ABSTRACT
The abundant and inexpensive transition metal iron was loaded onto the abundant nonmetal minerals of coal-bearing kaolin (CK) to synthesize cost-effective iron nanoparticle (FeNP)-loaded kaolin heterogeneous catalysts. Various characterization techniques were performed, such as X-ray diffraction (XRD), scanning electron microscopy (SEM), transmission electron microscopy (TEM) and X-ray photoelectron spectroscopy (XPS). The catalytic activity was screened for the direct esterification of benzoic acid with cyclic ether via activation of the C(sp3)-H bond in a cross-dehydrogenative-coupling reaction, and the corresponding α-acyloxy ether products were obtained in good yield. Notably, the FeNP-loaded CK solid catalysts were relatively stable and could be reused at least five times while retaining good catalytic activity.
GRAPHICAL ABSTRACT
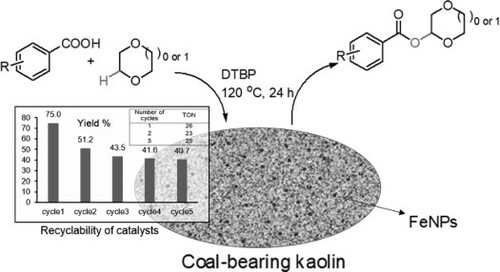
Highlights
Iron nanoparticle-loaded kaolin was successfully synthesized as a cost-effective heterogeneous catalyst.
The catalytic activity for the direct esterification of benzoic acid with cyclic ether via the C(sp3)-H bond activation CDC reaction was investigated.
The recycling test showed that good catalytic activity remained after 5 cycles.
1. Introduction
Transition metal-catalyzed direct functionalization of C–H bond formation in C-X (X = C, O, N, S, etc.) bonds has become an important tool in organic synthesis due to its excellent advantages, such as step economy, atom economy and environmental sustainability, which are also popular research topics in this area (Citation1). In past years, C–C bond formation via transition metal-catalyzed activation of C(sp)-H and C(sp2)-H bonds has attracted much attention from researchers, resulting in substantial development (Citation2). However, activation and functionalization of the C(sp3)-H bond catalyzed by transition metals remains a major challenge (Citation3).
For the formation of C-X bonds, cross-dehydrogenative-coupling (CDC) reactions play an important role. The advantage of the CDC reaction is that it does not require the prior introduction of a functional group onto the starting materials but directly removes one molecule of hydrogen from two raw material compounds and directly forms a C-X bond; therefore, it not only simplifies the reaction steps but also decreases the number of side products, thus providing excellent atom economy and step economy (Citation4). For catalysis of the CDC reaction, transition metals are more frequently reported that other catalysts and are regarded as having powerful catalytic activity; these transition metals include platinum, ruthenium, nickel, iridium, etc (Citation4, Citation5). However, these metals are expensive and have considerable toxicity, limiting their further development and use in wide applications. Thus, iron has attracted increasing attention as a transition metal and is regarded as having great potential for economic and environmental development due to its various features, including high abundance, widespread availability, low cost, and low toxicity (Citation6), 1d, f. Among the various iron-catalyzed reactions, the iron-catalyzed CDC reaction was first reported by the Li C. J research group in 2007, who used FeCl2 as a catalyst and di-tert-butyl peroxide (DTBP) as an oxidant, achieving direct dehydrogenative coupling formation of C(sp3)- C(sp3) bonds (Citation7). In the next year, the Li H. group reported an Fe2(CO)9-catalyzed CDC reaction between tetrahydrofuran and ethyl benzoylacetate to obtain a C(sp3)- C(sp3) coupling product (Citation8). Furthermore, there have been an increasing number of reports on iron-catalyzed C(sp3)-C(sp2) and C(sp3)-C(sp) bond formation reactions via C–H activation by the CDC reaction. For instance, the iron-catalyzed CDC reaction was used for benzyl C–H bonds with aromatic compounds (Citation9) and for a methyl group of tertiary aromatic amines with a 1-alkyne moiety (Citation10). However, since the first report of iron-catalyzed CDC reactions, many studies on ferric salts or organic iron-catalyzed cross dehydrogenative C–C coupling reactions have developed rapidly (1e,j,m,n, 6). Iron catalysis has attracted much attention in the area of CDC reaction research. However, the development of research on iron-catalyzed CDC formation of C–O bonds is relatively behind and still has many defects that need further evaluation.
