ABSTRACT
The group bacilli represent the Gram-positive ubiquitous spore-forming bacteria. Their diversity, versatility and the ability of producing diverse secondary metabolites including enzymes created enormous potential for applications in agriculture, biotechnology, environment and medicine. The bacilli are considered as one of the most studied groups of bacteria providing plant growth-promotion and biocontrol of multiple diseases reflecting their vital role in enhancing plants’ tolerance to biotic and abiotic stresses. Some of the Bacillus species are available commercially as phytostimulants, biopesticides, and biofertilizers. Genetically engineered plants such as maize, cotton, brinjal with endotoxins producing genes from Bacillus thuringiensis (Bt) has revolutionized agriculture. Many of these applications have been widely adopted in various fields as viable and environmentally friendly alternatives of synthetic chemical fertilizers and pesticides. A better understanding of the biology, ecology, and mechanisms of action of the beneficial strains of bacilli are needed for the development of products to support green biotechnology in agriculture and industries. This report comprehensively reviewed the applications of bacilli in agriculture and industry and discussed their potentials for the development of new products of biotechnological implications.
GRAPHICAL ABSTRACT
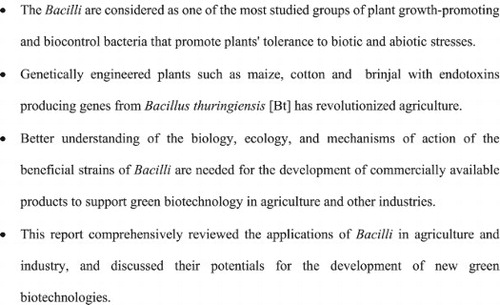
1. Introduction
Bacillus species are widely distributed in a wide range of environmental (including extreme) conditions such as water, dead insects, plants, soil, marine environment, the gut of animals and food samples (Citation1, Citation2). They are aerobic endospore-forming bacteria that can survive in the harshest climatic and edaphic conditions. Members of the genus Bacillus produce a vast array of bioactive metabolites that have potential applications in agriculture, fisheries, medical, and veterinary sciences (Citation3–5). In addition, Bacillus-produced enzymes, antibiotics and other metabolites have been utilized in medical, agricultural and pharmaceutical industires (Citation5). Mass production of metabolites such as cyclicpeptides (Citation6), polyketides (Citation7), and bacteriocin (Citation8) by the species of Bacillus is an attractive area of research with enormous potential of commercialization (Citation4). One of the promising applications of bacilli in agricultural sector is their use as a biological control agent of biotic diseases (Citation9, Citation10). Bacillus alone is estimated to account for half of the commercially available bacteria-based biological control agents. They belong to one of the most studied microorganisms that have been used as antagonists of phytopathogenic bacteria, fungi, oomycetes, nematodes, and insects (Citation10, Citation11). Currently, there is an urgent need for intensifying agricultural production to keep pace with the rapid increase of global population. This elevated production target compelled agricultural community to use synthetic products such as fertlizers and pesticices making the whole agro-ecosystem more unsustainable than ever. Therefore, there are growing appeals for the use of environmentally safe and sustainable pest control agents such as Bacillus spp. to support ‘Zero hunger’ the second most important sustainable development goal (SDG) set by the UNO. In this regard, several strains of the genus Bacillus have demonstrated the potential to be used as plant growth-promoting bacteria (PGPB) and proved to have antagonistic activities against several phytopathogenic microorganisms (Citation12).
According to estimates of the Food and Agriculture Organization (FAO), the world population would reach 9.7 billion by the year 2050 (Citation13). This necessitates substantial increase in agricultural production to feed a population of such magnitude. Besides, climate change is also posing a challenge to worldwide crop production. Much of the arable land may no longer be usable due to increase in salinity, drought or pest attack. These problems can’t be solved by the use of chemicals. Therefore, there is an urgent need to look for a sustainable way to maximize agricultural production. In this regard, the role of Bacillus species in controlling plant pathogens, enhancing their tolerance to various biotic and abiotic stresses thereby increasing production would be an exciting area of research. T he discovery of the elite strains of Bacillus spp. has high potentials for commercialization and management of abiotic and biotic stresses to improve crop production. Recent advances in genomics and postgenomics analyses have shed light on the molecular mechanisms of biocontrol of plant diseases by the Bacillus species (Citation14, Citation140). Insecticidal toxins produced in commercially available transgenic plants is originated from the soil bacterium Bacillus thuringiensis (Bt). Although Bt strains show differing specificities of insecticidal activity toward pests, they constitute a large reservoir of genes encoding insecticidal proteins, which are accumulated in the crystalline inclusion bodies produced by the bacterium on sporulation (Cry proteins, Cyt proteins) or expressed during bacterial growth (Vip proteins)(Citation15). Development of insect-resistant crops is one of the major successes of plant genetic engineering technology by inserting B. thuringiensis genes in many agriculturally important crops. Some of the success stories of Bt technology include cotton (Gossypium hirsutum) resistant to lepidopteran larvae (caterpillars) and maize (Zea mays) resistant to both lepidopteran and coleopteran larvae (rootworms) that have been widely used in global agriculture resulting in reductions in pesticide usage and lower production costs (Citation16, Citation17). A recently introduced Bt-brinjal in Bangladesh dramatically reduced synthetic pesticide application and increased farmers’ income (Citation18). However, the use of Bt GM crops comes with various challenges that are to be addressed. Some insects have developed resistance to Bt GM crops creating new economic or agronomic challenges. Additional scientific efforts were made to stack Bt genes in certain crops or new agronomic practices were introduced to prevent insects from developing resistance. For example, farmers need to plant a certain amount of conventional plants alongside Bt plants in ‘refuge’ areas. Several reviews have been published on bioactive compounds from terrestrial and marine bacilli, their biosynthetic pathways and engineering plants using Bt genes (Citation4, Citation14, Citation15, Citation19–24). However, there is no comprehensive review published on baccili as source of agrobiotechnology. This comprehensive review updates our understanding of the applications of bacilli in agriculture and industry, and discusses their promise for the development of new agrobiotechnology.
2. Bacillus spp. as biocontrol agents
2.1. Bacillus spp. as antagonists of phytopathogens
Phytopathogens pose a great challenge to world food security (Citation25, Citation11, Citation10). They diminish the annual yield by a significant amount that could otherwise feed millions of people (Citation25). Thus, they cause huge economic losses to both developing and developed economies. Various studies have established the potential use of Bacillus spp. in controlling plant pathogens (Citation26, Citation27). Antagonistic activities of these bacilli take place primarily through the production and secretion of various bioactive secondary metabolites (Citation11, Citation14, Citation28–32) and lytic enzymes (Citation33–36). Verschuere et al. (Citation37) argued that among bacteria-based antagonists, B. subtilis is one of the most studied and used bacteria with a wide range of activities against phytopathogens. On the other hand, B. thuringiensis (Bt) accounts for over 90% of all marketed biological control agents with millions of dollars of annual sales.
The emergence of fungal diseases in plants increased remarkably in the last few decades, and they are posing a serious threat to future food and nutritional security (Citation11, Citation38). From many years of research, a large body of literature is now available on the biological activities of the bacilli against fungal and other phytopathogens. A strain of B. subtilis has shown more than 80% suppression of Rhizoctonia solani, the causative agent of damping-off of tomato seedlings (Citation39). In a similar study, Ji et al. (Citation40) isolated a strain of B. amyloliquefaciens from the Republic of Korea and tested the strain against various fungal plant diseases. It displayed activities both in laboratory and greenhouse settings against cucumber scleotiorum rot. It also showed antagonistic activity in laboratory setting against tomato gray mold and powdery mildew disease in cucumber. Similarly, a strain B. velezensis isolated by Toral et al. (Citation41) demonstrated the potential for protecting tomatoes, grapes, and strawberries from various phytopathogens including B. cinerea. The strain produces various lipopeptides with activities against B. cinerea and has the potential to trigger an antioxidant activity in fruit. Novel linear lipopeptides produced by a marine B. subtilis strain 109GGC020 significantly suppressed oomycete phytopathogens (Citation42).
The filamentous fungus, Fusarium graminearum causes Fusarium head blight (FHB) on wheat and barley leading to massive economic loss to the growers (Citation43). It is also known to cause seedling blight on maize. Gu et al. (Citation44) investigated a strain of B. amyloliquefaciens’s potential to suppress F. graminearum. Results from their study showed that the strain produces bacillomycin D, which causes morphological changes in the plasma membrane and cell wall of F. graminearum, induces accumulation of reactive oxygen species, and ultimately causes cell death in the pathogen. Araujo et al. (Citation45) isolated strains of Bacillus from soil samples of Paraná State, Brazil, to investigate their potential as biological control agents of soybean seed pathogens. They identified strains of B. subtilis with promising antagonistic activities against several fungal phytopathogens, e.g. R. solani, Colletotrichum truncatum, Sclerotinia sclerotiorum, Macrophomina phaseolina, and Phomopsis spp. On the other hand, Zalila-Kolsi et al. (Citation46) have shown that co-application of B. amyloliquefaciens, B. subtilis, and P. polymyxa bacterial cells led to the highest protection against several phytopathogenic fungi in vivo in wheat. B. subtilis Ch-13, an industrial strain was shown to have a wide spectrum of antagonistic activities against phythopathogens. It produces various metabolites with the potentials to protect plants against fungal pathogens and promote their growth (Citation47).
2.2. Biocontrol of plant diseases
The term biological control was first used a century ago to describe the introduction of exotic species to suppress pests. However, its application is much older than the term itself (Citation48). While the use of chemical-based pesticides increased agricultural productivity, their lasting consequence on the environment urges the use of safe and effective alternatives. In this regard, the use of a biological control agent is an increasingly successful and widespread strategy to decrease plant pathogens and the negative effects of agricultural practices on the environment. Likewise, biological control of phytopthogens has received considerable attention and involves the use of bacteria or fungi with antagonistic activities against plant pathogens (Citation49, Citation50). A large body of literature suggests that elite strains of bacilli protect plants from the phytopathogens (). Some of the strains alone or in the consortium have been commercialized as biocontrol and/or phytostimulants. Application of B. amyloliquefaciens together with commercial fungicides significantly reduces branch canker disease in tea plants under field conditions (Citation51). In a related study, the use of B. amyloliquefaciens together with other microorganisms has been shown to improve the strain’s biocontrol potential (Citation52).
Table 1. Biocontrol of plant pathogens by Bacillus spp.
While biological control is often seen as the safest, most environment-friendly, and cost-effective method, there are challenges related to its application from a practical point of view. Waage et al. (Citation48) argued that the primary challenge with the use of biological control comes from conflicts of interest with the conservation of native species. Lack of consistent efficacy under different environmental conditions and longer registration time are also some of the hindrances that limit the use of Bacillus as biological control of plant pests (Citation53).
One important mechanism by which Bacilli may inhibit fungal pathogens that cause diseases in plants is through enhancing the host plant defence mechanisms by stimulating their immune systems. Surfactants are among the molecules that induce significant protective effects against diseases caused by fungal pathogens (Citation54). This has been demonstrated in maize through the treatment of the roots with a suspension of B. subtilis strain capable of inducing defence genes (Citation55).
A substantial amount of literature is available on the mechanisms of bacilli biocontrol. Genomics and postgenomics study also elucidated the mechanisms in biocontrol of phytopathogens (Citation19). Inhibition of hyphal growth, induction of cell death, competition for space and nutrients, direct effects on mycotoxin biosynthesis and stability, and promotion of host immunity are some of the modes of actions of the Bacillus antagonists (Citation59, Citation87). While biological control has several merits over chemical fungicides, there are also disadvantages if not applied properly. The misuse of biocontrol in terms of dose and frequency, for example, should be avoided.
2.3. Bacillus as insecticides
While plants and insects have coexisted for millions of years, their relationship is not confined to mutual benefits such as pollination but also involves insect predation of plants. Therefore, insect pests are one of the challenges plants face in the environment. Farmers lose a sizeable amount of agricultural production due to various insect pests every year (Citation88). Moreover, the rapid increase of the world population intensified the use of synthetic insecticides that has enabled doubling of worldwide grain production in the last century (Citation89). However, this progress came with undesirable environmental and ecological impacts. Therefore, there is a growing appeal for alternatives with natural and non-synthetic molecules (Citation90). In this regard, Bacillus-based pest control has attracted much attention and has been a subject of increased investigation for last couple of decades. Strains of B. thuringiensis are arguably the most used species as biological control of insect pests as they produce various metabolites that possess wide-spectrum insecticidal properties (Citation91).
Moreover, advances in genetic engineering techniques have enabled the production of transgenic pest-resistant crops by using the genes discovered in B. thuringiensis (Citation92). Maize, potato, brinjal, and cotton were engineered for the expression of the entomocidalδ-endotoxins from various strains of B. thuringiensis (). These toxins have provided efficient suppression of lepidopteran and coleopteran insects, and remarkably increased yield and reduced the application of insecticides (Citation15, Citation18). The worldwide application of Bt technologies remarkably reduced the application of hazardous chemical insecticides and increased the yield and income of the farmers. Increased yield alone, however, can not offset costs associated with genetically engineered seeds and, therefore, requires other considerations such as environmental stewardship and sustainability factored in.
Table 2. Insecticidal proteins/toxins produced by the starins of Bacillus thuringiensis.
Transgenic Bt-crops expressing cry genes from B. thuringiensis are protected against not only insect pests but also pathogenic microbes vectored by those insects. Bt-corn, although not very effective in aflatoxin mitigation, has been shown to reduce fumonisins (FUMs) and deoxynivalenol contamination (Citation100). Aspergillus flavus and A. parasiticus invade the seed through silk and are vectored mainly by insects that are not controlled by Bt-corn (Citation101). Lower mycotoxin levels in Bt-corn have been shown from the feeding damage caused by the European corn borer but not the corn earworm. B. thuringiensis may have additional biocontrol effects. For example, seed treatment with a chitinase from B. thuringiensis-controlled Fusarium infection in soybean (Citation102). Brown plant hopper (BPH; Nilaparvata lugens Stål) is one of the most destructive insect pests, which reduce rice yield remarkably in many rice-growing areas. Based on a high-throughput transcriptome analysis, Rashid et al. (Citation103) demonstrated that systemic resistance induced by B. velezensis YC7010 against BPH is linked with activation of salicylic acid (SA) – and jasmonic acid (JA)-dependent pathways in rice. Furthermore, the authors confirmed that bacterial metabolites, bacillopeptin A, B and X were found to be involved in the induction of systemic resistance in rice (Citation103).
2.4. Reduction of mycotoxin in agricultural produce
Mycotoxins are toxic secondary metabolites produced by various filamentous fungi. They have medical and veterinary importance. The toxins consist of low molecular weight fungal metabolites. Fungi of the genera Aspergillus, Fusarium, and Penicillium are responsible for the production of most mycotoxins reported (Citation104). Bennett (Citation105) describes mycotoxins as natural products that trigger a toxic response in animals, plants, and microorganisms. At present, hundreds of them have been identified and investigated from various fungal species. One of the highly toxic groups of mycotoxins is aflatoxins, which are produced by Aspergillus spp. The Aspergillus infects wheat, walnut, corn, cotton, peanuts, and tree nuts. Consumption of aflatoxin-contaminated food for a while leads to health complications. Liver cancer, a decline in the immune system, malnutrition, and retarded child growth are some of the health impacts caused by aflatoxin consumptions (Citation106). Acute toxicity can lead to liver failure and subsequent death associated with the ingestion of higher amounts of aflatoxin through contaminated foods (Citation107, Citation108). Other groups of mycotoxins such as ochratoxins, fumonisins, deoxynivalenol, patulin, zearalenone (ZEA), and trichothecenes have also been associated with acute or chronic toxicity. Moreover, they are ubiquitous and pose a significant threat to human and animal health (Citation109). Ringot et al. (Citation110) argued that ochratoxin A (OTA) as one of the most harmful metabolites produced by toxigenic fungi has the potential to contaminate products such as cereals, figs, grapes, spices, coffee beans, cocoa beans, pork meat and dried vine fruits. According to Peraica et al. (Citation111) mycotoxicosis has been a threat for millennia basically from the consumption of contaminated food. Few examples, ’St. Anthony’s fire’ caused by toxins from the ergot fungus, Claviceps purpurea, the 1891 cardiac beriberi in Japan, and alimentary toxic aleukia (characterized by nausea, vomiting, diarrhea, leukopenia, hemorrhaging, skin inflammation, and sometimes death) was another example of mycotoxicosis outbreak that occurred during the World War II in Russia and associated with the Fusarium toxin T-2. In addition to the health impact of mycotoxins on humans and animals, they account for a loss of about a quarter of global crop productions leading to the loss of billions of dollars.
