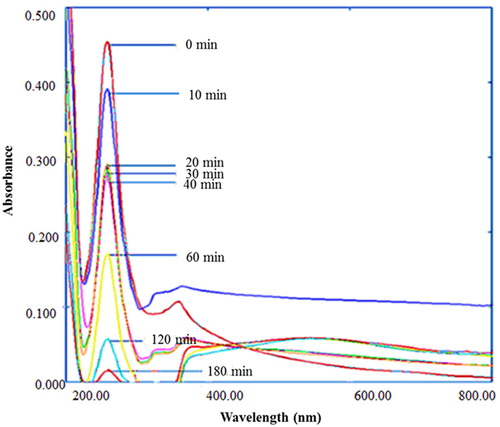
Figure 12. Percentage degradation of sulfanilamide at different time interval in the presence of 0.10% ZnO NPs under natural sunlight.
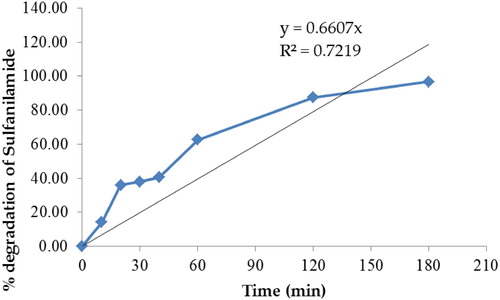
Dong et al. reported that the degradation efficiency of sulfanilamide under natural sunlight irradiation with BiOCl/RGO composite (1.00% RGO amount) as photocatalyst was 82.70% in 5 h ( Citation55). As compared to this, ZnO NPs might be a better photocatalyst for the sulfanilamide degradation under natural sunlight since 96.70% of sulfanilamide is degraded in 3 h. Dong et al. employed A6.26 (dumbbell-shaped A-BiVO4 with uniform size distribution at pH 6.26) and S6.26 (olive-shaped S-BiVO4 with uniform size distribution at pH 6.26) as photocatalysts in the degradation of sulfanilamide under simulated sunlight for 1 h. The degradation efficiency of sulfanilamide were 57.90% and 53.60% in presence of S6.26 and A6.26, respectively ( Citation58). However, in our study, the experiment was performed under natural sunlight as compared to the simulated sunlight unlikely to Dong et al. ( Citation58). Moreover, at 1 h, the comparative degradation efficiency was 62.64% in the case of our study which is slightly higher than the reported study.
indicates that in the presence of ZnO NPs, the degradation of sulfanilamide increased significantly as compared to the degradation of sulfanilamide alone without any photocatalyst at pH 10 under natural sunlight. Therefore, we propose that ZnO NPs are efficient photocatalysts for the degradation of sulfanilamide.
Figure 13. Percentage degradation of sulfanilamide at different time interval in the presence and absence of 0.10% ZnO NPs under natural sunlight.
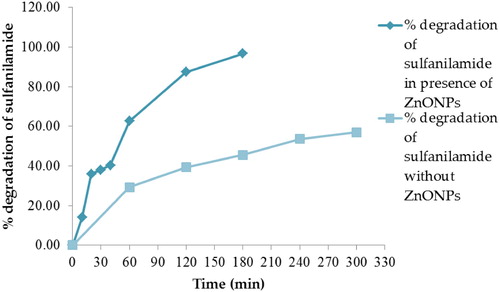
To evaluate the photostability and reusability of the catalyst, recycling experiments for the photodegradation of sulfanilamide were performed. We observed that 96.70% of the sulfanilamide was degraded upon first ZnO NPs use. However, after three recycles photocatalytic activity decreased significantly (second run 90.20%, third run 80.15%).