In recent years, esters synthesized via the CDC reaction have received attention, and direct esterification functionalization could be realized via the selective activation of inactive C(sp3)-H bonds to form C–O bonds catalyzed by transition metals or nonmetal catalysts. This has important application value for medicinal chemistry and organic synthesis (Citation11). Yu et al. (Citation12) and Zhang et al. (Citation13) developed a direct synthesis method of benzyl esters via alkylbenzene cross coupling with carboxylic acids catalyzed by tert-butylammonium iodide (TBAI) and Pd(OAc)2, respectively. Wang et al. (Citation14) and Patel and coworkers (Citation15) synthesized benzyl esters via alkylbenzene cross coupling with aromatic aldehydes catalyzed by TBAI and Cu(OAc)2, respectively. Direct synthesis of allylic ester occurred via the activation of allylic C–H bonds by oxidative cross coupling with carboxylic acids using TBAI (Citation16), CuCl (Citation17), and CuO (Citation18) as catalysts. Regarding the CDC reaction synthesis of α-acyloxy ethers, the Wan group developed a TBAI-catalyzed oxidative coupling of ether and carboxylic acid using tert-butyl hydroperoxide (TBHP) as an oxidant (Citation19). The Cu(acac)2-catalyzed reaction was performed by Chaudhuri and coworkers (Citation20). [20] However, iron-catalyzed CDC for C(sp3)-O bond formation was reported by Han and coworkers (Citation21), who showed the oxidative reaction of α-C(sp3)-H of cyclic ethers with aromatic carboxylic acid using Fe(acac)3 as a catalyst and DTBP as an oxidant. Subsequently, Liu et al. used iron porphyrin as a catalyst and performed the above reaction; they used a catalyst amount of only 0.5 mol% and successfully decreased the reaction time from 24 h to 4 h (Citation22).
However, transition metal-catalyzed direct functionalization via C(sp3)-H bond selective activation to form the C–O bond by the CDC reaction has attracted increasing attention. Research on the iron catalyst in the above reaction remains in its infancy, and there are only a few reports on the α-C(sp3)-H cyclic ether activation CDC reaction. Therefore, in this area, this aspect remains a major challenge but has research prospects. To the best of our knowledge, previous reports have researched homogeneous iron catalysts for C(sp3)-H-activated CDC reactions, but until now, they have not reported solid supported iron heterogeneous catalysts. It is well known that organometallic or inorganic metallic catalysts have the deficiencies of being difficult to recycle and the inability to be discharged directly, and further treatment is required to avoid causing environmental pollution. Therefore, in recent years, precious metals such as palladium and gold nanoparticle-loaded catalysts for organoliquid reaction catalysis or photocatalysis have undergone rapid development due to their advantages, such as high catalytic efficiency, recyclability, and environmental and economic value (Citation23). Herein, iron nanoparticle (FeNP)-loaded catalysts are used, as they are inexpensive, easy to obtain, environmentally friendly and have low toxicity. These materials have important research significance in green catalysis.
Kaolin is an important nonmetallic clay mineral. In the Inner Mongolia area of China, the coal-bearing kaolin mineral is widely and abundantly found, and it is easy to mine and has high kaolinite quality and high economic value. Unfortunately, the coal-bearing kaolin of Inner Mongolia is used only in ceramics, coatings, etc., following a simple treatment such as high-temperature calcination. In recent years, some studies have been conducted on the application of kaolin for the removal of water pollutants via adsorption (Citation24). Herein, our research group successfully prepared palladium nanoparticle-loaded kaolin and found that it has excellent catalytic activity for the directed C–H functionalization of arylpyrazoles (Citation25). Additionally, in previous work, we successfully prepared polyhydroxy-iron-modified coal-bearing kaolin via an environmentally friendly method for the adsorption removal of anionic dyes from water (Citation26). In this work, we synthesized a series of iron nanoparticle-loaded coal-bearing kaolins and researched the heterogeneous catalytic activity for direct esterification to form C–O bonds via activation of inactive C(sp3)-H bonds of cyclic ether by CDC with aryl carboxylic acid.