While preventing contamination of crops from mycotoxin producing strains is one of the best approaches to curb their effects on humans and animals’ health, degrading the toxins to their harmless state is also a potential method of controlling the damage they cause. While approaches such as the use of chemicals and physical techniques to degrade mycotoxins have been widely described, they are indicated to be unsafe and usually impractical. Hence, one of the methods to mitigate mycotoxin contamination is through biological detoxification in which the toxins are degraded into lesser or nontoxic metabolites (Citation112–116). The application of bacilli as agents of biocontrol of mycotoxin producing fungi is a promising approach for solving this critical problem. A report by Fu et al. (Citation117) showed that a strain of B. licheniformis degrades zearalenone in feed and reduces its toxicity in piglets. Similarly, fengycin producing B. amyloliquefaciens FZB42 controls Fusarium graminearum and significantly reduces mycotoxins such as deoxynivalenol (DON), 3-acetyldeoxinivalenol (3-ADON), 15-acetoxydeoxinivalenol (15-ADON) and zearalenone (ZEN) (Citation125). Biocontrol of mycotoxins in the agricultural produce by the application of Bacillus spp. has been reviewed (Citation118). Moreover, these approaches have received increased attention as they are safe and effective than the use of chemicals for mycotoxin contamination control (Citation110).
2.5. Mechanisms of biocontrol
The members of the genus Bacillus use various mechanisms to suppress phytopathogens. Competing with the pathogen for resources, secretion of secondary metabolites that inhibit the growth of pathogens, and stimulation of the systemic resistance in the host plants are some of the reported mechanisms of biocontrol of plant pests by the bacilli (Citation10).
2.5.1. Antibiosis
Antibiosis as a mechanism of biocontrol of plant pathogens by the Bacillus spp. has extensively been explored. A study published in weekly Nature in 1948 documented the isolation of an antibiotic subtilin from a freeze-dried B. subtils culture (Citation119). Bacillus species have also been hailed as sources of antifungal compounds that have effectively suppressed various pathogens. and presents some major antibiotics produced by various strains of Bacillus species. Cyclic lipopeptides (CLPs) from Bacillus have received much attention in recent years as potential class of antifungal compounds (Citation4). They are rings of amino acids with fatty acid side chains and exhibit remarkable heterogeneity. These structural diversities endowed CLPs with a broad-spectrum and environmentally-stable antimicrobial (Citation120). While CLPs belong to three families, surfactin, iturin, and fengycin, a single Bacillus strain can make one or more of them (Citation121). Although surfactins are characterized as poor antagonists of some pathogenic fungi, they have received attention because of their ability to induce systemic resistance in host plants (122; 56). Fengycins on the other hand, are known to inhibit most filamentous fungi and species such as F. verticilloides (Citation123–125). Their mode of action ranges from disrupting the membrane integrity to organelle dysfunction leading to cell death. The group iturins are also reported to have strong antagonistic activities against A. flavus (Citation126) and F. graminearum (Citation127). Besides, both surfactins and iturins are promoters of biofilm formation (Citation128).
Figure 1. Structures of the few representatives of antimicrobial metabolites discovered from various species of Bacillus.
Table 3. Secondary metabolites or antibiotics produced by the strains of Bacillus species.
Romero et al. (Citation130) reported that iturin and fengycin families of lipopeptides play a major role in the antagonism of B. subtilis against Podosphaera fusca (Citation129). The surfactin – and fengycin-type lipopeptides can act as bacterial determinants in plant cells by initiating the immune response through the stimulation of ISR (induced systemic resistance) (Citation77). Lipopeptides of B. subtilis S499 proved to be effective triggers of ISR in tobacco and bean plants (Citation77). Comparison of the level of production of antibiotic substances with different strains showed the advantages of B. subtilis species. For example, concentrations of lipopeptides in the culture medium of the most active strain of B. subtilis IB-54 exceeded those of active Pseudomonas. ehimensis and P. polymyxa by 10–100 times. Differences between B. subtilis strains were also observed both in an individual activity level and in the spectrum of the lipopeptides formed. It has been established that the main components of B. subtilis lipopeptide complex are iturin, surfactin, and related compounds (Citation129, Citation130). It was also identified that antifungal peptides produced by B. subtilis AU195 show similarity with bacillomycin (group iturin A) and exhibit strong antagonistic activity against Aspergillus flavus and other plant pathogenic fungi. The iturin and fengycin from B. subtilis strains UMAF6614, UMAF6616, UMAF6639, and UMAF8561 were involved in the suppression of cucurbit disease caused by Podosphaera fusca (Citation77). The analysis of culture filtrate, along with the recovery of inhibitory components (fengycin, surfactin, and iturin A or bacillomycin) from melon leaves inoculated with B. subtilis UMAF6614 and B. subtilis UMAF663, strongly supported the evidence of in situ production of these antimicrobials. Bais et al. (Citation131) reported that protective action of surfactin produced by B. subtilis against infection caused by Pseudomonas syringae in Arabidopsis thaliana and suggested that surfactin was necessary for both root colonization and protection of the plant against the pathogen. Ahmad et al. (Citation130) observed that endophytic B. subtilis 330–2 enhanced rice and maize plant growth (ranging 14–37%) and tolerance to biotic/abiotic stresses, regulate the expression of different non-ribosomal polyketide synthases (NRPSs) including surfactin (srfAA), fengycin (fen), and bacillaene (bae). In addition to NRPS, B. subtilis 330–2 triggered the production of secondary metabolites, including antibiotics such as macrolactin (mln), difficidin (dfn), iturin A (ituA), bacillibactin (dhbF), penicillin-binding protein 2B (pbpB) and beta-lactamase (penP), which are important manifestations of a plant’s defence mechanism to cope with competing microorganisms and inhibit the growth of phytopathogenic fungi or bacteria. It should be noted that only 10% of all 114 genes of B. subtilis 330–2 were involved that differentially expressed for antibiotic production (Citation130).
Bacteriocins produced by Bacillus species also display various biological activities that are heat-stable antimicrobial peptides and ribosomally synthesized.
Versatility in their structure and function made bacteriocins potential candidates for antimicrobial study (Citation132). These peptides are active against a wide range of microorganisms, including bacteria, fungi, oomycetes, and viruses. The linear non-cytotoxic lipopeptides, e.g. gageotetrins A-C produced by a marine B. subtilis effectively controlled oomycete Phytophthora capscisi that has a broad host range (Citation133), and the most destructive wheat killer fungus Magnaporthe oryzae Triticum (Citation134).
2.5.2. Production of lytic enzymes
Bacillus species are also good sources of various enzymes. Particularly, the production of hydrolytic enzymes by the species has attracted attention in the last decades. Keratinases, for example, from B. licheniformis and other microorganisms, have been extensively used in a variety of industrial applications such as feed, fertilizer, detergent, and leather industries, as well as for pharmaceutical and biomedical applications (Citation152). The bacilli also serve as a source of vital enzymes such as proteases, lipases, β-glucanases, L-asparaginase and cellulases (). The use of B. subtilis keratinolytic ability to recycle feathers and helped to reduce the environmental impact of the poultry industry. This is an interesting application as keratin contains a significant number of amino acids containing sulphur-forming disulphide bridges which present mechanical stability and resistance to common proteolytic enzymes (Citation153). There are other several classes of enzymes produced by Bacillus spp. with potential industrial and biotechnological applications. A group multi-copper oxidoreductase enzyme, laccases (E.C.1.10.3.2; benzenediol: oxygen-oxidoreductase) with broader substrate affinity to compounds such as phenolic, aniline, aromatic, amines, and some environmental pollutants have been used for decolorization of textile dyes, polymer synthesis, wine and beverage stabilization, detoxification of industrial effluents and biodegradation of environmental pollutants, in production of anti-cancerous and antibiotic drugs, ingredients for cosmetics, and hair coloring (Citation21,Citation154). Chitinolytic activity of some Bacillus spp. plays an important role in the biocontrol of A. parasiticus and show varied level of antifungal activity (Citation155).
Table 4. Lytic enzymes produced by Bacillus spp.
They induce cell death by actively degrading fungal cell walls (Citation160). Jayaraj et al. (Citation161) inoculated rice plants with a strain of B. subtilis capable of producing β-1-3-glucanases and thaumatin and have shown their protective capabilities against sheath blight disease. In a related study, an endophytic B. subtilis has shown protective effects against fungal pathogens. The strain produced β-1,3-glucanase, β-1,4-glucanase, and proteases that could degrade the fungal cell wall composition (Citation130).
2.5.3. Induction of systemic resistance in plants
Plants use various strategies to defend against pathogens and stressors. with, which induced systemic resistance (ISR) mediated by Bacillus spp. have been reported in several studies (Citation162). This defence mechanism is effective against fungi, bacteria, viruses, and insect herbivores (Citation163). Moreover, co-evolution between plants and their pathogens plays a role in the activation of plants’ innate immune system (Citation164). Bais et al. (Citation133) has demonstrated that surfactin and iturin producing Bacillus spp. have successfully reduced infection by Pseudomonas syringae in Arabidopsis. A study by Toure et al. (Citation165) involving inoculation of B. subtilis endospore to apple pulp has demonstrated in vivo synthesis of lipopeptides which have a strong protective effect against the gray mold of apple fruits. Induced systemic resistance and promotion of plant growth by Bacillus spp. have been reviewed (Citation162). A potential bioinsecticidal B. thuringiensis inoculum suppressed gray mold disease through induction of systemic resistance in tomato plants (Citation166). Biocontrol of plant disease by endophytic Bacillus species through induced systemic resistance has also been reviewed recently (Citation167).
2.5.4. Quorum sensing and biofilm formation
Quorum sensing (QS) is a process by which bacteria communicate. It involves detection and mounting of response to extracellular signaling molecules (autoinducers) through secretion of antibiotics, biofilm formation, and production of virulence factors. It allows bacteria to share information about cell density and adjust gene expression accordingly. This process enables bacteria to express energetically expensive processes as a collective only when the impact of those processes on the environment or a host will be maximized. Among the many traits controlled through quorum sensing, one is the expression of virulence factors by pathogenic bacteria (Citation168). This communication process among the cells involves the production, detection, and response to extracellular signaling molecules. As bacterial population density increases, extracellular signaling molecules or autoinducers (AIs) accumulate in the environment. It helps them monitoring the information and track changes in their cell numbers leading to gene expression. The QS controls genes that dictates beneficial activities when performed by groups of bacteria in synchrony. The processes that are controlled by QS include bioluminescence, sporulation, competence, antibiotic production, biofilm formation, and secretions related to virulence factor.
Studies show all known QS systems depend on three basic principles: first, members of the community produce AIs, which are the signaling molecules. At low cell density (LCD), AIs diffuse away present at concentrations below the threshold required for detection. At high cell density (HCD), the cumulative production of AIs leads to a locally high concentration, enabling detection and response (Citation169). Second, AIs are detected by receptors that exist in the cytoplasm or the membrane. Third, in addition to activating the expression of genes necessary for cooperative behaviors, detection of AIs results in activation of AI production (Citation170, Citation171). This feed-forward autoinduction loop presumably promotes synchrony in the population.
Microorganisms come together and form a community that is attached to a surface forming biofilms. Biofilm formation is a common phenomenon among bacteria (Citation172). Several lines of evidence show that plant growth-promoting rhizobacteria (PGPR) such as B. subtilis form beneficial biofilms on plants root (Citation172). They have been reported to protect the host plant from infections. Production of antimicrobial compounds such as surfactin in the biofilm matrix plays a major role in biocontrol. A study by Bais et al. (Citation131) demonstrated that biofilm formed on the roots of Arabidopsis by a strain of B. subtilis in Murashige and Skoog medium has led to protecting effect against P. syringae. The genetic regulatory circuit that governs biofilm formation has been well studied in Bacillus species (Citation173).
Rhizosphere colonization by plant growth-promoting rhizobacteria (PGPR) along plant roots facilitates the ability of PGPR to promote plant growth and health. Xu et al. (Citation122) provided evidence that the major wall teichoic acid (WTA) biosynthetic enzyme GtaB is involved in both biofilm formation and root colonization. The deficiency in biofilm formation of the ΔgtaB mutant may be due to an absence of UDP-glucose, which is necessary for the synthesis of biofilm matrix exopolysaccharides (EPS). Therefore, these observations provide insights into the root colonization process by a plant-beneficial Bacillus strain, which will help improve its application as a biofertilizer. The contribution of bacillomycin D in Bacillus amyloliquefaciens SQR9 to antifungal activity and biofilm formation has been reported (Citation122).
3. Plant growth promotion, quality improvement, and yield increase
A considerable amount of literature is available on the effects of plant growth-promoting rhizobacteria (PGPR) on plant growth and development (Citation174,Citation175). These PGPRs are closely associated with plant roots and promote plants’ defence against pathogens. There is a growing interest in root-colonizing bacteria as supplements or alternatives to chemicals to increase crop productivity in agriculture as they can influence plants’ growth and development through production of phytohormones such as auxins, gibberellins, and cytokinins. They also play a protective role against harmful microorganisms through competition and activation of plant defence mechanisms. Several experimental reports proved these roles of beneficial microorganisms in plant growth and development. A strain of B. megaterium has been shown to promote the growth of A. thaliana and Phaseolus vulgaris plants in vitro and in soil. Inoculation of B. Megaterium to the root system of A. thaliana inhibited primary root growth followed by an increase in lateral root number growth, and root hair length mediated by phytohormones (Citation176). In a similar study, the root-colonizing B. subtilis strain has been reported to suppress soil-borne disease thereby fostering the growth of tomato plants (Citation177). Seed treatment with B. amyloliquefaciens resulted in maximum enhancement of germination (84.75%), seedling vigor (1423.8) and an increase in vegetative growth parameters. Significant protection (71%) against anthracnose disease was observed in plants pretreated with B. amyloliquefaciens (Citation178). Besides production of phytohormones, Bacillus spp. such as B. licheniformis promotes plant growth through fixing atmospheric nitrogen and solubilizing phosphorus.
In addition to ISR, many BCBs also promote plant growth (Citation179), by producing phytohormones that are related to growth conditions (Citation180). For example, plants treated with B. aryabhattai showed greater tolerance to heat stress and produced more abscisic acid, the stress hormone that controls stomatal closure (Citation180). Heat and drought stresses are known to exacerbate mycotoxin synthesis and also insect damage (Citation105). Species such as B. megaterium and Paenibacillus polymyxa can be used as biofertilizers since they can fix nitrogen and solubilize phosphate (Citation181).
Strawberry is an excellent source of natural antioxidants with a high capacity for scavenging free radicals. Rahman et al. (Citation175) demonstrated that application of B. amyloliquefaciens BChi1 not only remarkably increased (43% over control) fruit yield but also enhanced contents of phenolics, carotenoids, flavonoids, anthocyanins and total antioxidants of strawberry fruit over untreated control. However, the mechanism of the quality improvement of fruits by the application of B. amyloliquefaciens is unknown. Further study is needed to elucidate the underlying mechanism of strawberry fruit quality improvement by the B. amyloliquefaciens strain BChi1.
3.1. Production of phytohormones
Plant associated microbes of different genera and species produce vital phytohormones (Citation182). Bacillus strains such as B. amyloliquefaciens, B. megaterium, B. licheniformis, B. pumilus, and B. subtilis are some of the species associated with crops. They reside in the rhizosphere and positively influence plants’ growth and development (Citation162). Phytohormones are also known as plant growth regulators (PGRs) that are produced at a very low concentration but play an important role in plants’ growth and development. Stress tolerance (Citation112), seed dormancy, formation of floral organs, lateral shoot growth (Citation183), cellular proliferation and differentiation, inhibition of premature leaf senescence (Citation184,Citation185), and promotion of heavy metal tolerance are some of the roles they play. Auxins, cytokinins, abscisic acid, gibberellic acid, and salicylic acids are well studied and functionally characterized phytohormones. They also function as signaling molecules enabling plants to mobilize responses to abiotic and biotic stresses (Citation186). A B. Aryabhattai strain isolated from rhizospheric soil of a soybean collected in South Korea for example has shown to significantly promote growth and heat tolerance of the plant through production of abscisic acid, indole acetic acid, cytokinin and different gibberellic acids (Citation187). Bacillus spp., as one of the phytohormone producing bacteria positively impact plants’ growth and development in many ways. They either improve the bioavailability of nutrients or influence phytohormone concentrations (Citation188–190). listed various phytohormones produced by some Bacillus species.