Cytotoxicity against Artemia salina
The commonly used feeds for larval fish cultures are brine shrimp, Artemia species, zooplankton such as daphnids and copepods which are vital for the flow of energy across the food chain in the marine environment ( Citation59). Artemia species have been utilized for assessing the acute toxicities of various substances including pesticides and heavy metals ( Citation55,Citation56). An extensive spectrum of Artemia-based standard bioassays has been developed due to the distinctive advantages of Artemia, viz., cost-effectiveness, year-round availability, shorter life-cycle, ease of culture development, high offspring production rate and lack of necessity to feed during the assay ( Citation60).
illustrates the mortality rates (cytotoxic effect) of ZnO NPs which indicates that the controls showed ∼ 3% mortality in 24 h. The brine exposures to ZnO NPs were executed in the absence of food. Control groups clearly demonstrated the experimental mortalities suggesting that the food deprivation did not prompt lethal effects. The also indicates that the LC50 value of synthesized colloidal ZnO NPs was observed to be of 0.889 mg/mL, whereas, the highest mortality of 76.75% was observed at 1.4 mg/mL. Therefore, the findings suggest that the concentration of ZnO NPs directly proportionated the lethality. Similarly, Soniya et al. reported the lethality for brine shrimp by using ZnO NPs synthesized by thermochemical method, where LC50 was ∼ 0.4 mg/mL at 24 h with the lethality of 81% at 48 h ( Citation61). In another study, Bhuvaneshwari et al. described the lethality for brine shrimp by using purchased ZnO NPs, where LC50 was ∼ 0.027 mg/mL ( Citation62) which is comparatively more toxic than our synthesized ZnO NPs and the reported ZnO NPs by Soniya et al. ( Citation61). Analogous to our findings, Ates et al. also reported that the LC50 value of purchased ZnO NPs was > 100 mg/mL, and the mortality rate was dependent on the concentration and time of interaction ( Citation63).
Conclusion
Durian rind aqueous extract based ZnO NPs were successfully synthesized, which not only exhibited antibacterial activities against human pathogens but have also demonstrated superior free-radical scavenging activities. The photocatalytic study indicated the conclusive efficiency of ZnO NPs to degrade methylene blue dye at pH 10, under solar irradiation. It has distinctly demonstrated that ZnO NPs possess superior photocatalytic activity as 96.70% of sulfanilamide was successfully degraded under natural sunlight in 3 h. The synthesized ZnO NPs were also not found to be toxic as the LC50 value of the synthesized colloidal ZnO NPs was found to be of 0.889 mg/mL against brine shrimp. Therefore, these can be explored certainly in the fields of textile industries, water purification, and wastewater management. Our findings strongly advocate that the plant-mediated NPs can categorically be used not only against the human pathogens as efficient therapeutic agents but also for the treatment of free radical generated diseases and for wastewater purification, in near future.
Supplemental Material
Download MS Word (183.4 KB)Acknowledgements
Authors are pleased to acknowledge AIMST University for financial assistance, AIMST University and Universiti Teknologi MARA, Malaysia for providing the necessary facilities to carry out this work.
Disclosure statement
No potential conflict of interest was reported by the author(s).
Notes on contributors
Sumitha Samuggam is pursuing her Ph.D. at Faculty of Applied Science, AIMST University, Malaysia. She did her BSc and MSc in the same University.
Cheah Yi Ning is a research student at Faculty of Pharmacy, AIMST University, Malaysia.
Ooi Yi Xian is a research student at Faculty of Pharmacy, AIMST University, Malaysia.
Ung Kiew Yu is a research student at Faculty of Pharmacy, AIMST University, Malaysia.
Syed Adnan Ali Shah is working at Atta-ur-Rahman Institute for Natural Products Discovery, Universiti Teknologi MARA, Puncak Alam Campus, 42300 Bandar Puncak Alam, Selangor Darul Ehsan, Malaysia. He has a number of publications in his credit.
Minaketan Tripathy served as senior lecturer at Faculty of Pharmacy, Universiti Teknologi MARA, Puncak Alam Campus, 42300 Bandar Puncak Alam, Selangor Darul Ehsan, Malaysia in between March 2008 and March 2019. He has many publications in his credit. He is presently working as Professor of Pharmaceutics of Adichunchanagiri University, B.G.Nagara-571448, Karnataka, India.
Neeraj Paliwal is a Lecturer in Pharmacy at Faculty of Pharmacy AIMST University, Malaysia. He has a diverse history of publications and conferences.
Veerasamy Ravichandran is a Professor of Pharmaceutical Chemistry at Faculty of Pharmacy, AIMST University, Malaysia. Head, Unit of Pharmaceutical Chemistry. His area of interest is the green synthesis of nanoparticles, synthesis and biomedical evaluation of organic compounds and molecular modeling. He has more than 125 publications in his credit.