2. Results and discussion
After preparing FeNP-loaded coal-bearing kaolin heterogeneous catalysts, a series of characterization methods were performed. The X-ray diffraction (XRD) patterns of raw coal-bearing kaolin (CK), organo-modified CK (NH2,Ph@CK), and fresh and used CK with 3%Fe/NH2,Ph (3%Fe/NH2,Ph@CK) are shown in . The XRD pattern of CK exhibited reflections at 12.2°, 20.6°, 24.8°, 25.6°, 36.5°, and 38.4°, which correspond to reflections of kaolinite (JCPDS file No. 79-1570). After treating with organic groups and iron, there were no indications of iron crystals or any other evident diffraction peaks other than CK. This means that functionalization by organic groups and iron had no influence on the CK crystal structure. The SBET result was 22 m2/g for 3%Fe/NH2,Ph@CK ( entry 3), suggesting that the addition of FeNP could improve the surface area of CK in small amounts (SBET of raw kaolin is 11 m2/g, entry 1). Elemental analysis of the iron is also shown in . There was a small quantity of iron in the raw kaolin (0.17 wt%, entry 1), and the content of iron in 3%Fe/NH2,Ph@CK was approximately 3%. After one cycle and five cycles, some of the iron content in the catalysts was lost (2.62% and 2.16%, entries 4 and 5), but fortunately, up to 2% of the iron contents remained. The scanning electron microscopy-energy dispersive X-ray spectroscopy (SEM-EDS) elemental content results also showed a similar Fe content of 2.9 wt% (b), which suggests that iron is distributed in the surface shallow layer of kaolin. On the other hand, in the XRD pattern, the 2θ reflection peak of the 001 crystal plane did not undergo any shift for either CK or 3%Fe/NH2,Ph@CK at 12.2° (). According to the literature, if the crystal can be intercalated, the 001 crystal plane reflection will shift down, which means that iron did not intercalate into the kaolin interlayer (Citation27). However, both the SEM-EDS elemental mapping (c) and the transmission electron microscopy-EDS (TEM-EDS) elemental mapping (d) showed that iron was distributed uniformly on kaolin. From the TEM image, it was observed elliptical Fe nanoparticles in both of fresh and used catalysts (b and c), sized as widths around 30-50 nm, length around120-150 nm. To observe the metallic state of iron on kaolin, X-ray photoelectron spectroscopy (XPS) analysis was performed for fresh and 5th-cycle 3%Fe/NH2,Ph@CK catalysts. As shown in , both the fresh and used catalysts exhibited a binding energy at 710.8 eV, which is attributed to the 2p3/2 peak of FeIII (Citation28). Over the cycles of use, the valence of Fe did not change, suggesting that FeIII is the catalytic cycle active center.
Figure 2. (a) SEM image, (b) SEM/EDS and (c) SEM-EDS elemental mapping of Al, Si, O, and Fe respectively.
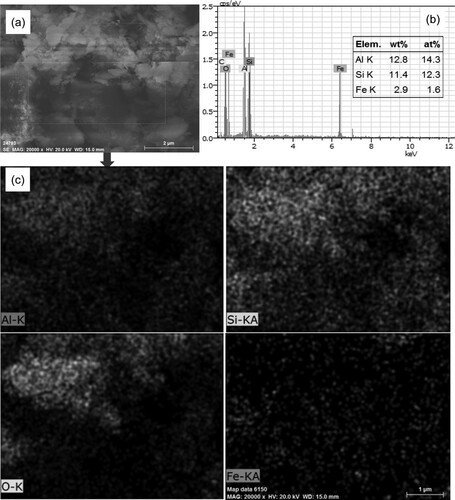
Figure 3. TEM images of (a) (b) fresh 3wt%Fe/NH2,Ph@CK and (c) used 3wt%Fe/NH2,Ph@CK, (d) TEM/EDS elemental mapping of 3wt%Fe/NH2,Ph@CK.
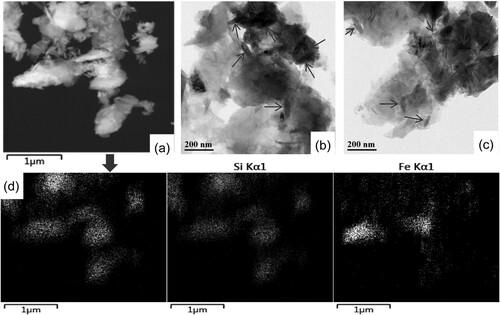
Table 1. Iron elemental analysis and SBET results.