Table 5. Phytohormone production by Bacillus spp.
3.2. Solubilization of nutrients
Rhizobacteria play a role as biofertilizers that enhance plant growth and development. Biofertilizers involve live microorganisms that have potential applications in stimulating plant growth and development (Citation198). They are preferred to chemical fertilizers as they are environmentally benign, support sustainability and may also be less expensive. Examples are phosphate and potassium solubilizing and N2 fixing bacteria. Phosphorus, one of the most important nutrients needed for plant growth and development has limited availability in soluble forms. To alleviate this challenge, either soil should be supplemented with artificial fertilizer, rich in phosphate or phosphate solubilizing microorganisms (PSMs) should be introduced. Various Bacillus spp. have demonstrated phosphate solubilizing potential. Bacilli such as B. circulans, B. megaterium, B. pulvifaciens, and B. sircalmous are few examples (Citation199). Likewise, the role of bacilli in N2 fixation has been known for a long time. The work of Wahab (Citation200) has demonstrated the potential of Bacillus as nitrogen-fixing species. A study by Pramanik et al (Citation201) has also demonstrated that a strain of B. pseudomycoides can be used as a Potassium-solubilizing biofertilizer.
4. Enhancement of stress tolerance
Plants are exposed to adverse environmental conditions known as abiotic stressors in their natural habitats. These stressors include salinity, drought, heat, cold, flooding, heavy metals, and UV radiation that pose serious threats to the sustainability of crop yields. Due to global climate change, the impacts of abiotic stresses on crop production are increasing. Studies have shown that losses to major crops due to abiotic stressors have increased at an alarming rate in recent years posing serious threats to food security (Citation202, Citation203). Since abiotic stresses are major constraints for crop production, finding biorational approaches to enhance stress tolerance is crucial to increase crop production and achieve food security. A large body of literature indicates that application of elite strains of Bacillus spp. enhances tolerance of plants to abiotic stresses (Citation204). Microbial genes providing beneficial effects to plants have been characterized (Citation204, Citation205). The Food and Agriculture Organization of the United Nations (FAO) predicts that current agricultural production should be increased by more than 70% (Citation206, Citation207) to feed the rapidly increasing world population.
4.1. Drought tolerance
As one of the most damaging abiotic stresses, drought affects agricultural productivity worldwide. Reports show that it inhibits growth and development of plants resulting a reduction in yield (Citation206, Citation208). The current trend in climate change shows drought is affecting a wide range of crop-producing countries posing a significant amount of threat to food security. Its effect, however, ranges from moderate and short to extremely severe and prolonged duration. Plants employ various adaptations and mitigation strategies to stand drought stress. They initiate a series of physiological reactions to reduce water loss through the closure of stomata, ABA accumulation, photosynthetic rate reduction, expression of aquaporins and vacuolar H+-pyrophosphatases for maintaining cell turgor through osmotic adjustments, accumulation of compatible osmolytes, and reactive oxygen species (ROS) enzymes, which result in cell integrity, functionality and survival of plants (Citation209–211).
Plants partly rely on soil microorganisms to mitigate stress caused by drought. Plants use microbial molecules such as exopolysaccharides (EPS), phytohormones, and 1-aminocyclopropane- 1-carboxylate (ACC) for drought tolerance. These molecules play a role in plant’s osmoregulation by decreasing electrolyte leakage and promoting the production of metabolites such as amino acids and sugars.
In many regions, agricultural production is adversely affected by a variety of abiotic stresses such as drought, salinity, extreme temperatures, and toxic metals resulting in a significant reduction of crop productivity (Citation201, Citation212). Abiotic stresses are often interrelated and induce general signaling pathways for the regulation of cellular responses aimed at plant adaptation causing similar morphological, physiological, biochemical, molecular, and genetic changes (Citation213–215). Many studies have shown that various strains of B. subtilis contribute to the protection of different host plants against diverse abiotic stresses leading to the increase of plant growth and productivity (Citation130, Citation197, Citation216, Citation217). Investigations on the mechanisms mediated by B. subtilis on plant stress tolerance are of the most urgent scientific demand in modern plant biology due to the fact that B. subtilis-induced responses against stresses could range from diverse to very specific (Citation130,Citation218). The comparative physiological and metabolic analysis revealed that a complex metabolic change in plants is associated with enhanced drought tolerance (Citation219). Application of B.subtilis, B. thuringiensis, and B. megaterium significantly enhanced drought tolerance through increased accumulation of riboflavin, L-asparagine, aspartate, glycerol, nicotinamide, 4-hydroxy methylglycine and 3-hydroxy-3-methylglutarate in the leaves of chickpea (Citation219). Another important aspect associated with the enhancement of drought tolerance in plants by Bacillus spp. is the ability of biofilm formation on plants’ surfaces. In a recent report, Wang et al. (Citation220) demonstrated that B. amyloliquefaciens 54 inoculation in tomato exerts drought tolerance in plants through enhanced expression levels of stress-responsive genes, such as lea, tdi65, and ltpg2 in the treated plants. Enhancement of drought tolerance was also linked to the enhancement of survival rate, relative water content and root vigor in tomato. It was also found that B. amyloliquefaciens 54 induced stomatal closure through the abscisic acid pathway. Biofilm forming ability of Bacillus bacteria was positively correlated with plant root colonization. Interestingly, plants inoculated with hyper-robust biofilm-forming (ΔabrB and ΔywcC) mutants were better able to resist drought stress compared to defective biofilm mutants (Citation220). Although a large body of literature is available on the enhancement of drought tolerance in plants by plant-associated bacilli, precise mechanisms of their beneficial actions in plants are still poorly understood. Further genomics and postgenomics studies are needed to clarify the mode of actions of the elite strains of bacilli associated with plants.
4.2. Salinity tolerance
Disturbed water balance and ion homeostasis lead to soil salinity imposing deleterious effects on plant growth and development (Citation215, Citation221). This leads to a loss in crop yield. Salinity causes oxidative stress, water deficit (Citation222) and deficiency of essential nutrients. It also affects lipid metabolism, protein synthesis, and biomass accumulation (Citation215). This also impacts the process of photosynthesis leading to perturbations in carbon and nitrogen assimilatory pathways (Citation223). It has also been associated with the accumulation of toxic ions such as Na+ and Cl- in cells resulting in immediate stress. To mitigate the effects of salt stress, plants employ strategies that regulate water status and have osmoregulatory functions. They initiate the expression of genes coding for various enzymes and accumulation of metabolites such as amino acids, sugars, and betaines (Citation224).
Harnessing the potential of beneficial microorganisms present in the rhizosphere is an alternative strategy for improving plant stress tolerance. Promising results have been reported in areas where saline irrigation of crops is practiced. A handful of studies revealed that some elite strains of plant-associated bacilli enhance plants’ tolerance to salinity (Citation204, Citation205). In the Sinai region of Egypt, a strain of B. subtilis was field-tested and reported to promote salt tolerance in two cultivars of eggplant and pepper in saline soil (Citation225). B. subtilis has been reported to enhance the production of phytohormones: auxin, IAA, CKs, and giberellines in crops such as wheat thereby increasing their salinity tolerance (Citation225–227). In a similar study, Bochow et al. (Citation225) described that plants in saline soil that grew from B. subtilis FZB2 treated seeds showed a multi-fold increase in yield. In a similar study, endophytic strain B. subtilis NUU4 improved symbiotic performance of host plant (Cicer arietinum L.) with rhizobia by uptaking more nutrients, which resulted in a significant increase in growth parameters (roots and shoots) and yield under salinity compared to non-inoculated control plants (Citation197). This strain is known to produce IAA, HCN, siderophores, cell wall degrading enzymes, and demonstrated antagonistic activity against F. oxysporum, F. solani, F. culmorum, B. cinerea, and A. alternata. Most of the common osmoprotectants are low molecular weight metabolites such as amino acids that show no toxicity towards plant tissues when present in higher concentrations (Citation228). The amino acid proline is known to enable plants to cope with osmotic stress (Citation229). An important mechanism of rhizobacteria-induced salt tolerance in plants is the production of enzyme 1-aminocyclopropane-1-carboxylic acid (ACC) deaminase, which acts by degrading ACC (Citation230). The enzymatic activity of ACC deaminase results in the production of alpha-ketoglutarate and ammonia, which, by lowering ACC levels, prevents excessive increases in the synthesis of ethylene and enhances plants tolerance to salt stress.
Rhizobacteria exert beneficial functions to plants through induction of gene expression in the interacting plants. For example, B. amyloliquefaciens-SN13 induced changes in expression of a considerable number of photosynthesis, hormone, and stress responsive genes in rice under salt stress as compared to salt stress or SN13 inoculation alone, indicating its potential role in reducing harmful effects of salinity (Citation204). Functional expression of rice genes OsNAM and OsGRAM in yeast showed enhanced tolerance to various abiotic stresses including salinity. (Citation204). Likewise, expression analysis of OsGRAM genes in rice roots subjected to salt stress with or without B. amyloliquefaciens SN13 inoculation revealed significant differential expression patterns suggesting their crucial role in beneficial plant-rhizobacteria interactions under salinity (Citation205). Further study using mutants of OsGRAM would precisely elucidated the role of these genes in salt tolerance induction in rice by SN13. Findings of these and other studies demonstrate that Bacillus spp. has the potential to induce gene expression in plants associated with salt tolerance. Bacteria that enhance salt tolerance in plants are recruited by root exudate secreted from the roots of the host plants. The secreted organic acids are 2-methylbutyric acid, stearic acid, palmitic acid, palmitoleic acid, and oleic acid. Precise molecular cross-talks between roots and rhizobacteria are needed to be elucidated for getting better benefit from the elite strains of bacteria that exert salt tolerance to plants.
4.3. Low temperature tolerance
Cold stress can affect plant distribution, development, and productivity thereby threatening food security by limiting crop yield. Plants activate various physiological and molecular processes in response to cold stress (Citation220). In particular, Bacillus spp. has demonstrated the capability of increasing plant’s tolerance against cold. They have genetic features that enable them to initiate a physiological response to cold stress including synthesizing of cold shock proteins, initiation of signal transduction pathways, osmotic regulation, membrane transportation, and antioxidant enzymes (Citation231, Citation232).
Advancement in biotechnology has enabled the identification of genes associated with stress tolerance. While this opens opportunities to improve cold tolerance by gene transformation or altering multigenic traits, challenges do exist in understanding the changes in cellular, biochemical and molecular mechanisms that occur in response to cold stress (Citation233)
Members of the Bacillus genus are among the most naturally abundant plant growth-promoting rhizobacteria (PGPR) in the soil. As Bacillus spp. are among the most naturally abundant PGPR capable of producing phytohormones such as AUX, CK, and GA, they can also increase plant growth by reducing their susceptibility to stressors such as cold (Citation205). They cause alterations in metabolic and physiological activities leading to production of metabolites, abundant proteins with protective properties during cold stress. Zubair et al. (Citation232) demonstrated that inoculation of cold-tolerant Bacillus strains aided in inducing stress responses in wheat by regulating abscisic acid, lipid peroxidation and proline accumulation pathways in a beneficial manner.
5. Bioremediation of heavy metals and contaminated soils
The discharge of untreated industrial wastewater containing biotoxic substances of heavy metals in the ecosystem is one of the most important environmental and health challenges society facing today. Hence, there is a growing need for the development of a novel, efficient, eco-friendly, and cost-effective approach for the remediation of toxic metals (Cr, Hg, Cd, and Pb) released into the environment and to safeguard the ecosystem. In this regard, recent advances in microbe-based heavy metal bioremediation have propelled a prospective alternative to conventional techniques. Bioremediation is a process that involves all methods and activities for the reduction of environmental pollutants with the help of biological entities. Bioremediation offers a promising means to reclaim such contaminated soil in an economical and eco-friendly way (Citation234). Metal toxicity is of great environmental concern because of its bioaccumulation and non-biodegradability in nature (Citation235). Bioremediation is employed to transform toxic heavy metals into a less harmful state using microbes. Species of Bacillus isolated from solar salterns were shown to have a potential of detoxifying the heavy metals such as lead, chromium, and copper by biosorption (Citation236).
Pollution of soils with heavy metals (HM) has become a common phenomenon throughout the world due to the increase in the scale of anthropogenic activities. The plants growing on HM contaminated soils are characterized by a decreased growth rates and yields (Citation237). Some of the direct toxic effects caused by high concentrations of HM include inhibition of cytoplasmic enzymes and damage to cellular structures due to the development of oxidative stress (Citation238). An example of an indirect toxic effect is the replacement by HM of cations in the functional groups of various bioorganic compounds (Citation239). Moreover, the negative influence of HM on the activity of soil microorganisms can indirectly affect plant growth. The decrease in the number of beneficial soil microorganisms due to the high concentration of metal can lead to a decrease in the decomposition of organic matter, which in turn can cause a decrease in the content of certain nutrients in the soil. The PGPB, including B. subtilis, can grow in HM-contaminated environment and protect plants against toxicity of HM (Citation240). Beneficial microorganisms have different mechanisms for HM tolerance through mobilization, immobilization, and transformation to less toxic forms. Such mechanisms include exclusion, extrusion, active removal, biotransformation, biosorption, precipitation or bioaccumulation of metals both in external and intracellular spaces of plants (Citation237). Naseem et al. (Citation241) demonstrated that inoculation of wheat with Bacillus sp. AMP2 caused a reduction in the Cr uptake of seedlings both at 10 and 20 μg mL-¹ chromium salts (CrCl3, K2CrO4 and K2Cr2O7) when compared with non-inoculated plants. Moreover, increased levels of acid phosphatase and PA were recorded in bacterial inoculated plants compared to the control (Citation241). In a related study, inoculation of wheat plants with B. subtilis QM3 promoted their growth in the presence of Pb (Citation240) with concurrent increase of antioxidant enzymes like superoxide dismutase (SOD, EC 1.15.1.1), ascorbate peroxidase (APX, EC 1.11.1.1), and catalase (CAT, EC 1.11.1.6) in plant tissues and decrease of metal concentration in the roots in comparison with non-inoculated control plants (Citation240). It was shown that endophytic strains of B. subtilis26D and 11VM improves tolerance of S. alba to the toxic effect of Cd and Ni and reduces manifestation of oxidative stress in the presence of higher levels of metal ions in the above-ground part of plants (Citation242). Treesubsuntorn et al. (Citation243) showed that B. subtilis inoculation of rice (Oryza sativa L.) can highly reduce Cd accumulation in every part of rice roots and shoots (45 days), and grains (120 days). B. subtilis can effectively absorb Cd compared to B. cereus, which might be the main mechanism to reduce Cd transportation in rice plants. Interestingly, plants that were inoculated with bacterial species individually, harbored higher calcium (Ca) and magnesium (Mg) accumulation; B. subtilis-inoculated plants had the highest levels of Ca and Mg compared to plants inoculated with B. cereus. Moreover, B. subtilis could increase the dry weight of the rice plant and protect them from Cd stress due to the ability to produce IAA, solubilize phosphate, and control ethylene levels by ACC-deaminase activity (Citation243).
Arguably one of the environmental challenges we face today is soil contamination of our environment. A petroleum waste causes an imbalance between carbon, nitrogen, and phosphorus ratio in soil affecting all forms of life (Citation244). As cleaning is costly and labor-intensive, companies show less interest in the use of physicochemical methods. On the other hand, bioremediation is attracting attention as an alternative to physicochemical methods of cleaning an oil-contaminated environment (Citation245). The use of microorganisms that have the potential to degrade pollutants (Bioaugmentation) can be employed to degrade petroleum wastes (Citation246). Microorganisms produce various biosurfactants. These molecules play a significant role in reducing surface tension and critical micelle dilution. This favors the creation of micro-emulsion formation leading to the solubilization of hydrocarbons in water (Citation247). Application of biosurfactants as bioremediation in an oil-contaminated environment has an advantage over the use of synthetic surfactants as they are less toxic and more biodegradable (Citation248). Moreover, biosurfactants show efficiency, high selectivity, and versatility (pH and salinity) (Citation245).