References
- Kim, J.S.; Kuk, E.; Yu, K.N.; Kim, J.H.; Park, S.J.; Lee, H.J.; Kim, S.H.; Park, Y.K.; Hwang, C.Y.; Kim, Y.K. Nanomed. Nanotechnol. Biol. Med. 2007, 3, 95–101. doi: 10.1016/j.nano.2006.12.001
- Mukunthan, K.S.; Elumalai, E.K.; Patel, T.N.; Ramachandra Murty, F.V. Asian. Pac. J. Trop. Biomed. 2011, 1, 270–274. doi: 10.1016/S2221-1691(11)60041-5
- Saifuddin, N.; Wong, A.A.; Yasumira, N. E-J. Chem. 2009, 6, 61–70. doi: 10.1155/2009/734264
- Buazar, F.; Baghlani-Nejazd, M.H.; Badri, M.; Kashisaz, M.; Khaledi-Nasab, A. Starch - Stärke 2016, 68, 796–804. doi: 10.1002/star.201500347
- Buazar, F.; Bavi, M.; Kroushawi, F.; Halvani, M.; Khaledi-Nasab, A.; Hossieni, S.A. J. Exp. Nanosci. 2016, 11 (3), 175–184. doi: 10.1080/17458080.2015.1039610
- Tien, H.N.; Luan, V.H.; Hoa, L.T.; Khoa, N.T.; Hahn, S.H.; Chung, J.S.; Shin, E.W.; Hur, S.H. Chem. Eng. J. 2013, 229, 126–133. doi: 10.1016/j.cej.2013.05.110
- Chao, L.C.; Chiang, P.C.; Yang, S.H.; Huang, J.W.; Liau, C.C.; Chen, J.S.; Su, C.Y. Appl. Phys. Lett. 2006, 88, 251111–251113. doi: 10.1063/1.2214146
- Li, B.; Liu, T.; Wang, Y.; Wang, Z. J. Colloid Interf. Sci 2012, 37, 7114–7121.
- Hu, S.H.; Chen, Y.C.; Hwang, C.C.; Peng, C.H.; Gong, D.C. J. Alloys Compd. 2010, 500, L17–L21. doi: 10.1016/j.jallcom.2010.03.235
- Moharram, A.H.; Mansour, S.A.; Hussein, M.A.; Rashad, M. J. Nanomat 2014, Article ID 716210.
- Manokari, M.; Shekhawat, M.S. WSN 2016, 47, 267–278.
- Yedurkar, S.; Maurya, C.; Mahanwar, P. Open J. Syn. Theory App 2016, 25, 1–14.
- Al-Dhabi, N.A.; Arasu, M.A. Nanomaterials 2018, 8, 500. doi: 10.3390/nano8070500
- Padalia, H.; Chanda, S. Artif. Cells. Nanomed. Biotechnol. 2017, 45, 1751–1761. doi: 10.1080/21691401.2017.1282868
- Khalafi, T.; Buazar, F.; Ghanemi, K. Sci. Rep. 2019, 9 (1), 6866. doi: 10.1038/s41598-019-43368-3
- Pati, R.; Mehta, R.K.; Mohanty, S.; Goswami, C.; Sonawane, A. Nanomed. Nanotechnol. Biol. Med. 2014, 10, 1195–1208. doi: 10.1016/j.nano.2014.02.012
- Shamsuzzaman, A.; Mashrai, H.; Khanam, R.N.; Aljawfi, R.N. Arab. J. Chem 2013, 10S, 1530–S1536.
- Shanmugasundaram, T.; Balagurunathan, R. Artif. Cells. Nanomed. Biotechnol. 2017, 45, 1521–1529. doi: 10.1080/21691401.2016.1260577
- Tripathi, R.M.; Bhadwal, A.S.; Gupta, R.K.; Singh, P.; Shrivastav, A.; Shrivastav, B.R. J. Photochem. Photobiol., B 2014, 141, 288–295. doi: 10.1016/j.jphotobiol.2014.10.001
- Singh, B.N.; Rawat, A.K.S.; Khan, W.; Naqvi, A.H.; Singh, B.R. PLOS ONE 2014, 9, e106937. doi: 10.1371/journal.pone.0106937
- Hasnidawani, J.N.; Azlina, H.N.; Norita, H.; Bonnia, N.N.; Ratim, S.; Ali, E.S. Procedia. Chem. 2016, 19, 211–216. doi: 10.1016/j.proche.2016.03.095
- Ismail, L.F.M.; Emara, M.M.; El-Moselhy, M.M.; Maziad, N.A.; Hussein, O.K. Spectrochim. Acta, Part A 2014, 131, 158–168. doi: 10.1016/j.saa.2014.03.041
- Atrak, K.; Ramazania, A.; Fardood, S.T. J. Photochem. Photobiol., A 2019, 382, 111942. doi: 10.1016/j.jphotochem.2019.111942
- Ghotekar, S. Asian J. Green Chem 2019, 3, 187–200.