To investigate the catalytic efficiency of kaolin-supported iron nanoparticles for the activation of C–H via CDC to form C–O bonds, the model reaction chosen was with 4-methoxybenzoic acid (1a) and 1,4-dioxane (2a) as substrates. Initially, we discussed the effect of the support material on the catalytic activity using raw kaolin and various organo-modified and unmodified kaolin-supported FeNPs, as shown in . All reactions were performed under the same conditions, with DTBP as the oxidant, at 120 °C, and under stirring for 24 h. According to the images, the product was not detected if the reaction was catalyst-free or if raw kaolin was used as the catalyst. However, the FeNP-loaded unmodified kaolin catalyst of 3%Fe@CK, the FeNP-loaded organo-modified kaolin catalyst of 3%Fe/NH2@CK and 3%Fe/Ph@CK showed efficacious catalytic activities, giving chemical yields of 56.0% (entry 3) 59.6% (entry 4) and 55.8% (entry 5), respectively; notably, Fe/NH2,Ph@CK gave an excellent yield of 75% (entry 6). This suggests that using NH2 and Ph groups to modify kaolin with organic groups is beneficial for improving the chemical yields of FePN-loaded kaolin-catalyzed reactions. According to previous work[25] and this work, it is possible that using an –NH2 group to functionalize the kaolin surface is beneficial for improving the catalytic efficiency because FeNPs are uniformly distributed on the kaolin surface, and functionalizing with the -Ph group is beneficial for increasing the organophilic property of the kaolin surface. Further, to detect the influence of the loading amount of iron on the catalytic efficiency of kaolin, a series of different wt% Fe//NH2,Ph@CK ratios of 1 wt% to 5 wt% were tested in the above reaction (, entries 6-10). It is clear that the iron loading amount has an influence on the catalytic efficiency, and the best performance was achieved with the 3 wt% Fe-loaded catalyst, with an efficiency that was significantly higher than that of the catalysts loaded with higher amounts of 4 and 5 wt% Fe (61.8% and 62.9%, entries 9 and 10) and lower amounts of 1 and 2 wt% Fe (58.9% and 63.8%, entries 7 and 8). Further, we discussed the other iron compounds as catalysts, including iron oxides and iron salts of Fe2+ and Fe3+ (, entries 7-10), but they did not provide any improvement. The amount (mol%, comparison with 4-methoxybenzoic acid) of 3wt%Fe/NH2,Ph@CK added was also discussed. The results showed that a wide range of catalyst loading gave good yields of up to 60%; note that when 3 and 10 mol% of 3wt%Fe/NH2,Ph@CK were added, the best yields of 75% (, entry 2) and 74% (entry 5) were achieved. However, from an economic viewpoint, 3 mol% was selected as the optimized amount. For comparison, we also loaded FeNPs on commercial γ-Al2O3 to prepare a catalyst; unfortunately, it provided a product yield of only 47.0%. As shown in , a series of oxidants were tested, such as DTBP, TBHP, H2O2, CH3CO3H, cumene hydroperoxide, benzoyl peroxide, and tert-butyl peroxybenzoate. Among them, DTBP (entry 1) was demonstrated to be superior to the other oxidants. Additionally, the reaction did not proceed without the oxidant (entry 8). In the optimization studies, the reaction time (8, 12, 24, 36, and 48 h) and reaction temperature were also investigated. A reaction time of 24 h at a reaction temperature of 120 °C (b) showed the highest product yield (a).
Table 2. Effect of different functionalized support material of kaolin and different loaded content of iron for catalytic activity.Table Footnotea
Table 3. Different amount of 3wt%Fe/NH2,Ph@CK was used in the reaction or other various different catalysts were used in the reaction.Table Footnotea
Table 4. The effect of oxidants on the reaction.
The scope of the FeNP-loaded CK heterogeneous-catalyzed CDC reaction of substituted benzoic acids with 1,4-dioxane is presented in . Benzoic acid with electron donating groups was smoothly converted to a-acyloxy ethers (3a-m) as yields up to 40%, especially para-OMe, para-tert-butyl and non-substituted benzoic acid, giving the desired products in good to excellent yields of 75% (3a), 62% (3 l) and 64% (3b), respectively. However, electron-withdrawing groups of halogen and nitro groups (3r-x) were not detected, and substituted para-formyl (3n) and para-cyano (3q) groups exhibited low desired product yields. This result suggests that electron-withdrawing groups are not beneficial for the reaction.