Some members of the microbial community might have the ability to secrete important degradative enzymes and growth factors, whereas others can exhibit the potential of biosurfactant production leading to the enhanced solubilization of hydrophobic hydrocarbons for their better utilization by microbes (Citation249). Ubiquitous presence of cypermethrin as a contaminant in surface stream and soil necessitates developing potential bioremediation methods to degrade and eliminate this pollutant from the environment. B. subtilis has been found efficient in biodegradation and detoxification of cypermethrin (Citation250). Enhanced degradation of cypermethrin by B. thuringiensis strain SG4 has also been reported (Citation251). The β-glalactosidase generated by B. subtilis catalyzes the hydrolysis of o-nitrophenyl-β-D-galactopyranoside (ONPG) to produce o-nitrophenol (ONP), which can be detected at 420 nm and used to evaluate acute biotoxicity of heavy metal ions that inhibit the activity of the enzyme (Citation252). This B. subtilis-based bioassay is a sensitive, economically feasible, simple and promising alternative for acute biotoxicity assessment. Generally, remediation of heavy metal using microbes has several advantages. It is eco-friendly and considered to be a long-term solution compared to the physical and chemical methods. However, shortcomings like slowness of the process, difficulty in controlling the treatment, specificity of the microbes towards a particular metal contaminant, and problem with large-scale applications are the areas that need improvement.
6. Bacillus as a reservoir of novel genes for engineering
B. thuringiensis is ubiquitous in nature. It produces insecticidal crystal proteins (Cry) on sporulation. The most widely known toxins of B. thuringiensis include the δ-endotoxins; Cry and Cyt toxins. Cry proteins consist of various groups based on their structure (Citation253). Strains of B. thuringiensis are recognized as one of the most used biocontrol agents. Crystal proteins produced by the species are commercially available for pest control. They show larvicidal activities against larvae of hymenoptera, homoptera, lepidopteran, dipteran, coleopteran etc (Citation254). Recent advancements in the omics technology has created an opportunity to mine for potential gene cluster to increase bioactive metabolites discovery. Moreover, the host-specific toxicity of Bt proteins has attracted researchers’ attention to the search for genes potentially coding for novel toxins within the group. Selective solubility of Bt crystal and their specificity for their hosts have gained worldwide attention as an alternative to chemical insecticides. The usefulness of these insecticidal proteins has also motivated researcher to explore new Bt isolates from the most diverse habitats in order to identify and characterize new insecticidal proteins with different specificities. Some of these isolates exhibit novel and unexpected toxic activities against organisms other than insects, suggesting a pluripotential nature of some toxins. Bt corn, cotton, brinjal etc. that have been genetically modified to express insecticidal toxins derived from the bacterium B. thuringiensis to kill lepidopteran pests feeding on these plants. Genetically modified Bt corn has lower levels of mycotoxins compared to non-Bt (Citation255). On farm field trials revealed that Bt cotton technology substantially reduces pest damage and increases yields (Citation256). Discussions and debates of environmental risks and benefits of adopting genetically engineered Bt technology in corn, cotton and brinjal are highly polarized between pro – and anti-biotechnology groups (Citation257). Nonetheless, the current state of our knowledge is frequently overlooked in this debate. While organic and conventional crops’ value could be jeopardized if genes from GE crops flow to non-GE varieties through cross-pollination or seed mingling, a survey of existing scientific literature reveals that key experiments on both the environmental risks and benefits are lacking (Citation257). However, the number of genes present in various species of Bacillus that encode insecticidal toxins are great resources for future research and judicious introgression in different crops.
7. Bacillus spp. as a source of carotenoids
Carotenoids represent one of the most abundant pigments available in nature. They have considerable industrial applications in biotechnology, food processing, and pharmaceuticals (Citation258). They are also currently receiving attention as studies indicate that they can be used to prevent the incidence of cancers, cardiovascular diseases, and some degenerative diseases (Citation259–262). This prompted an interest in the commercial production of carotenoids. Few studies have reported bacilli as potential sources of carotenoids. Sy et al. (Citation263) recovered two Bacillus species, B. indicus and B. firmus capable of producing carotenoids from a marine environment. A strain of B. megaterium has also been characterized as a potential producer of carotenoids (Citation264).
8. Concluding remarks and future perspectives
The genus Bacillus represents spore-forming Gram-positive bacteria. They are ubiquitous in the environment. They produce diverse secondary metabolites that have applications in the fields of biotechnology and agriculture. They are good sources of bioactive compounds, enzymes, vitamins, and other several secondary metabolites that could be used for industrial applications. Members of the genus have also been known for their effect on plant growth and development. They improve crop yield through enhancing plant stress tolerance, disease defence, and through facilitating minerals and water uptake. Moreover, the current trend in the rapid increase of the world population together with climate unpredictability urges immediate and effective strategies to increase crop productivity. This subsequently involves the use of synthetic chemicals and fertilizers that may make the whole production system unsustainable with unwanted environmental consequences. Therefore, developing biological control against pests and plant pathogens, biofertilizers, and stress-tolerant crop varieties are the areas of future research. Despite their immense potential, bacilli are not fully utilized in the fields of agriculture and biotechnology. Therefore, research on bacilli should aim at (i) searching for novel bioactive metabolites that could be used as lead compounds in developing effective remedy against plant pathogens; (ii) developing biofertilizers that have minimal adverse impact on the environment; (iii) selecting strains that have the potential of stimulating plant defence mechanisms and improve yield; and (iv) applying bacilli species as a source of plant growth hormones.
Disclosure statement
No potential conflict of interest was reported by the author(s).
Additional information
Funding
References
- Shewan, J.M.; Hodgkiss, W.; Liston, J. A Method for the Rapid Differentiation of Certain non-Pathogenic, Asporogenous Bacilli. Nature 1954, 173, 208–209.
- Krawczyk, A.O.; de Jong, A.; Eijlander, R.T.; Berendsen, E.M.; Holsappel, S.; Wells-Bennik, M.H.; Kuipers, O.P. Next-generation Whole-Genome Sequencing of Eight Strains of Bacillus Cereus, Isolated from Food. Genome. Announc. 2015, 3, e01480–15.
- Sharp, R.J.; Riley, P.W.; White, D. Heterotrophic Thermophilic Bacilli. In: Thermophilic Bacteria. Kristjanssono, J.K.,, Ed.; CRC Press, Inc.: Boca Raton. 1992; pp. 19–50.
- Stein, T. Bacillus Subtilis Antibiotics: Structures, Syntheses and Specific Functions. Mol. Microbiol 2005, 56, 845–857.
- Piewngam, P.; Zheng, Y.; Nguyen, T.H.; Dickey, S.W.; Joo, H.S.; Villaruz, A.E.; Glose, K.A.; Fisher, E.L.; Hunt, R.L.; Li, B.; Chiou, J. Pathogen Elimination by Probiotic Bacillus via Signalling Interference. Nature 2018, 562, 532–537.
- Kawulka, K.E.; Sprules, T.; Diaper, C.M.; Whittal, R.M.; McKay, R.T.; Mercier, P.; Zuber, P.; Vederas, J.C. Structure of Subtilosin A, a Cyclic Antimicrobial Peptide from Bacillus Subtilis with Unusual Sulfur to α-Carbon Cross-Links: Formation and Reduction of α-Thio-α-Amino Acid Derivatives. Biochemistry 2004, 43, 3385–3395.
- Chen, X.H.; Vater, J.; Piel, J.; Franke, P.; Scholz, R.; Schneider, K.; Koumoutsi, A.; Hitzeroth, G.; Grammel, N.; Strittmatter, A.W.; Gottschalk, G. Structural and Functional Characterization of Three Polyketide Synthase Gene Clusters in Bacillus Amyloliquefaciens FZB 42. J. Bacterial 2006, 188, 4024–4036.
- Joseph, B.; Dhas, B.; Hena, V.; Raj, J. Bacteriocin from Bacillus Subtilis as a Novel Drug Against Diabetic Foot Ulcer Bacterial Pathogens. Asian Pac. J. Trop. Biomed 2013, 3, 942–946.
- Shoda, M. Bacterial Control of Plant Diseases. Biosci. Bioeng 2000, 89, 515–521.
- Islam, M.T.; Kim, K.H.; Choi, J. Wheat Blast in Bangladesh: the Current Situation and Future Impacts. Plant Pathol. J 2019, 35, 1.
- Islam, M.; Laatsch, H.; von Tiedemann, A. Inhibitory Effects of Macrotetrolides from Streptomyces spp. on Zoosporogenesis and Motility of Peronosporomycete Zoospores are Likely Linked with Enhanced ATPase Activity in Mitochondria. Front. Microbiol. 2016, 7, 1824.
- Kumar, S.N.; Sreekala, S.R.; Chandrasekaran, D.; Nambisan, B.; Anto, R.J. Biocontrol of Aspergillus Species on Peanut Kernels by Antifungal Diketopiperazine Producing Bacillus Cereus Associated with Entomopathogenic Nematode. PLOS one 2014, 9 (8). doi:10.1371/journal.pone.0106041
- Clarke, J.L.; Zhang, P. Plant Biotechnology for Food Security and Bioeconomy. Plant Mol. Biol 2013, 83, 1–3.
- Compant, S.; Duffy, B.; Nowak, J.; Clément, C.; Barka, E.A. Use of Plant Growth-Promoting Bacteria for Biocontrol of Plant Diseases: Principles, Mechanisms of Action, and Future Prospects. Appl. Environ. Microbiol 2005, 71, 4951–4959.
- Gatehouse, A.M.; Ferry, N.; Edwards, M.G.; Bell, H.A. Insect-resistant Biotech Crops and Their Impacts on Beneficial Arthropods. Philos. T.R. Soc B: Biol. Sci. 2011, 366, 1438–1452.
- Toenniessen, G.H.; O’Toole, J.C.; DeVries, J. Advances in Plant Biotechnology and its Adoption in Developing Countries. Curr. Opin. Plant Biol 2003, 6, 191–198.
- Brookes, G.; Barfoot, P. GM Crops: The Global Economic and Environmental Impact-The First Nine Years 1996-2004. AgroBioForum. 2005, 8, 187–196.
- Shelton, A.M.; Hossain, M.J.; Paranjape, V.; Azad, A.K.; Rahman, M.L.; Khan, A.S.; Prodhan, M.Z.; Rashid, M.A.; Majumder, R.; Hossain, M.A.; Hussain, S.S. Bt Eggplant Project in Bangladesh: History, Present Status, and Future Direction. Front. Bioeng. Biotechnol 2018, 6, 106.
- Dash, H.R.; Mangwani, N.; Chakraborty, J.; Kumari, S.; Das, S. Marine Bacteria: Potential Candidates for Enhanced Bioremediation. Appl. Microbiol. Biotechnol 2013, 97, 561–571.
- Duc, L.H.; Fraser, P.D.; Tam, N.K.; Cutting, S.M. Carotenoids Present in Halotolerant Bacillus Spore Formers. FEMS Microbiol. Lett 2006, 255, 215–224.
- Shakambari, G.; Birendranarayan, A.K.; Lincy, M.J.; Rai, S.K.; Ahmad, Q.T.; Ashokkumar, B.; Saravanan, M.; Mahesh, A.; Varalakshmi, P. Correction: Hemocompatible Glutaminase Free l-Asparaginase from Marine Bacillus Tequilensis PV9W with Anticancer Potential Modulating p53 Expression. RSC Adv. 2016, 6, 32089.
- Hamdache, A.; Lamarti, A.; Aleu, J.; Collado, I.G. Non-peptide Metabolites from the Genus Bacillus. J. Nat. Prod 2011, 74, 893–899.
- Zhang, M.M.; Wang, Y.; Ang, E.L.; Zhao, H. Engineering Microbial Hosts for Production of Bacterial Natural Products. Nat. Prod. Rep 2016, 33, 963–987.
- Dame, Z.T.; Islam, M.T. Tapping the Potential of Metabolomics in New Natural Products Discovery from Bacillus Species. In Bacilli and Agrobiotechnology: Phytostimulation and Biocontrol; Islam, M.T., Ed.; Springer: Cham, 2019; pp. 201–215.
- Duan, G.; Christian, N.; Schwachtje, J.; Walther, D.; Ebenhöh, O. The Metabolic Interplay Between Plants and Phytopathogens. Metabolites 2013, 3, 1–23.
- Krebs, B.; Höding, B.; Kübart, S.; Workie, M.A.; Junge, H.; Schmiedeknecht, G.; Grosch, R.; Bochow, H.; Hevesi, M. Use of Bacillus Subtilis as Biocontrol Agent. I. Activities and Characterization of Bacillus Subtilis Strains/Anwendung von Bacillus Subtilis als Mittel für den Biologischen Pflanzenschutz. I. Aktivitäten und Charakterisierung von Bacillus Sibtills-Stämmen. Zeitschrift für Pflanzenkrankheiten und Pflanzenschutz. J. Plant Dis. Protect 1998, 105, 181–197.
- Beneduzi, A.; Ambrosini, A.; Passaglia, L.M. Plant Growth-Promoting Rhizobacteria (PGPR): Their Potential as Antagonists and Biocontrol Agents. Genet. Mol. Biol 2012, 35, 1044–1051.
- Asaka, O.; Shoda, M. Biocontrol of R. Solani Damping-off of Tomato with Bacillus Subtilis RB14. Appl. Environ. Microbiol 1996, 62, 4081–4085.
- Mnif, I.; Ghribi, D. Review Lipopeptides Biosurfactants: Mean Classes and new Insights for Industrial, Biomedical, and Environmental Applications. Pept. Sci. 2015, 104, 129–147.
- Surovy, M.Z.; Gupta, D.R.; Mahmud, N.U.; Dame, Z.T.; Roy, P.K.; Islam, M.T. Genomics and Post-Genomics Approaches for Elucidating Molecular Mechanisms of Plant Growth-Promoting Bacilli. In Bacilli and Agrobiotechnology: Phytostimulation and Biocontrol; Islam MT et al., Ed.; Springer: Cham, 2019; pp. 161–200.
- Islam, M.T.; von Tiedemann, A.; Laatsch, H. Protein Kinase C is Likely to be Involved in Zoosporogenesis and Maintenance of Flagellar Motility in the Peronosporomycete Zoospores. Mol. Plant-Microbe Interact 2011, 24, 938–947.
- Islam, M.T.; Fukushi, Y. Growth Inhibition and Excessive Branching in Aphanomyces Cochlioides Induced by 2, 4-Diacetylphloroglucinol is Linked to Disruption of Filamentous Actin Cytoskeleton in the Hyphae. World J. Microbiol. Biotechnol. 2010, 26, 1163–1170.
- Chirakkal, H.; O’Rourke, M.; Atrih, A.; Foster, S.J.; Moir, A. Analysis of Spore Cortex Lytic Enzymes and Related Proteins in Bacillus Subtilis Endospore Germination. Microbiology 2002, 148, 2383–2392.
- Saber, W.I.; Ghoneem, K.M.; Al-Askar, A.A.; Rashad, Y.M.; Ali, A.A.; Rashad, E.M. Chitinase Production by Bacillus Subtilis ATCC 11774 and its Effect on Biocontrol of Rhizoctonia Diseases of Potato. Acta Biol. Hung. 2015, 66, 436–448.
- Kapoor, R.; Harde, H.; Jain, S.; Panda, A.K.; Panda, B.P. Downstream Processing, Formulation Development and Antithrombotic Evaluation of Microbial Nattokinase. J. Biomed. Nanotechnol 2015, 11, 1213–1224.
- Swift, S.M.; Etobayeva, I.V.; Reid, K.P.; Waters, J.J.; Oakley, B.B.; Donovan, D.M.; Nelson, D.C. Characterization of LysBC17, a Lytic Endopeptidase from Bacillus Cereus. Antibiotics 2019, 8, 155.
- Verschuere, L.; Rombaut, G.; Sorgeloos, P.; Verstraete, W. Probiotic Bacteria as Biological Control Agents in Aquaculture. Microbiol. Mol. Biol. Rev 2000, 64, 655–671.
- Fisher, M.C.; Henk, D.A.; Briggs, C.J.; Brownstein, J.S.; Madoff, L.C.; McCraw, S.L.; Gurr, S.J. Emerging Fungal Threats to Animal, Plant and Ecosystem Health. Nature 2012, 484, 186–194.