- Powara, N.S.; Patelb, V.J.; Pagarec, P.K.; Pandavd, R.S. Chem. Methodol. 2019, 3, 457–480.
- Sorbiun, M.; Mehr, E.S.; Ramazani, A.; Fardood, S.D. J. Mater. Sci.: Mater. Electron. 2018, 29 (4), 2806–2814.
- Arancibia-Avila, P.; Toledo, F.; Park, Y.S.; Jung, S.T.; Kang, S.G.; Heo, B.G. LWT - Food Sci. Technol. 2008, 41, 2118–2125. doi: 10.1016/j.lwt.2007.12.001
- Leontowicz, H.; Leontowicz, M.; Haruenkit, R.; Poovarodom, S.; Jastrzebski, Z. Food Chem. Toxicol. 2008, 46, 581–589. doi: 10.1016/j.fct.2007.08.042
- Jaswir, I.; Man, Y.B.C.; Selamat, J.; Ahmad, F.; Sugisawa, H. J. Food Process. Preserv. 2008, 32, 740–750. doi: 10.1111/j.1745-4549.2008.00211.x
- Zahran, M.K.; Ahmed, H.B.; El-Rafie, M.H. Carbohydr. Polym. 2014, 111, 971–978. doi: 10.1016/j.carbpol.2014.05.028
- Veerasamy, R.; Xin, T.Z.; Gunasagaran, S.; Xiang, T.F.W.; Yang, E.F.C.; Jeyakumar, N.; Dhanaraj, S.A. J. Saudi Chem. Soc. 2011, 15, 113–120. doi: 10.1016/j.jscs.2010.06.004
- Ravichandran, V.; Shalini, S.; Vasanthi, S.; Shaa, S.A.A.; Harish, R. Mater. Lett. 2016, 180, 264–267. doi: 10.1016/j.matlet.2016.05.172
- Brand-Williams, W.; Cuvelier, M.E.; Berset, C. Food Sci. Tech 1995, 28, 25–30.
- Sahu, M.K.; Patel, R.K. J. Ind. Eng. Chem. 2016, 40, 72–82. doi: 10.1016/j.jiec.2016.06.008
- Prakash, S.; Ahila, N.K.; Sri Ramkumar, V.; Ravindran, J.; Kannapiran, E. Biocatal. Agric. Biotechnol. 2015, 4, 114–121. doi: 10.1016/j.bcab.2014.11.002
- Vasily, V.V.; Victoria, V.G.; Fedor, V.V.; Olga, A.P.; Nikolay, Y.S.; Olga, G.S.; Sergey, V.P. J. Chem 2017, ID.5898594.
- Nadezhda, V.I.; Natalya, N.T.; Lydmila, A.E.; Vasilyi, A.B. Int. J. Carbohydrate Chem 2012, ID 459410.
- Lingaraju, K.; Raja Naika, H.; Manjunath, K.; Basavaraj, R.B.; Nagabhushana, H.; Nagaraju, G.; Suresh, D. Appl. Nanosci. 2016, 6, 703–710. doi: 10.1007/s13204-015-0487-6
- Khorsand Zak, A.; Razali, R.; Majid, W.H.A.; Darroudi, M. Int. J. Nanomed 2011, 61, 399–1403.
- Tang, Y.; Zhou, H.; Zhang, K.; Ding, J.; Fan, T.; Zhang, D. Chem. Eng. J. 2015, 262, 260–267. doi: 10.1016/j.cej.2014.09.095
- Park, Y. Toxicol. Res. 2014, 30, 169–178. doi: 10.5487/TR.2014.30.3.169
- Anbukkarasi, V.; Srinivasan, R.; Elangovan, N. Int. J. Pharm. Sci. Rev. Res 2015, 33 (2), 110–115.