Table 5. Substitute scope of various benzoic acids catalyzed reaction with 1,4-dioxane.Table Footnotea
To extend the scope of cyclic ethers, 1,3-dioxolane and tetrahydrofuran (THF) were tested in the coupling reaction with benzoic acids (the results are shown in ). Herein, 1,3-dioxolane resulted in the corresponding product, but its yield was as low as 20-25% (4a-e). However, THF does not undergo oxidative coupling with benzoic acid to give the corresponding product, so we tried using it in a standard reaction herein (4f).
Table 6. Coupling of 1,3-Dioxolane and Tetrahydrofuran (THF) with Substituted Benzoic Acids.Table Footnotea
To investigate the possibility of recycling the FeNP-loaded coal-bearing kaolin catalyst, the reactions of 4-methoxybenzoic acid (1a) and 1,4-dioxane (2a) were examined. Although after reusing for two to five cycles, the product yield of 3a () decreased, but TON still remained (). It suggest the activity catalyst would be remained after reusing several cycles, the product yield decreased probably due to loss of iron loaded amount. Therefore, following after improve immobilization of iron on kaolin, the FeNP-loaded coal-bearing kaolin catalyst is preferred for green catalysis in the C(sp3)-H activation via the CDC reaction.
To determine the mechanistic details of the heterogeneous iron-catalyzed oxidative dehydrogenative esterification of cyclic ether with carboxylic acid, first, a radical capture experiment was performed. When 1 eq of the radical inhibitor TEMPO (2,2,6,6-tetramethylpiperidine-N-oxyl) was present in the reaction of 1 mmol of 4-methoxybenzoic acid (1a) and 2 ml of 1,4-dioxane (2a), a 52% yield of the corresponding product was obtained, but when 2 or 3 eq of TEMPO was added, no desired product was formed. Next, an intermolecular competing kinetic isotope effect (KIE) experiment was performed in a model reaction in the presence of 1:1 1,4-dioxane and 1,4-dioxane-d8. According to the 1H NMR spectroscopic analysis results, a KIE value of kH/kD = 2.5 was found (see SI). This result suggests that the C(sp3)-H bond of ether cleavage was the possible rate-determining step. On the basis of our above results and the literature reports,[11, 21, 22] the reaction mechanism was presumed and is illustrated in . First, FeNPs were oxidized by DTBP and combined with benzoic acid to form FeIV complex A. Next, complex A captured the hydrogen of 1,4-dioxane to form complex B and provided the corresponding radical intermediate. Then, the radical intermediate reacted with complex B to release the desired product.
3. Conclusion
In this work, to realize an atom economic, step economic, and environmentally sustainable green catalytic system, iron salts and abundant nonmetal minerals of coal-bearing kaolin were used to successfully synthesize FeNP-loaded kaolin heterogeneous catalysts. From the TEM images, the iron nanoparticle size was 3.1 nm, and both TEM and SEM elemental mappings showed that iron was distributed uniformly on kaolin. Both the fresh and used catalysts showed binding energies in the Fe 2P XPS spectra at 710.8 eV, which is attributed to the FeIII 2p3/2 peak. The catalytic activity for the C(sp3)-H bond activation in a CDC reaction was determined using a model reaction of the direct esterification of benzoic acid with cyclic ether. The model reaction showed efficient catalytic activity for various substituted benzoic acids with 1,4-dioxane and 1,3-dioxolane. The catalyst can be reused and remains active after 5 cycles. The prepared catalyst is easy to separate and recycle and is preferred for green catalysis in the C(sp3)-H activation CDC reaction, thus having the potential to be an alternative to homogeneous iron catalysts.
Supporting information
Details of experimental section, spectra of the KIE experiment and 1H and 13C NMR spectra are given in supporting information.
Acknowledgement
This research was financially supported by the National Science Foundation of China (22061034), the Research Program of Science and Technology at University of Inner Mongolia Autonomous Region (NJZZ21010), and the Collaborative Innovation Center for Water Environmental Security of Inner Mongolia Autonomous Region (XTCX003).
Disclosure statement
No potential conflict of interest was reported by the author(s).