- Asaka, O.; Shoda, M. Biocontrol of R. Solani Damping-off of Tomato with Bacillus Subtilis RB14. Appl. Environ. Microbiol 1996, 62, 4081–4085.
- Ji, S.H.; Paul, N.C.; Deng, J.X.; Kim, Y.S.; Yun, B.S.; Yu, S.H. Biocontrol Activity of Bacillus Amyloliquefaciens CNU114001 Against Fungal Plant Diseases. Mycobiology 2013, 41, 234–242.
- Toral, L.; Rodríguez, M.; Béjar, V.; Sampedro, I. Antifungal Activity of Lipopeptides from Bacillus XT1 CECT 8661 Against Botrytis Cinerea. Front.Microbiol 2018, 9, 1315.
- Tareq, F.S.; Shin, H.J. Bacilotetrins A and B, Anti-Staphylococcal Cyclic-Lipotetrapeptides from a Marine-Derived Bacillus Subtilis. J. Nat. Prod 2017, 80, 2889–2892.
- Yang, F.; Jacobsen, S.; Jørgensen, H.J.; Collinge, D.B.; Svensson, B.; Finnie, C. Fusarium Graminearum and its Interactions with Cereal Heads: Studies in the Proteomics era. Front. Plant Sci 2013, 28, 37.
- Gu, Q.; Yang, Y.; Yuan, Q.; Shi, G.; Wu, L.; Lou, Z.; Huo, R.; Wu, H.; Borriss, R.; Gao, X. Bacillomycin D Produced by Bacillus Amyloliquefaciens is Involved in the Antagonistic Interaction with the Plant-Pathogenic Fungus Fusarium Graminearum. Appl. Environ. Microbiol 2017, 83, e01075–17.
- Araujo, F.F.; Henning, A.A.; Hungria, M. Phytohormones and Antibiotics Produced by Bacillus Subtilis and Their Effects on Seed Pathogenic Fungi and on Soybean Root Development. World J. Microbiol. Biotechnol 2005, 21, 1639–1645.
- Zalila-Kolsi, I.; Mahmoud, A.B.; Ali, H.; Sellami, S.; Nasfi, Z.; Tounsi, S.; Jamoussi, K. Antagonist Effects of Bacillus spp. Strains Against Fusarium Graminearum for Protection of Durum Wheat (Triticum Turgidum L. Subsp. Durum). Microbiol. Res 2016, 192, 148–158.
- Makarova, N.M.; Shaposhnikov, A.I.; Kravchenko, L.V. Antifungal and Phytostimulating Characteristics of Bacillus Subtilis Ch-13 Rhizospheric Strain, Producer of Bioprepations. Appl. Biochem. Microbiol 2009, 45, 419–423.
- Waage, J.K.; Greathead, D.J. Biological Control: Challenges and Opportunities. Philos. T.R. Soc B: Biol. Sci. 1988, 318, 111–128.
- Islam, M.T.; Hashidoko, Y.; Deora, A.; Ito, T.; Tahara, S. Suppression of Damping-off Disease in Host Plants by the Rhizoplane Bacterium Lysobacter sp. Strain SB-K88 is Linked to Plant Colonization and Antibiosis Against Soilborne Peronosporomycetes. Appl. Environ. Microbiol 2005, 71, 3786–3796.
- Gaiero, J.R.; McCall, C.A.; Thompson, K.A.; Day, N.J.; Best, A.S.; Dunfield, K.E. Inside the Root Microbiome: Bacterial Root Endophytes and Plant Growth Promotion. Am.J.Bot 2013, 100, 1738–1750.
- Jeyaraman, M.; Robert, P.S. Bio Efficacy of Indigenous Biological Agents and Selected Fungicides Against Branch Canker Disease of (Macrophoma Theicola) tea Under Field Level. BMC Plant Biol. 2018, 18, 222.
- Wu, Y.; Xu, L.; Chang, L.; Ma, M.; You, L.; Jiang, C.; Li, S.; Zhang, J. Bacillus Thuringiensis cry1C Expression from the Plastid Genome of Poplar Leads to High Mortality of Leaf-Eating Caterpillars. Tree Physiol. 2019, 39, 1525–1532.
- Velivelli, S.L.; Sessitsch, A.; Prestwich, B.D. The Role of Microbial Inoculants in Integrated Crop Management Systems. Potato Res 2014, 57, 291–309.
- Cawoy, H.; Debois, D.; Franzil, L.; De Pauw, E.; Thonart, P.; Ongena, M. Lipopeptides as Main Ingredients for Inhibition of Fungal Phytopathogens by Bacillus Subtilis/Amyloliquefaciens. Microb. Biotechnol 2015, 8, 281–295.
- Gond, S.K.; Bergen, M.S.; Torres, M.S.; White Jr., J.F. Endophytic Bacillus spp. Produce Antifungal Lipopeptides and Induce Host Defence Gene Expression in Maize. Microbiol.Res 2015, 172, 79–87.
- Yuan, J.; Li, B.; Zhang, N.; Waseem, R.; Shen, Q.; Huang, Q. Production of Bacillomycin-and Macrolactin-Type Antibiotics by Bacillus Amyloliquefaciens NJN-6 for Suppressing Soilborne Plant Pathogens. J. Agric. Food Chem 2012, 60, 2976–2981.
- Gautam, S.; Chauhan, A.; Sharma, R.; Sehgal, R.; Shirkot, C.K. Potential of Bacillus Amyloliquefaciens for Biocontrol of Bacterial Canker of Tomato Incited by Clavibacter Michiganensis ssp. Michiganensis. Microb. Pathog. 2019, 130, 196–203.
- Banerjee, G.; Gorthi, S.; ChattoPadhyay, P. Beneficial Effects of bio-Controlling Agent Bacillus Cereus IB311 on the Agricultural Crop Production and its Biomass Optimization Through Response Surface Methodology. Anais da Academia Brasileira de Ciências 2018, 90, 2149–2159.
- Geng, C.; Nie, X.; Tang, Z.; Zhang, Y.; Lin, J.; Sun, M.; Peng, D. A Novel Serine Protease, Sep1, from Bacillus Firmus DS-1 has Nematicidal Activity and Degrades Multiple Intestinal-Associated Nematode Proteins. Sci. Rep 2016, 6, 1–2.
- Xiong, J.; Zhou, Q.; Luo, H.; Xia, L.; Li, L.; Sun, M.; Yu, Z. Systemic Nematicidal Activity and Biocontrol Efficacy of Bacillus Firmus Against the Root-Knot Nematode Meloidogyne Incognita. World J. Microbiol. Biotechnol 2015, 31 (4), 661–667.
- Slama, H.B.; Cherif-Silini, H.; Chenari Bouket, A.; Qader, M.; Silini, A.; Yahiaoui, B.; Alenezi, F.N.; Luptakova, L.; Triki, M.A.; Vallat, A.; Oszako, T. Screening for Fusarium Antagonistic Bacteria from Contrasting Niches Designated the Endophyte Bacillus Halotolerans as Plant Warden Against Fusarium. Front.Microbiol. 2019, 9, 3236.
- Irkitova, A.N.; Grebenshchikova, A.V. Antimicrobial Action of a Bacterial Consortium Containing Strains of the Genus Bacillus. Ukr. J. Ecol 2018, 8 (4), 444–449.
- Gutiérrez-Mañero, F.J.; Ramos-Solano, B.; Probanza, A.N.; Mehouachi, J.R.; Tadeo, F.; Talon, M. The Plant-Growth-Promoting Rhizobacteria Bacillus Pumilus and Bacillus Licheniformis Produce High Amounts of Physiologically Active Gibberellins. Physiol. Plant. 2001, 111, 206–211.
- Tendulkar, S.R.; Saikumari, Y.K.; Patel, V.; Raghotama, S.; Munshi, T.K.; Balaram, P.; Chattoo, B.B. Isolation, Purification and Characterization of an Antifungal Molecule Produced by Bacillus Licheniformis BC98, and its Effect on Phytopathogen Magnaporthe Grisea. J. Appl. Microbiol 2007, 103, 2331–2339.
- Jeong, M.H.; Lee, Y.S.; Kim, K.Y. Isolation of Bacillus licheniformis MH48 with antagonistic activity against plant pathogens. InRecent trends in PGPR research for sustainable crop productivity. 4th Asian PGPR Conference Proceedings, Hanoi, Vietnam, 2015 2016 (pp. 251-258). Asian PGPR Society.
- Won, S.J.; Kwon, J.H.; Kim, D.H.; Ahn, Y.S. The Effect of Bacilluslicheniformis MH48 on Control of Foliar Fungal Diseases and Growth Promotion of Camellia Oleiferaseedlings in the Coastal Reclaimed Land of Korea. Pathogens 2019, 8, 6.
- Lee, J.P.; Lee, S.W.; Kim, C.S.; Son, J.H.; Song, J.H.; Lee, K.Y.; Kim, H.J.; Jung, S.J.; Moon, B.J. Evaluation of Formulations of Bacillus Licheniformis for the Biological Control of Tomato Gray Mold Caused by Botrytis Cinerea. Biol. Control 2006, 37, 329–337.
- Tumbarski, Y.; Deseva, I.; Mihaylova, D.; Stoyanova, M.; Krastev, L.; Nikolova, R.; Yanakieva, V.; Ivanov, I. Isolation, Characterization and Amino Acid Composition of a Bacteriocin Produced by Bacillus Methylotrophicus Strain BM47. Food. Sci. Biotechnol. 2018, 56, 546–552.
- Abed, H.; Rouag, N.; Mouatassem, D.; Rouabhi, A. Screening for Pseudomonas and Bacillus Antagonistic Rhizobacteria Strains for the Biocontrol of Fusarium Wilt of Chickpea. Eurasian J. Soil Sci 2016, 5, 182–191.
- Yan, H.; Yun, J.; Ai, D.; Zhang, W.; Bai, J.; Guo, J. Two Novel Cationic Antifungal Peptides Isolated from Bacillus Pumilus HN-10 and Their Inhibitory Activity Against Trichothecium Roseum. World J. Microb. Biot. 2018, 34, 21.
- Wang, J.; Tian, H.; Kong, F.Y.; Wang, Y.H.; Zhang, C.S.; Feng, C. Inhibition of Bacillus Pumilus AR03 on Alternaria Alternata and Erysiphe Cichoracearum on Tobacco. J. Appl. Ecol 2015, 26, 3167–3173.
- Caulier, S.; Gillis, A.; Colau, G.; Licciardi, F.; Liépin, M.; Desoignies, N.; Modrie, P.; Legrève, A.; Mahillon, J.; Bragard, C. Versatile Antagonistic Activities of Soil-Borne Bacillus spp. and Pseudomonas spp. Against Phytophthora Infestans and Other Potato Pathogens. Front. Microbiol 2018, 9, 143.
- Nikolić, I.; Berić, T.; Dimkić, I.; Popović, T.; Lozo, J.; Fira, D.; Stanković, S. Biological Control of Pseudomonas Syringae pv. Aptata on Sugar Beet with Bacillus Pumilus SS-10.7 and Bacillus Amyloliquefaciens (SS-12.6 and SS-38.4) Strains. J. Appl. Microbiol 2019, 126, 165–176.
- Khan, N.; Martínez-Hidalgo, P.; Ice, T.A.; Maymon, M.; Humm, E.A.; Nejat, N.; Sanders, E.R.; Kaplan, D.; Hirsch, A.M. Antifungal Activity of Bacillus Species Against Fusarium and Analysis of the Potential Mechanisms Used in Biocontrol. Front. Microbial. 2018, 9, 2363.
- Sharma, R.; Chauhan, A.; Shirkot, C.K. Characterization of Plant Growth Promoting Bacillus Strains and Their Potential as Crop Protectants Against Phytophthora Capsici in Tomato. Biol. Agric. Hortic. 2015, 31, 230–244.
- Mnif, I.; Hammami, I.; Triki, M.A.; Azabou, M.C.; Ellouze-Chaabouni, S.; Ghribi, D. Antifungal Efficiency of a Lipopeptide Biosurfactant Derived from Bacillus Subtilis SPB1 Versus the Phytopathogenic Fungus. Fusarium Solani. Environ. Sci. Pollut. Res 2015, 22, 18137–18147.
- Ongena, M.; Jourdan, E.; Adam, A.; Paquot, M.; Brans, A.; Joris, B.; Arpigny, J.L.; Thonart, P. Surfactin and Fengycin Lipopeptides of Bacillus Subtilis as Elicitors of Induced Systemic Resistance in Plants. Environ. Microbiol 2007, 9, 1084–1090.
- Luo, W.; Liu, L.; Qi, G.; Yang, F.; Shi, X.; Zhao, X. Embedding Bacillus Velezensis NH-1 in Microcapsules for Biocontrol of Cucumber Fusarium Wilt. Appl. Environ. Microbiol 2019, 85, e03128–18.
- Wang, X.; Wang, J.; Jin, P.; Zheng, Y. Investigating the Efficacy of Bacillus Subtilis SM21 on Controlling Rhizopus rot in Peach Fruit. Int. J. Food Microbiol 2013, 164, 141–147.
- Xu, Z.; Xie, J.; Zhang, H.; Wang, D.; Shen, Q.; Zhang, R. Enhanced Control of Plant Wilt Disease by a Xylose-Inducible DegQ Gene Engineered Into Bacillus Velezensis Strain SQR9XYQ. Phytopathology 2019, 109, 36–43.
- Wambacq, E.; Audenaert, K.; Höfte, M.; De Saeger, S.; Haesaert, G.; Bacillus Velezensis as Antagonist Towards Penicillium Roqueforti sl in Silage: in Vitro and in Vivo Evaluation. J. Appl. Microbiol 2018, 125, 986–996.
- Wang, N.; Li, P.; Pan, J.; Wang, M.; Long, M.; Zang, J.; Yang, S. Bacillus Velezensis A2 Fermentation Exerts a Protective Effect on Renal Injury Induced by Zearalenone in Mice. Sci. Rep 2018, 8, 1–4.
- Ayed, H.B.; Azabou, M.C.; Hmidet, N.; Triki, M.A.; Nasri, M. Economic Production and Biocontrol Efficiency of Lipopeptide Biosurfactants from Bacillus Mojavenis A21. Biodegradation 2019, 30, 273–286.
- Daranas, N.; Roselló, G.; Cabrefiga, J.; Donati, I.; Francés, J.; Badosa, E.; Spinelli, F.; Montesinos, E.; Bonaterra, A. Biological Control of Bacterial Plant Diseases with Lactobacillus Plantarum Strains Selected for Their Broadspectrum Activity. Ann. Appl. Biol 2019, 174, 92–105.
- Song, J.; Zhang, J.H.; Kang, S.J.; Zhang, H.Y.; Yuan, J.; Zeng, C.Z.; Zhang, F.; Huang, Y.L. Analysis of Microbial Diversity in Apple Vinegar Fermentation Process Through 16s rDNA Sequencing. Food Sci. Nutr 2019, 7, 1230–1238.
- Du, N.; Shi, L.; Yuan, Y.; Sun, J.; Shu, S.; Guo, S. Isolation of a Potential Biocontrol Agent Paenibacillus Polymyxa NSY50 from Vinegar Waste Compost and its Induction of Host Defense Responses Against Fusarium Wilt of Cucumber. Microbiol. Res 2017, 202, 1–0.
- Shafi, J.; Tian, H.; Ji, M. Bacillus Species as Versatile Weapons for Plant Pathogens: a Review. Biotechnol. Biotechnol. Equip 2017, 31, 446–459.
- Edwards, M.G.; Gatehouse, A.M. Biotechnology in Crop Protection: Towards Sustainable Insect Control. In Novel Biotechnologies for Biocontrol Agent Enhancement and Management; Vurro, M., Gressel, J., Eds.; Springer: Dordrecht, 2007; pp. 1–23.
- Catarino, R.; Ceddia, G.; Areal, F.J.; Park, J. The Impact of Secondary Pests on Bacillus Thuringiensis (Bt) Crops. Plant Biotechnol. J 2015, 13, 601–612.
- Gerhardson, B. Biological Substitutes for Pesticides. Trends Biotechnol. 2002, 20, 338–343.
- Alfonzo, A.; Piccolo, S.L.; Conigliaro, G.; Ventorino, V.; Burruano, S.; Moschetti, G. Antifungal Peptides Produced by Bacillus Amyloliquefaciens AG1 Active Against Grapevine Fungal Pathogens. Ann. Microbiol 2012, 62, 1593–1599.