- Raj, A.; Lawrence, R.S.; Jalees, M.; Lawrence, K. Int. J. Adv. Res 2015, 3 (11), 322–328.
- Thangam, A.; Pritam, S.; Ramlakshmi, S. Asian J. Pharm. Clin. Res 2014, 7, 202–206.
- Abdulrahman, N.B.; Nssaif, Z.M. Tikrit J. Pure Sci 2016, 21 (3), 49–53.
- Nazari, Z.E.; Banoee, M.; Sepahi, A.A.; Rafii, F.; Shahverdi, A.R. Gold. Bull. 2012, 45, 53–59. doi: 10.1007/s13404-012-0048-7
- Zhang, L.; Jiang, Y.; Ding, Y.; Povey, M.; York, D. J. Nanopart. Res. 2007, 9, 479–489. doi: 10.1007/s11051-006-9150-1
- Li, M.; Zhu, L.; Lin, D. Environ. Sci. Technol. 2011, 45 (5), 1977–1983. doi: 10.1021/es102624t
- Peng, X.; Palma, S.; Fisher, N.S.; Wong, S.S. Aquat. Toxicol. 2011, 102 (3), 186–196. doi: 10.1016/j.aquatox.2011.01.014
- Nagajyothi, P.C.; JuCha, S.; Yang, I.J.; Sreekanth, T.V.M.; MookShin, K.J.K. J. Photochem. Photobiol., B 2015, 146, 10–17. doi: 10.1016/j.jphotobiol.2015.02.008
- Anbuvannan, M.; Ramesh, M.; Viruthagiri, G.; Shanmugam, N.; Kannadasan, N. Spectrochim. Acta, Part A 2015, 143, 304–308. doi: 10.1016/j.saa.2015.01.124
- Parthiban, C.; Sundaramurthy, N. Int. J. Innovative Res. Sci. Eng. Technol 2015, 4 (10), 9710–9718.
- Wu, T.X.; Liu, G.M.; Zhao, J.C.; Serpone, H.H.N. J. Phys. Chem. B 1998, 102, 5845–5851. doi: 10.1021/jp980922c
- Lam, S.M.; Sin, J.C.; Abdullah, A.Z.; Mohamed, A.R. Desalin. Water Treat. 2012, 41, 131–169. doi: 10.1080/19443994.2012.664698
- Dong, S.; Pi, Y.; Li, Q.; Hu, L.; Li, Y.; Han, X. J. Alloys Compd. 2016, 663, 1–9. doi: 10.1016/j.jallcom.2015.12.027
- National Center for Atmospheric Research. Radiation and Albedo Experiment [Internet]. Boulder: National Center for Atmospheric Research; 2018 [cited, 2018 May 7]. Available from: https://scied.ucar.edu/activity/learn/radiation-albedo.
- Liu, Y.; Wang, J. J. Hazard. Mater. 2013, 250-251, 99–105. doi: 10.1016/j.jhazmat.2013.01.050
- Dong, S.; Feng, J.; Li, Y.; Hu, L.; Liu, M.; Wang, Y. Appl. Catal., B 2014, 152-153 (1), 413–424. doi: 10.1016/j.apcatb.2014.01.059
- Sorgeloos, P. Mar. Ecol. Prog. Ser. 1980, 3, 363–364. doi: 10.3354/meps003363
- Kokkali, V.; Katramados, I.; Newman, J.D. Biosensors. 2011, 1, 36–45. doi: 10.3390/bios1020036
- Soniya, P.; Kumaresn, K.; Parthiban, D.; Arun, N.; Kumaravel, P. Res. J. Pharm. Dosage Forms Technol. 2015, 7 (2), 103–110. doi: 10.5958/0975-4377.2015.00015.4
- Bhuvaneshwari, M.; Sagar, B.; Doshi, S.; Chandrasekaran, N.; Mukherjee, A. Environ.Sci. Pollut. Res. 2017, 24 (6), 5633–5646. doi: 10.1007/s11356-016-8328-z
- Ates, M.; Daniels, J.; Arslan, Z.; Farah, I.O.; Rivera, H.F. Environ. Sci.: Processes Impacts 2013, 15, 225–233.