References
- Huang, Z.; Lumb, J.P. Phenol-Directed C–H Functionalization. ACS. Catal 2019, 9, 521–555. (b) Shalit, H.; Dyadyuk, A.; Pappo, D. Selective Oxidative Phenol Coupling by Iron Catalysis. J. Org. Chem. 2019, 84, 1677−1686. (c) Chu, XQ.; Ge, D.; Shen, ZL.; Loh, TP. Recent Advances in Radical-Initiated C(sp3)–H Bond Oxidative Functionalization of Alkyl Nitriles. ACS. Catal. 2018, 8, 258−271. (d) Shang, R.; Ilies, L.; Nakamura, E. Iron-Catalyzed C–H Bond Activation. Chem. Rev. 2017, 117, 9086−9139. (e) Qin, Y.; Zhu, L.; Luo, S. Organocatalysis in Inert C–H Bond Functionalization. Chem. Rev. 2017, 117, 9433−9520. (f) Cera, G.; Ackermann, L. Iron-Catalyzed C–H Functionalization Processes. Top. Curr. Chem. 2016, 374, 57. (g) Girard, SA.; Knauber, T.; Li, C. The Cross-Dehydrogenative Coupling of C -H Bonds: A Versatile Strategy for C-C Bond Formations. Angew. Chem. Int. Ed. 2014, 53, 74- 100. (h) Scheuermann, C. Beyond Traditional Cross Couplings: The Scope of the Cross Dehydrogenative Coupling Reaction. J. Chem-Asian. 2010, 5, 436- 451. (i) Yeung, CS.; Dong, VM. Catalytic Dehydrogenative Cross-Coupling: Forming Carbon−Carbon Bonds by Oxidizing Two Carbon−Hydrogen Bonds. Chem. Rev. 2011, 111, 1215-1292. (j) Krylov, IB.; Vil, VA.; Terent'ev, AO. Cross-dehydrogenative coupling for the intermolecular C–O bond formation. Beilstein. J. Org. Chem. 2015, 11, 92- 146. (k) Li, C-J. Cross-Dehydrogenative Coupling (CDC): Exploring C−C Bond Formations beyond Functional Group Transformations. A Acc. Chem. Res. 2009, 42, 335- 344. (l) Sun, CL.; Li, BJ.; Shi, Z. Direct C−H Transformation via Iron Catalysis. Chem. Rev. 2011, 111, 1293-1314. (m) Sun, C-L.; Li, B-J.; Shi, Z-J. Direct C−H Transformation via Iron Catalysis. Chem. Rev. 2011, 111, 1293–1314. (n) Jia, F.; Li, Z-P. Iron-catalyzed/mediated oxidative transformation of C–H bonds. Org. Chem. Front. 2014, 1, 194–214.
- Wu, Y.N.; Wang, J.; Mao, F.; Kwong, F.Y. Palladium-Catalyzed Cross-Dehydrogenative Functionalization of C(sp2)-H Bonds. Chem. Asian J 2014, 9, 26–47. (b) Shi, W.; Liu, C.; Lei, A. Transition-metal catalyzed oxidative cross-coupling reactions to form C–C bonds involving organometallic reagents as nucleophiles. Chem. Soc. Rev. 2011, 40, 2761−2776. (c) Liu, C.; Zhang, H.; Shi, W.; Lei, A. Bond Formations between Two Nucleophiles: Transition Metal Catalyzed Oxidative Cross-Coupling Reactions. Chem. Rev. 2011, 111, 1780−1824.
- Pla, D.; Gomez, M. Metal and Metal Oxide Nanoparticles: A Lever for C−H Functionalization. ACS Catal. 2016, 6, 3537–3552.
- Krylov, I.B.; Vil, V.A.; Terent’ev, A.O. Cross-dehydrogenative Coupling for the Intermolecular C–O Bond Formation. Beilstein. J. Org. Chem 2015, 11, 92–146.
- Parsharamulu, T.; Vishnuvardhan, R.P.; Likhar, P.R.; Lakshmi, K.M. Dehydrogenative and Decarboxylative C–H Alkynylation of Heteroarenes Catalyzed by Pd(II)–Carbene Complex. Tetrahedron 2015, 71, 1975–1981. (b) Kozhushkov, SI.; Ackermann, L. Ruthenium-catalyzed direct oxidative alkenylation of arenes through twofold C–H bond functionalization. Chem. Sci. 2013, 4, 886-896. (c) Jin, LK.; Wan, L.; Feng, J.; Cai, C. Nickel-Catalyzed Regioselective Cross-Dehydrogenative Coupling of Inactive C(sp3)–H Bonds with Indole Derivatives. Org. Lett. 2015, 17, 4726- 4729. (d) Li, F.; Meng, Z.; Hua, J.; Li, W.; Lou, H.; Liu, L. Indium-catalyzed oxidative cross-dehydrogenative coupling of chromenes with 1,3-dicarbonyls and aryl rings. Org. Biomol. Chem. 2015, 13, 5710-5715. (e) Rosen, BM.; Quasdorf, KW.; Wilson, DA.; Zhang, N.; Resmerita, AM.; Garg, NK.; Percec, V. Nickel-Catalyzed Cross-Couplings Involving Carbon−Oxygen Bonds. Chem. Rev. 2011, 111, 1346–1416.