- Kumar, S.; Chandra, A.; Pandey, K.C. Bacillus Thuringiensis (Bt) Transgenic Crop: an Environment Friendly Insect-Pest Management Strategy. J. Environ. Biol 2008, 29, 641–653.
- Baragamaarachchi, R.Y.; Samarasekera, J.K.; Weerasena, O.V.; Lamour, K.; Jurat-Fuentes, J.L. Identification of a Native Bacillus Thuringiensis Strain from Sri Lanka Active Against Dipel-Resistant Plutella Xylostella. Peer J. 2019, 7, e7535.
- N’do, S.; Bayili, K.; Bayili, B.; Namountougou, M.; Sanou, R.; Ouattara, A.; Dabiré, R.K.; Malone, D.; Ouédraogo, A.G.; Borovsky, J.; Borovsky, D. Effect of Bacillus Thuringiensis var. Israelensis Sugar Patches on Insecticide Resistant Anopheles Gambiae sl Adults. J. Med.Entomol 2019, 56, 1312–1317.
- Zhao, G.H.; Liu, J.N.; Hu, X.H.; Batool, K.; Jin, L.; Wu, C.X.; Wu, J.; Chen, H.; Jiang, X.Y.; Yang, Z.H.; Huang, X.H. Cloning, Expression and Activity of ATP-Binding Protein in Bacillus Thuringiensis Toxicity Modulation Against Aedes Aegypti. Parasite Vector 2019, 12, 319.
- Geng, J.; Jiang, J.; Shu, C.; Wang, Z.; Song, F.; Geng, L.; Duan, J.; Zhang, J. Bacillus Thuringiensis Vip1 Functions as a Receptor of Vip2 Toxin for Binary Insecticidal Activity Against Holotrichia Parallela. Toxins. (Basel) 2019, 11, 440.
- Wang, Y.; Wang, J.; Fu, X.; Nageotte, J.R.; Silverman, J.; Bretsnyder, E.C.; Chen, D.; Rydel, T.J.; Bean, G.J.; Li, K.S.; Kraft, E. Bacillus Thuringiensis Cry1Da_7 and Cry1B. 868 Protein Interactions with Novel Receptors Allow Control of Resistant Fall Armyworms, Spodoptera Frugiperda (JE Smith). Appl. Environ. Microbiol 2019, 85, e00579–19.
- Sopko, M.S.; Narva, K.E.; Bowling, A.J.; Pence, H.E.; Hasler, J.J.; Letherer, T.J.; Larsen, C.M.; Zack, M.D. Modification of Vip3Ab1 C-Terminus Confers Broadened Plant Protection from Lepidopteran Pests. Toxins. (Basel) 2019, 11, 316.
- E Castro, B.M.D.C.; Martinez, L.C.; Barbosa, S.G.; Serrão, J.E.; Wilcken, C.F.; Soares, M.A.; da Silva, A.A.; de Carvalho, A.G.; Zanuncio, J.C. Toxicity and Cytopathology Mediated by Bacillusthuringiensis in the Midgut of Anticarsiagemmatalis (Lepidoptera: Noctuidae). Sci. Rep 2019, 9, 6667.
- Bowers, E.L. Fumonisin content in conventional versus Bt maize and implications of fumonisin contamination on fuel ethanol yield and DDGS quality.
- Abbas, H.K.; Zablotowicz, R.M.; Weaver, M.A.; Shier, W.T.; Bruns, H.A.; Bellaloui, N.; Accinelli, C.; Abel, C.A. Implications of Bt Traits on Mycotoxin Contamination in Maize: Overview and Recent Experimental Results in Southern United States. J. Agric. Food. Chem 2013, 61, 11759–11770.
- Gomaa, E.Z. Chitinase Production by Bacillus Thuringiensis and Bacillus Licheniformis: Their Potential in Antifungal Biocontrol. J. Microbiol 2012, 50, 103–111.
- Harun-Or-Rashid, M.; Kim, H.J.; Yeom, S.I.; Yu, H.A.; Manir, M.; Moon, S.S.; Kang, Y.J.; Chung, Y.R. Bacillus Velezensis YC7010 Enhances Plant Defenses Against Brown Planthopper Through Transcriptomic and Metabolic Changes in Rice. Front. Plant Sci 2018, 9, 1904.
- Sweeney, M.J.; Dobson, A.D. Mycotoxin Production by Aspergillus, Fusarium and Penicillium Species. Int. J. Food Microbiol 1998, 43, 141–158.
- Bennett, J.W. Mycotoxins, Mycotoxicoses, Mycotoxicology and Mycopathologia. Mycopathologia 1987, 100, 3–5.
- Kensler, T.W.; Roebuck, B.D.; Wogan, G.N.; Groopman, J.D. Aflatoxin: a 50-Year Odyssey of Mechanistic and Translational Toxicology. Toxicol. Sci 2011, 120 (suppl_1), S28–48.
- Kamei, K.; Watanabe, A. Aspergillus Mycotoxins and Their Effect on the Host. Med. Mycol. 2005, 43 (sup1), 95–99.
- Lewis, L.; Onsongo, M.; Njapau, H.; Schurz-Rogers, H.; Luber, G.; Kieszak, S.; Nyamongo, J.; Backer, L.; Dahiye, A.M.; Misore, A.; DeCock, K. Aflatoxin Contamination of Commercial Maize Products During an Outbreak of Acute Aflatoxicosis in Eastern and Central Kenya. Environ. Health Perspect 2005, 113, 1763–1767.
- Wild, C.P.; Miller, J.D.; Groopman, J.D. Eds. Mycotoxin Control in low-and Middle-Income Countries; International Agency for Research on Cancer: Lyon, France, 2015.
- Ringot, D.; Chango, A.; Schneider, Y.J.; Larondelle, Y. Toxicokinetics and Toxicodynamics of Ochratoxin A, an Update. Chem.-Biol. Interact. 2006, 159 (1), 18–46.
- Peraica, M.; Radić, B.; Lucić, A.; Pavlović, M. Toxic Effects of Mycotoxins in Humans. Bull. W. H. O. 1999, 77, 754–766.
- Wang, J.Q.; Yang, F.; Yang, P.L.; Liu, J.; Lv, Z.H. Microbial Reduction of Zearalenone by a new Isolated Lysinibacillus sp. ZJ-2016-1. World Mycotoxin J. 2018, 11, 571–578.
- Ciegler, A.; Lillehoj, E.B.; Peterson, R.E.; Hall, H.H. Microbial Detoxification of Aflatoxin. Appl. Environ. Microbiol 1966, 14, 934–939.
- Adebo, O.A.; Njobeh, P.B.; Mavumengwana, V. Degradation and Detoxification of AFB1 by Staphylocococcus Warneri, Sporosarcina sp. and Lysinibacillus Fusiformis. Food Control 2016, 68, 92–96.
- Verheecke, C.; Liboz, T.; Mathieu, F. Microbial Degradation of Aflatoxin B1: Current Status and Future Advances. Int. J. Food Microbiol 2016, 237, 1–9.
- Vanhoutte, I.; Audenaert, K.; De Gelder, L. Biodegradation of Mycotoxins: Tales from Known and Unexplored Worlds. Front. Microbiology 2016, 7, 561.
- Fu, G.; Ma, J.; Wang, L.; Yang, X.; Liu, J.; Zhao, X. Effect of Degradation of Zearalenone-Contaminated Feed by Bacillus Licheniformis CK1 on Postweaning Female Piglets. Toxins. (Basel) 2016, 8, 300.
- Chalivendra, S.; Ham, J.H. Bacilli in the Biocontrol of Mycotoxins. In: Bacilli and Agrobiotechnology: Phytostimulationand Biocontrol, Bacilli in Climate Resilient Agriculture and Bioprospecting; Islam, M.T. et al., Ed.; Springer International: Switzerland AG, 2019; pp. 49–62.
- Hassall, C.H.; Subtilin, C. An Antibiotic Concentrate from Bacillus Subtilis. Nature 1948, 161, 317–318.
- DeFilippi, S.; Groulx, E.; Megalla, M.; Mohamed, R.; Avis, T.J. Fungal Competitors Affect Production of Antimicrobial Lipopeptides in Bacillus Subtilis Strain B9–5. J. Chem. Ecol 2018, 44, 374–383.
- Ongena, M.; Jacques, P. Bacillus Lipopeptides: Versatile Weapons for Plant Disease Biocontrol. Trends Microbiol. 2008, 16, 115–125.
- Leclère, V.; Marti, R.; Béchet, M.; Fickers, P.; Jacques, P. The Lipopeptides Mycosubtilin and Surfactin Enhance Spreading of Bacillus Subtilis Strains by Their Surface-Active Properties. Arch. Microbiol 2006, 186, 475–483.
- Hu, X.; Roberts, D.P.; Xie, L.; Maul, J.E.; Yu, C.; Li, Y.; Zhang, S.; Liao, X. Bacillus Megaterium A6 Suppresses Sclerotinia Sclerotiorum on Oilseed Rape in the Field and Promotes Oilseed Rape Growth. Crop Prot. 2013, 52, 151–158.
- Farzaneh, M.; Shi, Z.Q.; Ahmadzadeh, M.; Hu, L.B.; Ghassempour, A. Inhibition of the Aspergillus Flavus Growth and Aflatoxin B1 Contamination on Pistachio nut by Fengycin and Surfactin-Producing Bacillus Subtilis UTBSP1. PlantPathol. J. 2016, 32, 209.
- Hanif, A.; Zhang, F.; Li, P.; Li, C.; Xu, Y.; Zubair, M.; Zhang, M.; Jia, D.; Zhao, X.; Liang, J.; Majid, T. Fengycin Produced by Bacillus Amyloliquefaciens FZB42 Inhibits Fusarium Graminearum Growth and Mycotoxins Biosynthesis. Toxins. (Basel) 2019, 11, 295.
- Gong, Q.; Zhang, C.; Lu, F.; Zhao, H.; Bie, X.; Lu, Z. Identification of Bacillomycin D from Bacillus Subtilis FmbJ and its Inhibition Effects Against Aspergillus Flavus. Food Control 2014, 36, 8–14.
- Sun, J.; Li, W.; Liu, Y.; Lin, F.; Huang, Z.; Lu, F.; Bie, X.; Lu, Z. Growth Inhibition of Fusarium Graminearum and Reduction of Deoxynivalenol Production in Wheat Grain by Bacillomycin D. J. Stored Prod. Res 2018, 75, 21–28.
- Xu, Z.; Shao, J.; Li, B.; Yan, X.; Shen, Q.; Zhang, R. Contribution of Bacillomycin D in Bacillus Amyloliquefaciens SQR9 to Antifungal Activity and Biofilm Formation. Appl. Environ. Microbiol 2013, 79, 808–815.
- Romero, D.; de Vicente, A.; Rakotoaly, R.H.; Dufour, S.E.; Veening, J.W.; Arrebola, E.; Cazorla, F.M.; Kuipers, O.P.; Paquot, M.; Pérez-García, A. The Iturin and Fengycin Families of Lipopeptides are key Factors in Antagonism of Bacillus Subtilis Toward Podosphaera Fusca. Mol. Plant-Microbe. Interact 2007, 20, 430–440.
- Ahmad, Z.; Wu, J.; Chen, L.; Dong, W. Isolated Bacillus Subtilis Strain 330-2 and its Antagonistic Genes Identified by the Removing PCR. Sci. Rep. 2017, 11 (7), 1–3.
- Bais, H.P.; Fall, R.; Vivanco, J.M. Biocontrol of Bacillus Subtilis Against Infection of Arabidopsis Roots by Pseudomonas Syringae is Facilitated by Biofilm Formation and Surfactin Production. Plant Physiol. 2004, 134, 307–319.
- Hegarty, J.W.; Guinane, C.M.; Ross, R.P.; Hill, C.; Cotter, P.D. Bacteriocin Production: a Relatively Unharnessed Probiotic Trait? F1000Res 2016, 5, 2587
- Tareq, F.S.; Lee, M.A.; Lee, H.S.; Lee, Y.J.; Lee, J.S.; Hasan, C.M.; Islam, M.T.; Shin, H.J. Gageotetrins A–C, Noncytotoxic Antimicrobial Linear Lipopeptides from a Marine Bacterium Bacillus Subtilis. Org. Lett. 2014, 7, 928–931.
- Chakraborty, M.; Mahmud, N.U.; Gupta, D.R.; Tareq, F.S.; Shin, H.J.; Islam, T. Inhibitory Effects of Linear Lipopeptides from Amarine Bacillus Subtilis on the Wheat Blast Fungus Magnaporthe Oryzae Triticum. Front. Microbiol. 2020. doi:10.3389/fmicb.2020.00665
- Ravu, R.R.; Jacob, M.R.; Chen, X.; Wang, M.; Nasrin, S.; Kloepper, J.W.; Liles, M.R.; Mead, D.A.; Khan, I.A.; Li, X.C. Bacillusin A, an Antibacterial Macrodiolide from Bacillus Amyloliquefaciens AP183. J. Nat. Prod 2015, 78, 924–928.
- Romero-Tabarez, M.; Jansen, R.; Sylla, M.; Lünsdorf, H.; Häußler, S.; Santosa, D.A.; Timmis, K.N.; Molinari, G. 7-O-malonyl Macrolactin A, a new Macrolactin Antibiotic from Bacillus Subtilis Active Against Methicillin-Resistant Staphylococcus Aureus, Vancomycin-Resistant Enterococci, and a Small-Colony Variant of Burkholderia Cepacia. Antimicrob. Agents Chemother 2006, 50, 1701–1709.
- Ma, Z.; Hu, J. Plipastatin A1 Produced by a Marine Sediment-Derived Bacillus Amyloliquefaciens SH-B74 Contributes to the Control of Gray Mold Disease in Tomato. 3. Biotech. 2018, 8, 125.
- Chakraborty, K.; Thilakan, B.; Raola, V.K. Antimicrobial Polyketide Furanoterpenoids from Seaweed-Associated Heterotrophic Bacterium Bacillus Subtilis MTCC 10403. Phytochemistry 2017, 142, 112–125.
- Li, D.; Carr, G.; Zhang, Y.; Williams, D.E.; Amlani, A.; Bottriell, H.; Mui, A.L.; Andersen, R.J. Turnagainolides A and B, Cyclic Depsipeptides Produced in Culture by a Bacillus sp.: Isolation, Structure Elucidation, and Synthesis. J. Nat. Prod 2011, 74, 1093–1099.
- Xie, C.L.; Xia, J.M.; Su, R.Q.; Li, J.; Liu, Y.; Yang, X.W.; Yang, Q. Bacilsubteramide A, a new Indole Alkaloid, from the Deep-sea-Derived Bacillus Subterraneus 11593 Nat. Prod.Res 2018, 32, 2553–2557.
- Pinzón-Espinosa, A.; Martinez-Matamoros, D.; Castellanos, L.; Duque, C.; Rodríguez, J.; Jiménez, C.; Ramos, F.A. Cereusitin A, a Cyclic Tetrapeptide from a Bacillus Cereus Strain Isolated from the Soft Coral Antillogorgia (syn. Pseudopterogorgia) Elisabethae. Tetrahedron Lett. 2017, 58, 634–637.
- Jin, P.; Wang, H.; Liu, W.; Fan, Y.; Miao, W. A new Cyclic Lipopeptide Isolated from Bacillus Amyloliquefaciens HAB-2 and Safety Evaluation. Pestic Biochem Phys 2018, 147, 40–45.
- Anjum, K.; Bi, H.; Chai, W.; Lian, X.Y.; Zhang, Z. Antiglioma Pseurotin A from Marine Bacillus sp. FS8D Regulating Tumour Metabolic Enzymes. Nat. Prod. Res. 2018, 32, 1353–1356.
- Le Marrec, C.; Hyronimus, B.; Bressollier, P.; Verneuil, B.; Urdaci, M.C. Biochemical and Genetic Characterization of Coagulin, a new Antilisterial Bacteriocin in the Pediocin Family of Bacteriocins, Produced by Bacillus Coagulans I4. Appl. Environ. Microbiol 2000, 66, 5213–5220.
- Kamoun, F.; Mejdoub, H.; Aouissaoui, H.; Reinbolt, J.; Hammami, A.; Jaoua, S. Purification, Amino Acid Sequence and Characterization of Bacthuricin F4, a new Bacteriocin Produced by Bacillus Thuringiensis. J. Appl. Microbiol 2005, 98, 881–888.
- Bizani, D.; Dominguez, A.P.; Brandelli, A. Purification and Partial Chemical Characterization of the Antimicrobial Peptide Cerein 8A. Lett. Appl. Microbiol 2005, 41, 269–273.
- Lisboa, M.P.; Bonatto, D.; Bizani, D.; Henriques, J.A.; Brandelli, A. Characterization of a Bacteriocin-Like Substance Produced by Bacillus Amyloliquefaciens Isolated from the Brazilian Atlantic Forest. Int. Microbiol 2006, 9, 111–118.
- Chehimi, S.; Delalande, F.; Sable, S.; Hajlaoui, M.R.; Van Dorsselaer, A.; Limam, F.; Pons, A.M. Purification and Partial Amino Acid Sequence of Thuricin S, a new Anti-Listeria Bacteriocin from Bacillus Thuringiensis. Can. J. Microbiol 2007, 53, 284–290.
- Kalinovskaya, N.I.; Kuznetsova, T.A.; Ivanova, E.P.; Romanenko, L.A.; Voinov, V.G.; Huth, F.; Laatsch, H. Characterization of Surfactin-Like Cyclic Depsipeptides Synthesized by Bacillus Pumilus from Ascidian Halocynthia Aurantium. Mar. Biotechnol 2002, 4, 179–188.
- Steinborn, G.; Hajirezaei, M.R.; Hofemeister, J. bac Genes for Recombinant Bacilysin and Anticapsin Production in Bacillus Host Strains. Arch. Microbiol 2005, 183, 71–79.
- Tamehiro, N.; Okamoto-Hosoya, Y.; Okamoto, S.; Ubukata, M.; Hamada, M.; Naganawa, H.; Ochi, K. Bacilysocin, a Novel Phospholipid Antibiotic Produced by Bacillus Subtilis 168. Antimicrob. Agents Chemother 2002, 46, 315–320.
- Gupta, R.; Ramnani, P. Microbial Keratinases and Their Prospective Applications: an Overview. Appl. Microbial. Biotechnol. 2006, 70, 21.
- Siezen, R.J.; Leunissen, J.A. Subtilases: the Superfamily of Subtilisin-Like Serine Proteases. Protein Sci. 1997, 6, 501–523.
- Sharma, V.; Ayothiraman, S.; Dhakshinamoorthy, V. Production of Highly Thermo-Tolerant Laccase from Novel Thermophilic Bacterium Bacillus sp. PC-3 and its Application in Functionalization of Chitosan Film. J. Biosc. Bioeng 2019, 127, 672–678.
- Wang, K.; Yan, P.S.; Ding, Q.L.; Wu, Q.X.; Wang, Z.B.; Peng, J. Diversity of Culturable Root-Associated/Endophytic Bacteria and Their Chitinolytic and Aflatoxin Inhibition Activity of Peanut Plant in China. World J. Microbiol. Biotechnol 2013, 29, 1–10.
- Israni, N.; Thapa, S.; Shivakumar, S. Biolytic Extraction of Poly (3-Hydroxybutyrate) from Bacillus Megaterium Ti3 Using the Lytic Enzyme of Streptomyces Albus Tia1. J. Gen. Eng. and Biot 2018, 16, 265–271.
- Riyami, B.A.; Ghosh, A.; Rees, E.J.; Christie, G. Novel Cortex Lytic Enzymes in Bacillus Megaterium QM B1551 Spores. FEMS Microbial. Lett 2019, 366, fnz146.
- Hong, S.; Son, B.; Ryu, S.; Ha, N.C. Crystal Structure of LysB4, an Endolysin from Bacillus Cereus-Targeting Bacteriophage B4. Mol. Cells 2019, 42, 79.
- Muslim, S.N.; AL-Kadmy, I.M.; Hussein, N.H.; Ali, A.N.; Taha, B.M.; Aziz, S.N.; Al Kheraif, A.A.; Divakar, D.D.; Ramakrishnaiah, R. Chitosanase Purified from Bacterial Isolate Bacillus Licheniformis of Ruined Vegetables Displays Broad Spectrum Biofilm Inhibition. Microb. Pathog 2016, 100, 257–162.
- Leelasuphakul, W.; Sivanunsakul, P.; Phongpaichit, S. Purification, Characterization and Synergistic Activity of β-1, 3-Glucanase and Antibiotic Extract from an Antagonistic Bacillus Subtilis NSRS 89-24 Against Rice Blast and Sheath Blight. Enzyme Microbiol. Technol. 2006, 38, 990–997.
- Jayaraj, J.; Yi, H.; Liang, G.H.; Muthukrishnan, S.; Velazhahan, R. Foliar Application of Bacillus Subtilis AUBS1 Reduces Sheath Blight and Triggers Defense Mechanisms in Rice. J. Plant Dis. Protect 2004, 111, 115–125.
- Kloepper, J.W.; Ryu, C.M.; Zhang, S. Induced Systemic Resistance and Promotion of Plant Growth by Bacillusspp. Phytopathology 2004, 94, 1259–1266.
- Van der Ent, S.; Verhagen, B.W.; Van Doorn, R.; Bakker, D.; Verlaan, M.G.; Pel, M.J.; Joosten, R.G.; Proveniers, M.C.; Van Loon, L.C.; Ton, J.; Pieterse, C.M. MYB72 is Required in Early Signaling Steps of Rhizobacteria-Induced Systemic Resistance in Arabidopsis. Plant Physiol. 2008, 146, 1293–1304.
- Jones, J.D.; Dangl, J.L. The Plant Immune System. Nature 2006, 444, 323.
- Toure, Y.; Ongena, M.A.; Jacques, P.; Guiro, A.; Thonart, P. Role of Lipopeptides Produced by Bacillus Subtilis GA1 in the Reduction of Grey Mould Disease Caused by Botrytis Cinerea on Apple. J. Appl. Microbiol 2004, 96 (5), 1151–1160.
- Yoshida, S.; Koitabashi, M.; Yaginuma, D.; Anzai, M.; Fukuda, M. Potential of Bioinsecticidal Bacillus Thuringiensis Inoculum to Suppress Gray Mold in Tomato Based on Induced Systemic Resistance. J. Phytopathol 2019, 167, 679–685.
- Hossain, M.T.; Chung, Y.R. Endophytic Bacillus Species Induce Systemic Resistance to Plant Diseases. In Bacilli and Agrobiotechnology: Phytostimulation and Biocontrol; Islam, M.T., et al. Ed.; Springer: Cham, 2019; pp. 151–160.
- Rutherford, S.T.; Bassler, B.L. Bacterial Quorum Sensing: its Role in Virulence and Possibilities for its Control. CSH. Perspect. Med 2012, 2, a012427.
- Kaplan, H.B.; Greenberg, E.P. Diffusion of Autoinducer is Involved in Regulation of the Vibrio Fischeri Luminescence System. J. Bacteriol. 1985, 163, 1210–1214.
- Novick, R.P.; Projan, S.J.; Kornblum, J.; Ross, H.F.; Ji, G.; Kreiswirth, B.; Vandenesch, F.; Moghazeh, S. Theagr P2 Operon: An Autocatalytic Sensory Transduction System in Staphylococcus Aureus. Mol. Gen. Genet 1995, 248, 446–458.
- Seed, P.C.; Passador, L.; Iglewski, B.H. Activation of the Pseudomonas Aeruginosa LasI Gene by LasR and the Pseudomonas Autoinducer PAI: An Autoinduction Regulatory Hierarchy. J. Bacteriol. 1995, 177, 654–659.
- Vlamakis, H.; Chai, Y.; Beauregard, P.; Losick, R.; Kolter, R. Sticking Together: Building a Biofilm the Bacillus Subtilis way. Nat. Rev. Microbiol 2013, 11, 157–168.
- Beauregard, P.B.; Chai, Y.; Vlamakis, H.; Losick, R.; Kolter, R. Bacillus Subtilis Biofilm Induction by Plant Polysaccharides. Proc. Natl. Acad. Sci 2013, 110, E1621–30.
- Persello-Cartieaux, F.; Nussaume, L.; Robaglia, C. Tales from the Underground: Molecular Plant–Rhizobacteria Interactions. Plant Cell Environ. 2003, 26, 189–199.
- Rahman, M.; Sabir, A.A.; Mukta, J.A.; Khan, M.M.; Mohi-Ud-Din, M.; Miah, M.G.; Rahman, M.; Islam, M.T. Plant Probiotic Bacteria Bacillus and Paraburkholderia Improve Growth, Yield and Content of Antioxidants in Strawberry Fruit. Sci. Rep 2018, 8, 1–1.
- López-Bucio, J.; Campos-Cuevas, J.C.; Hernández-Calderón, E.; Velásquez-Becerra, C.; Farías-Rodríguez, R.; Macías-Rodríguez, L.I.; Valencia-Cantero, E. Bacillus Megaterium Rhizobacteria Promote Growth and Alter Root-System Architecture Through an Auxin-and Ethylene-Independent Signaling Mechanism in Arabidopsis Thaliana. Mol. Plant-Microbe Interact 2007, 20, 207–217.
- Qiao, J.; Yu, X.; Liang, X.; Liu, Y.; Borriss, R.; Liu, Y. Addition of Plant-Growth-Promoting Bacillus Subtilis PTS-394 on Tomato Rhizosphere has no Durable Impact on Composition of Root Microbiome. BMC Microbiol. 2017, 17, 131.
- Gowtham, H.G.; Murali, M.; Singh, S.B.; Lakshmeesha, T.R.; Murthy, K.N.; Amruthesh, K.N.; Niranjana, S.R. Plant Growth Promoting Rhizobacteria-Bacillus Amyloliquefaciens Improves Plant Growth and Induces Resistance in Chilli Against Anthracnose Disease. Biol. Control 2018, 126, 209–217.
- Shrestha, B.K.; Karki, H.S.; Groth, D.E.; Jungkhun, N.; Ham, J.H. Biological Control Activities of Rice-Associated Bacillus sp. Strains Against Sheath Blight and Bacterial Panicle Blight of Rice. PloS One 2016, 11, e0146764. DOI:10.1371/journal.pone.0146764.
- Park, J.W.; Balaraju, K.; Kim, J.W.; Lee, S.W.; Park, K. Systemic Resistance and Growth Promotion of Chili Pepper Induced by an Antibiotic Producing Bacillus Vallismortis Strain BS07. Biol.Control. 2013, 65, 246–257.
- Anand, R.; Chanway, C. N 2-Fixation and Growth Promotion in Cedar Colonized by an Endophytic Strain of Paenibacillus Polymyxa. Biol. Fert. Soils 2013, 49, 235–239.
- Sgroy, V.; Cassán, F.; Masciarelli, O.; Del Papa, M.F.; Lagares, A.; Luna, V. Isolation and Characterization of Endophytic Plant Growth-Promoting (PGPB) or Stress Homeostasis-Regulating (PSHB) Bacteria Associated to the Halophyte Prosopisstrombulifera. Appl. Microbiol. Biotechnol 2009, 85, 371–381.
- Olszewski, N.; Sun, T.P.; Gubler, F. Gibberellin Signaling: Biosynthesis, Catabolism, and Response Pathways. Plant Cell 2002, 14, 61–S80.
- Schmulling, T. New Insights Into the Functions of Cytokinins in Plant Development. J. Plant Growth Regul 2002, 21, 40–49.
- Asgher, M.; Khan, M.I.; Anjum, N.A.; Khan, N.A. Minimising Toxicity of Cadmium in Plants—Role of Plant Growth Regulators. Protoplasma 2015, 252, 399–413.
- Großkinsky, D.K.; van der Graaff, E.; Roitsch, T.. Regulation of Abiotic and Biotic Stress Responses by Plant Hormones. In Plant Pathogen Resistance Biotechnology; Collinge, D.B., Ed.; 2016; pp. 131–154.
- Park, Y.G.; Mun, B.G.; Kang, S.M.; Hussain, A.; Shahzad, R.; Seo, C.W.; Kim, A.Y.; Lee, S.U.; Oh, K.Y.; Lee, D.Y.; Lee, I.J. Bacillus Aryabhattai SRB02 Tolerates Oxidative and Nitrosative Stress and Promotes the Growth of Soybean by Modulating the Production of Phytohormones. PLoS One 2017, 12 (3), e0173203. DOI:10.1371/journal.pone.0173203.
- de OLIVEIRA, A.L.; Urquiaga, S.; Baldani, J.I. Processos e mecanismos envolvidos na influência de microrganismos sobre o crescimento vegetal. Embrapa Agrobiologia-Documentos (INFOTECA-E); 2003.
- Jha, B.; Gontia, I.; Hartmann, A. The Roots of the Halophyte Salicornia Brachiata are a Source of new Halotolerant Diazotrophic Bacteria with Plant Growth-Promoting Potential. Plant Soil 2012, 356, 265–277.
- Ishak, Z.; Mohd Iswadi, M.K.; Russman Nizam, A.H.; Ahmad Kamil, M.J.; Ernie Eileen, R.R.; Wan Syaidatul, A.; Ainon, H. Plant Growth Hormones Produced by Endophytic Bacillus Subtilis Strain LKM-BK Isolated from Cocoa. Malays Cocoa J 2016, 9, 127–133.
- Singh, R.P.; Jha, P.N. A Halotolerant Bacterium Bacillus Licheniformis HSW-16 Augments Induced Systemic Tolerance to Salt Stress in Wheat Plant (Triticum Aestivum). Front. Plant Sci. 2016, 7, 1890.
- Upadhyay, S.K.; Singh, J.S.; Saxena, A.K.; Singh, D.P. Impact of PGPR Inoculation on Growth and Antioxidant Status of Wheat Under Saline Conditions. Plant Biology 2012, 14, 605–611.
- Hashem, A.; Abd_Allah, E.F.; Alqarawi, A.; Al-Huqail, A.A.; Wirth, S.; Egamberdieva, D. The Interaction Between Arbuscular Mycorrhizal Fungi and Endophytic Bacteria Enhances Plant Growth of Acacia Gerrardii Under Salt Stress. Front. Plant Sci 2016, 7, 1089.
- Zaidi, S.; Usmani, S.; Singh, B.R.; Musarrat, J. Significance of Bacillus Subtilis Strain SJ-101 as a Bioinoculant for Concurrent Plant Growth Promotion and Nickel Accumulation in Brassica Juncea. Chemosphere 2006, 64, 991–997.
- Liu, F.; Xing, S.; Ma, H.; Du, Z.; Ma, B. Cytokinin-producing, Plant Growth-Promoting Rhizobacteria That Confer Resistance to Drought Stress in Platycladus Orientalis Container Seedlings. Appl. Microbiol. Biotech 2013, 97, 9155–9164.
- Shahzad, R.; Khan, A.L.; Bilal, S.; Waqas, M.; Kang, S.M.; Lee, I.J. Inoculation of Abscisic Acid-Producing Endophytic Bacteria Enhances Salinity Stress Tolerance in Oryza Sativa. Environ. Exp. Bot. 2017, 136, 68–77.
- Egamberdieva, D.; Wirth, S.J.; Shurigin, V.V.; Hashem, A.; AbdAllah, E.F. Endophytic Bacteria Improve Plant Growth, Symbiotic Performance of Chickpea (Cicer Arietinum L.) and Induce Suppression of Root rot Caused by Fusarium Solani Under Salt Stress. Front. Microbiol 2017, 8, 1887.
- Glick, BR. Plant Growth-Promoting Bacteria: Mechanisms and Applications. Scientifica. (Cairo). 2012. doi:10.6064/2012/963401
- Kalayu, G. Phosphate Solubilizing Microorganisms: Promising Approach as Biofertilizers. Int. J. Agro. 2019. doi:10.1155/2019/4917256.
- Wahab, A.A. Nitrogen Fixation by Bacillus Strains Isolated from the Rhizosphere of Ammophila Arenaria. Plant Soil 1975, 42, 703–708.
- Pramanik, P.; Goswami, A.J.; Ghosh, S.; Kalita, C. An Indigenous Strain of Potassium-Solubilizing Bacteria Bacillus Pseudomycoides Enhanced Potassium Uptake in tea Plants by Increasing Potassium Availability in the Mica Waste-Treated Soil of North-East India. J. Appl. Microbiol 2019, 126, 215–222.
- FAO. http://www.fao.org/3/a-i6030e.pdf (2016).