- Itazaki, M.; Nakazawa, H. Iron-Catalyzed Cross-Dehydrogenative-Coupling Reactions. Top. Organomet. Chem. 2015, 50, 47–81.
- Li, Z.; Cao, L.; Li, C.-J. FeCl2-Catalyzed Selective C-C Bond Formation by Oxidative Activation of a Benzylic C-H Bond. Angew. Chem. Int. Ed 2007, 46, 6505–6517.
- Li, Z.; Yu, R.; Li, H. Iron-Catalyzed C-C Bond Formation by Direct Functionalization of C-H Bonds Adjacent to Heteroatoms. Angew. Chem. Int. Ed 2008, 47, 7497–7500.
- Li, Y.-Z.; Li, B.-J.; Lu, X.-Y.; Lin, S.; Shi, Z.-J. Cross Dehydrogenative Arylation (CDA) of a Benzylic C-H Bond with Arenes by Iron Catalysis. Angew. Chem. Int. Ed 2009, 48, 3817–3820.
- Volla, C.M.R.; Vogel, P. Chemoselective C−H Bond Activation: Ligand and Solvent Free Iron-Catalyzed Oxidative C−C Cross-Coupling of Tertiary Amines with Terminal Alkynes. Reaction Scope and Mechanism. Org. Lett 2009, 11, 1701–1704.
- Majji, G.; Rout, S.K.; Rajamanickam, S.; Guin, S.; Patel, B.K. Synthesis of Esters via sp3 C–H Functionalization. Org. Biomol. Chem 2016, 14, 8178–8211.
- Feng, J.; Liang, S.; Chen, S.Y.; Zhang, J.; Fu, S.S.; Yu, X.Q. A Metal-Free Oxidative Esterification of the Benzyl C-H Bond. Adv. Synth. Catal 2012, 354, 1287–1292.
- Liu, H.; Shi, G.; Pan, S.; Jiang, Y.; Zhang, Y. Palladium-Catalyzed Benzylation of Carboxylic Acids with Toluene via Benzylic C–H Activation. Org. Lett 2013, 15, 4098–4101.
- Huang, J.; Li, L.T.; Li, H.Y.; Husan, E.; Wang, P.; Wang, B. Bu4NI-catalyzed Benzylic Acyloxylation of Alkylarenes with Aromatic Aldehydes. Chem. Commun 2012, 48, 10204–10206.
- Rout, S.K.; Guin, S.; Ghara, K.K.; Banerjee, A.; Patel, B.K. Copper Catalyzed Oxidative Esterification of Aldehydes with Alkylbenzenes via Cross Dehydrogenative Coupling. Org. Lett 2012, 14, 3982–3985.
- Shi, E.; Shao, Y.; Chen, S.; Hu, H.; Liu, Z.; Zhang, J.; Wan, X. Tetrabutylammonium Iodide Catalyzed Synthesis of Allylic Ester with Tert-Butyl Hydroperoxide as an Oxidant. Org. Lett 2012, 14, 3384–3387.
- Tran, B.L.; Driess, M.; Hartwig, J.F. Copper-Catalyzed Oxidative Dehydrogenative Carboxylation of Unactivated Alkanes to Allylic Esters via Alkenes. J. Am. Chem. Soc 2014, 136, 17292–17301.
- Wang, C.Y.; Song, R.J.; Wei, W.T.; Fan, J.H.; Li, J.H. Copper-catalyzed Oxidative Coupling of Acids with Alkanes Involving Dehydrogenation: Facile Access to Allylic Esters and Alkylalkenes. Chem. Commun 2015, 51, 2361–2363.
- Chen, L.; Shi, E.; Liu, Z.; Chen, S.; Wei, W.; Li, H.; Xu, K.; Wan, X. Bu4NI-Catalyzed C-O Bond Formation by Using a Cross-Dehydrogenative Coupling (CDC) Reaction. Chem. – Eur. J 2011, 17, 4085–4089.
- Talukdar, D.; Borah, S.; Chaudhuri, M.K. Bis(Acetylacetonato)Copper(II) Catalyzed Oxidative Cross-Dehydrogenative Coupling (CDC) for the Synthesis of α-Acyloxy Ethers Through Direct Activation of α-C(sp3)–H Bond of Cyclic Ether. Tetrahedron Lett. 2015, 56, 2555–2558.
- Zhao, J.; Fang, H.; Zhou, W.; Han, J.; Pan, Y. Iron-Catalyzed Cross-Dehydrogenative Coupling Esterification of Unactive C(sp3)–H Bonds with Carboxylic Acids for the Synthesis of α-Acyloxy Ethers. J. Org. Chem 2014, 79, 3847–3855.
- Wen, W.H.; Xie, A.N.; Wang, H.H.; Zhang, D.X.; Ali, A.; Ying, X.; Liu, H.Y. Iron Porphyrin-Catalyzed C(SP3) -H Activation for the Formation of C-O Bond via Cross-Dehydrogenative Coupling of Cycloether and Aromatic Acid. Tetrahedron 2017, 73, 7169–7176.
- Zhang, Y.; Cui, X.J.; Shi, F.; Deng, Y.Q. Nano-Gold Catalysis in Fine Chemical Synthesis. Chem. Rev 2012, 112, 2467–2505. (b) Turner, M.; Golovko, VB.; Vaughan, OPH.; Abdulkin, P.; Berenguer-Murcia, A.; Tikhov, MS.; Johnson, BFG.; Lambert, RM. Selective oxidation with dioxygen by gold nanoparticle catalysts derived from 55-atom clusters. Nature 2008, 454, 981-983. (c) Hashmi, ASK.; Hutchings, GJ. Gold Catalysis. Angew. Chem. Int. Ed. 2006, 45, 7896-7936. (d) Grirrane, A.; Corma, A.; Carcia, H. Gold-Catalyzed Synthesis of Aromatic Azo Compounds from Anilines and Nitroaromatics. Science 2008, 322, 1661-1664.
- Hai, Y.; Li, X.; Wu, H.; Zhao, S.; Deligeer, W.; Asuha, S. Modification of Acid-Activated Kaolinite with TiO2 and its use for the Removal of azo Dyes. Appl. Clay Sci 2015, 114, 558–567. (b) Gao, Z.; Li, X.; Wu, H.; Zhao, S.; Deligeer, W.; Asuha S. Magnetic modification of acid-activated kaolin: Synthesis, characterization, and adsorptive properties. Microporous Mesoporous Mater. 2015, 202, 1-7.
- Yang, P.; Bao, Y.S. Palladium Nanoparticles Supported on Organofunctionalized Kaolin as an Efficient Heterogeneous Catalyst for Directed C–H Functionalization of Arylpyrazoles. RSC. Adv 2017, 7, 53878–53886.
- Muschin, T.; Duo, X.; Bao, U.; Zulchin, H.; Agula, B. Environmentally Friendly Treatment of Coal-Bearing Kaolin by Polyhydroxy-Iron for Anionic Dye Removal. Chem. Select 2019, 4, 13810–13816.
- Éva, M.; András, K.; Tamás, K. Influencing Parameters of Direct Homogenization Intercalation of Kaolinite with Urea, Dimethyl Sulfoxide, Formamide, and N-Methylformamide. Appl. Clay Sci 2019, 182, 105287. (b) Éva, M.; János, K.; Erzsébet, H.; Veronika, V. Mechanochemical intercalation of low reactivity kaolinite. Appl. Clay Sci. 2013, 83-84, 24-31. (c) Li, X.; Liu, Q.; Cheng, H.; Zhang, S.; Frost, L. R. Mechanism of kaolinite sheets curling via the intercalation and delamination process. J.Colloid Interface Sci. 2015, 444, 74-80.
- Hawn, D.D.; DeKoven, B.M. Deconvalution as a Correction for Photoelectron Inelastic Energy Losses in the Core Level XPS Spectra of Iron Oxides. Surf. Interface Anal 1987, 10, 63. (b) Carvert, J. C.; Schweitzer, G. K. Use of X-Ray Photoelectron Spectroscopy to Study Bonding in Cr, Mn, Fe, and Co Compounds. J. Chem. Phys. 1972, 57, 973-982.