- Di Benedetto, N.A.; Corbo, M.R.; Campaniello, D.; Cataldi, M.P.; Bevilacqua, A.; Sinigaglia, M.; Flagella, Z. The Role of Plant Growth Promoting Bacteria in Improving Nitrogen use Efficiency for Sustainable Crop Production: a Focus on Wheat. AIMS Microbiol 2017, 3, 413.
- Chauhan, P.S.; Lata, C.; Tiwari, S.; Chauhan, A.S.; Mishra, S.K.; Agrawal, L.; Chakrabarty, D.; Nautiyal, C.S. Transcriptional Alterations Reveal Bacillus Amyloliquefaciens-Rice Cooperation Under Salt Stress. Sci. Reports 2019, 9, 1–3.
- Tiwari, S.; Shweta, S.; Prasad, M.; Lata, C. Genome-wide Investigation of GRAM-Domain Containing Genes in Rice Reveals Their Role in Plant-Rhizobacteria Interactions and Abiotic Stress Responses. Int. J. Biol. Macromol 2020, 156, 1243–1257.
- FAO. http://www.fao.org/ news/story/ en/item/273303/icode (2014).
- FAO. Food losses and waste. (2015) URL http://www.fao.org/food-loss-and-food-waste/en/.
- Araus, J.L.; Slafer, G.A.; Royo, C.; Serret, M.D. Breeding for Yield Potential and Stress Adaptation in Cereals. Crit. Rev. Plant Sci 2008, 27, 377–412.
- Araus, J.L.; Slafer, G.A.; Reynolds, M.P.; Royo, C. Plant Breeding and Drought in C3 Cereals: What Should we Breed for? Ann. Bot 2002, 89, 925–940.
- Ruan, Y.L.; Jin, Y.; Yang, Y.J.; Li, G.J.; Boyer, J.S. Sugar Input, Metabolism, and Signaling Mediated by Invertase: Roles in Development, Yield Potential, and Response to Drought and Heat. Mol. Plant 2010, 3, 942–955.
- Krasensky, J.; Jonak, C. Drought, Salt, and Temperature Stress-Induced Metabolic Rearrangements and Regulatory Networks. J. Exp. Bot 2012, 63, 1593–1608.
- Pereira, A. Plant Abiotic Stress Challenges from the Changing Environment. Front. Plant Sci 2016, 7, 1123.
- Potters, G.; Pasternak, T.P.; Guisez, Y.; Palme, K.J.; Jansen, M.A. Stress-induced Morphogenic Responses: Growing out of Trouble? Trends Plant Sci. 2007, 12, 98–105.
- Huang, D.; Wu, W.; Abrams, S.R.; Cutler, A.J. The Relationship of Drought-Related Gene Expression in Arabidopsis Thaliana to Hormonal and Environmental Factors. J. Exp. Bot 2008, 59, 2991–3007.
- Munns, R.; Tester, M. Mechanisms of Salinity Tolerance. Annu. Rev. Plant Biol 2008, 59, 651–681.
- Turan, M.; Gulluce, M.; Şahin, F. Effects of Plant-Growth-Promoting Rhizobacteria on Yield, Growth, and Some Physiological Characteristics of Wheat and Barley Plants. Commun. Soil Sci. Plant Analysis 2012, 43, 1658–1673.
- Wani, P.A.; Wahid, S.; Singh, R.; Kehinde, A.M. Antioxidant and Chromium Reductase Assisted Chromium (VI) Reduction and Cr (III) Immobilization by the Rhizospheric Bacillus Helps in the Remediation of Cr (VI) and Growth Promotion of Soybean Crop. Rhizosphere 2018, 6, 23–30.
- Niu, D.D.; Liu, H.X.; Jiang, C.H.; Wang, Y.P.; Wang, Q.Y.; Jin, H.L.; Guo, J.H. The Plant Growth–Promoting Rhizobacterium Bacillus Cereus AR156 Induces Systemic Resistance in Arabidopsis Thaliana by Simultaneously Activating Salicylate-and Jasmonate/Ethylene-Dependent Signaling Pathways. Mol. Plant-Microbe Interact 2011, 24, 533–542.
- Khan, N.; Bano, A.; Rahman, M.A.; Guo, J.; Kang, Z.; Babar, M.A. Comparative Physiological and Metabolic Analysis Reveals a Complex Mechanism Involved in Drought Tolerance in Chickpea (Cicer Arietinum L.) Induced by PGPR and PGRs. Sci. Rep 2019, 9, 1–9.
- Wang, D.C.; Jiang, C.H.; Zhang, L.N.; Chen, L.; Zhang, X.Y.; Guo, J.H. Biofilms Positively Contribute to Bacillus Amyloliquefaciens 54-Induced Drought Tolerance in Tomato Plants. Int. J. Mol. Sci. 2019, 20, 6271.
- Pitman, M.G.; Läuchli, A. Global Impact of Salinity and Agricultural Ecosystems. In Salinity: Environment-Plants-Molecules; Luechli, A., Lttg, U., Eds.; Springer: Dordrecht, 2002; pp. 3–20.
- Hasegawa, P.M.; Bressan, R.A.; Zhu, J.K.; Bohnert, H.J. Plant Cellular and Molecular Responses to High Salinity. Annu. Rev. Plant Biol 2000, 51, 463–499.
- Tejera, N.A.; Campos, R.; Sanjuan, J.; Lluch, C. Nitrogenase and Antioxidant Enzyme Activities in Phaseolus Vulgaris Nodules Formed by Rhizobium Tropici Isogenic Strains with Varying Tolerance to Salt Stress. J. Plant Physiol 2004, 161, 329–338.
- Chen, H.; Jiang, J.G. Osmotic Adjustment and Plant Adaptation to Environmental Changes Related to Drought and Salinity. Environ. Rev 2010, 18, 309–319.
- Bochow, H.; El-Sayed, S.F., Junge, H.; Stavropoulou, A.; Schmiedeknecht, G. Use of Bacillus subtilis as biocontrol agent. IV. Salt-stress tolerance induction by Bacillus subtilis FZB24 seed treatment in tropical vegetable field crops, and its mode of action/Die Verwendung von Bacillus subtilis zur biologischen Bekämpfung. IV. Induktion einer Salzstress-Toleranz durch Applikation von Bacillus subtilis FZB24 bei tropischem Feldgemüse und sein Wirkungsmechanismus. Zeitschrift für Pflanzenkrankheiten und Pflanzenschutz/Journal of Plant Diseases and Protection. 2001, 21-30.
- Ashraf, M.; Hasnain, S.; Berge, O.; Mahmood, T. Inoculating Wheat Seedlings with Exopolysaccharide-Producing Bacteria Restricts Sodium Uptake and Stimulates Plant Growth Under Salt Stress. Biol. Fert. Soils 2004, 40, 157–162.
- Saleh, S.A.; Heuberger, H.; Schnitzler, W.H. Alleviation of Salinity Effect on Artichoke Productivity by Bacillus Subtilis FZB24, Supplemental Ca and Micronutrients. J. Appl. Bot. Food Qual 2005, 79, 24–32.
- Rontein, D.; Basset, G.; Hanson, A.D. Metabolic Engineering of Osmoprotectant Accumulation in Plants. Metab. Eng 2002, 4, 49–56.
- Hare, P.D.; Cress, W.A.; Van Staden, J. Dissecting the Roles of Osmolyte Accumulation During Stress. Plant Cell Environ. 1998, 21, 535–553.
- del Carmen Orozco-Mosqueda, M.; Glick, B.R.; Santoyo, G. ACC Deaminase in Plant Growth-Promoting Bacteria (PGPB): an Efficient Mechanism to Counter Salt Stress in Crops. Microbiol. Res. 2020, 15, 126439.
- Subramanian, P.; Kim, K.; Krishnamoorthy, R.; Mageswari, A.; Selvakumar, G.; Sa, T. Cold Stress Tolerance in Psychrotolerant Soil Bacteria and Their Conferred Chilling Resistance in Tomato (Solanum Lycopersicum Mill.) Under low Temperatures. PloS One 2016, 11, e0161592.
- Zubair, M.; Hanif, A.; Farzand, A.; Sheikh, T.M.; Khan, A.R.; Suleman, M.; Ayaz, M.; Gao, X. Genetic Screening and Expression Analysis of Psychrophilic Bacillus spp. Reveal Their Potential to Alleviate Cold Stress and Modulate Phytohormones in Wheat. Microorganisms. 2019, 7, 337.
- Sanghera, G.S.; Wani, S.H.; Hussain, W.; Singh, N.B. Engineering Cold Stress Tolerance in Crop Plants. Curr. Genom. 2011, 12, 30.
- Igiri, B.E.; Okoduwa, S.I.; Idoko, G.O.; Akabuogu, E.P.; Adeyi, A.O.; Ejiogu, I. K. Toxicity and Bioremediation of Heavy Metals Contaminated Ecosystem from Tannery Wastewater: a Review. J. Toxicol. 2018. doi:10.1155/2018/2568038.
- Gautam, R.K.; Soni, S.; Chattopadhyaya, M. C. Functionalized Magnetic Nanoparticles for Environmental Remediation. In Handbook of Research on Diverse Applications of Nanotechnology in Biomedicine, Chemistry, and Engineering; Soni, S., Salhotra, A., Suar, M., Eds.; IGI Global: Hershey, PA, 2015; pp. 518–551.
- Syed, S.; Chinthala, P. Heavy Metal Detoxification by Different Bacillus Species Isolated from Solar Salterns. Scientifica. (Cairo). 2015. doi:10.1155/2015/319760
- Ma, Y.; Rajkumar, M.; Zhang, C.; Freitas, H. Beneficial Role of Bacterial Endophytes in Heavy Metal Phytoremediation. J. Environ. Manage 2016, 174, 14–25.
- Jadia, C.D.; Fulekar, M.H. Phytoremediation of Heavy Metals: Recent Techniques. Afr. J. Biotechnol 2009, 8, 921–928.
- Sytar, O, Brestic, M.; Taran, N.; Zivcak, M. Plants Used for Biomonitoring and Phytoremediation of Trace Elements in Soil and Water. Ahmad, P. Ed.: In Plant Metal Interaction: Emerging Remediation Techniques; Elsevier, 2016; pp. 361–384.
- Hao, Y.; Wu, H.; Liu, Y.; Hu, Q. Mitigative Effect of Bacillus Subtilis QM3 on Root Morphology and Resistance Enzyme Activity of Wheat Root Under Lead Stress. Adv. Microbiol 2015, 5, 469.
- Naseem, S.; Yasin, M.; Faisal, M.; Ahmed, A. Comparative Study of Plant Growth Promoting Bacteria in Minimizing Toxic Effects of Chromium on Growth and Metabolic Activities in Wheat (Triticum Aestivum). J. Chem. Soc. Pakistan 2016, 1, 38.
- Kuramshina, Z.M.; Smirnova, Y.V.; Khairullin, R.M. Cadmium and Nickel Toxicity for Sinapis Alba Plants Inoculated with Endophytic Strains of Bacillus Subtilis. Russ. J. Plant Physiol 2018, 65, 269–277.
- Treesubsuntorn, C.; Dhurakit, P.; Khaksar, G.; Thiravetyan, P. Effect of Microorganisms on Reducing Cadmium Uptake and Toxicity in Rice (Oryza Sativa L.). Environ. Sci. Pollut. Res 2018, 25, 25690–25701.
- Sarkar, D.; Ferguson, M.; Datta, R.; Birnbaum, S. Bioremediation of Petroleum Hydrocarbons in Contaminated Soils: Comparison of Biosolids Addition, Carbon Supplementation, and Monitor red Natural Attenuation. Environ. Pollut 2005, 136, 187–195.
- Varjani, S.J.; Upasani, V.N. Biodegradation of Petroleum Hydrocarbons by Oleophilic Strain of Pseudomonas Aeruginosa NCIM 5514. Bioresour.Technol 2016, 222, 195–201.
- Gojgic-Cvijovic, G.D.; Milic, J.S.; Solevic, T.M.; Beskoski, V.P.; Ilic, M.V.; Djokic, L.S.; Narancic, T.M.; Vrvic, M.M. Biodegradation of Petroleum Sludge and Petroleum Polluted Soil by a Bacterial Consortium: a Laboratory Study. Biodegradation 2012, 23, 1–4.
- Banat, I.M.; Satpute, S.K.; Cameotra, S.S.; Patil, R.; Nyayanit, N.V. Cost Effective Technologies and Renewable Substrates for Biosurfactants’ Production. Front. Microbiol 2014, 5, 697.
- Antoniou, E.; Fodelianakis, S.; Korkakaki, E.; Kalogerakis, N. Biosurfactant Production from Marine Hydrocarbon-Degrading Consortia and Pure Bacterial Strains Using Crude oil as Carbon Source. Front. Microbiol 2015, 7, 274.
- Ghazali, F.M.; Rahman, R.N.; Salleh, A.B.; Basri, M. Biodegradation of Hydrocarbons in Soil by Microbial Consortium. Int. Biodeterior. Biodegradation 2004, 1, 61–67.
- Gangola, S.; Sharma, A.; Bhatt, P.; Khati, P.; Chaudhary, P. Presence of Esterase and Laccase in Bacillus Subtilis Facilitates Biodegradation and Detoxification of Cypermethrin. Sci. Rep 2018, 8, 1–1.
- Bhatt, P.; Huang, Y.; Zhang, W.; Sharma, A.; Chen, S. Enhanced Cypermethrin Degradation Kinetics and Metabolic Pathway in Bacillus Thuringiensis Strain SG4. Microorganisms. 2020, 2, 223.
- Fang, D.; Yu, Y.; Wu, L.; Wang, Y.; Zhang, J.; Zhi, J. Bacillus Subtilis-Based Colorimetric Bioassay for Acute Biotoxicity Assessment of Heavy Metal Ions. RSC Adv. 2015, 5, 59472–59479.
- Okumura, S; Ohba, M.; Mizuki, E.; Crickmore, N.; Coté, J. C.; Nagamatsu, Y.; Kitada, S.; Sakai, H.; Harata, K.; Shin, T. Parasporin Nomenclature. 2010. http://parasporin.fitc.pref.fukuoka.jp/.
- Maeda, M.; Mizuki, E.; Nakamura, Y.; Hatano, T.; Ohba, M. Recovery of Bacillus Thuringiensis from Marine Sediments of Japan. Curr. Microbiol 2000, 40, 418–422.
- Wu, F. Mycotoxin Reduction in Bt Corn: Potential Economic, Health, and Regulatory Impacts. Transgenic Res. 2006, 15, 277–289.
- Qaim, M.; Zilberman, D. Yield Effects of Genetically Modified Crops in Developing Countries. Science 2003, 299, 900–902.
- Wolfenbarger, L.L.; Phifer, P.R. The Ecological Risks and Benefits of Genetically Engineeredplants. Science 2000, 290, 2088–2093.
- Torregrosa-Crespo, J.; Montero, Z.; Fuentes, J.L.; Reig García-Galbis, M.; Garbayo, I.; Vílchez, C.; Martínez-Espinosa, R.M. Exploring the Valuable Carotenoids for the Large-Scale Production by Marine Microorganisms. Mar. Drugs 2018, 16, 203.
- Perera, C.O.; Yen, G.M. Functional Properties of Carotenoids in Human Health. Int. J. Food Prop 2007, 10, 201–230.
- Barnett, Y.A.; King, C.M. An Investigation of Antioxidant Status, DNA Repair Capacity and Mutation as a Function of age in Humans. Mutation Research/DNAging 1995, 338, 115–128.
- Bendich, A. Recent Advances in Clinical Research Involving Carotenoids. Pure App. Chem 1994, 66, 1017–1024.
- Krinsky, N.I. Carotenoids as Antioxidants. Nutrition 2001, 17, 815–817.
- Sy, C.; Gleize, B.; Chamot, S.; Dangles, O.; Carlin, F.; Veyrat, C.C.; Borel, P. Glycosyl Carotenoids from Marine Spore-Forming Bacillus sp. Strains are Readily Bioaccessible and Bioavailable. Int. Food Res. J 2013, 51, 914–923.
- Hartz, P.; Milhim, M.; Trenkamp, S.; Bernhardt, R.; Hannemann, F. Characterization and Engineering of a Carotenoid Biosynthesis Operon from Bacillus Megaterium. Metab. Eng 2018, 49, 47–58.