ABSTRACT
Gold nanoparticles (AuNPs) are essential metal nanoparticles, and they have much application in different areas such as bio-imaging, medical therapy, enzyme assays and the environment. Various physical and chemical methods have been used for the synthesis of AuNPs. However the disadvantage of most of them is to generate a large number of by-products that are dangerous, in this sense, green synthesis with plant extracts has become one of the preferred methods for the development of these materials because it produces particles with high bioavailability and reduces the use of toxic chemicals. In addition to presenting unique characteristics of biocompatibility, large surface area, and high dispersion, they have been used to treat different types of cancer, such as colon cancer. This review presents the various methods for synthesizing gold nanoparticles and their application in cancer therapy, focusing on the green synthesis of gold nanoparticles and their specific colon cancer application.
GRAPHICAL ABSTRACT
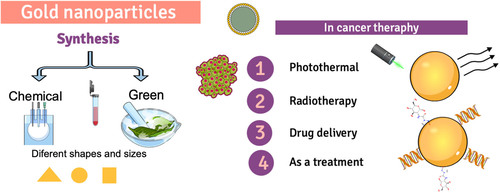
1. Introduction
Nanotechnology is a recently emerging discipline involving biology, physical and chemical methods to crate nano-sized particles (1–100 nm), holding particular functions and applications in science such as physics, medicine, pharmacy, food technology, and material science (Citation1). One of the most studied nanomaterials is the metal nanoparticles obtained by different metals like gold (Au) (Citation2), nickel (Ni) (Citation3), silver (Ag) (Citation4), platinum (Pt) (Citation5), iron (II, III) oxide (Fe2O3, Fe3O4, FeO) (Citation6) and zinc oxide (ZnO) (Citation7). One of the most studied metal nanoparticles is the gold nanoparticles (AuNPs), principally because they have unique properties. They include chemical stability, biocompatibility, simple preparation, easy modification, plasmonic and optical properties, that make them viable for use in diverse therapeutic applications, principally for drug delivery systems in many diseases like cancer, cardiovascular diseases, diabetes mellitus; developed of biosensors, and environmental applications (Citation8–10). AuNPs can be synthesized using chemical and physical methods. However often require expensive equipment and leads to the generation of toxic by-products, as an alternative to these methods was the green synthesis, which offers advantages like the use of environmentally friendly solvents and non-toxic reducing agents, which are extracted from plants, peel of fruits, bacteria, and fungi (Citation11,Citation12). AuNPs have recently been studied as an alternative to conventional cancer treatments, one of the most common types of cancer is colon cancer.
Colon cancer is one of the leading health problems, causing death worldwide. According to World Cancer Research Fund, it is the third most commonly occurring cancer in men and the second most commonly occurring cancer in women. There were over 1.8 million new cases in 2018, developed countries present. The countries with the highest incidence are Hungary, South Korea, Slovakia, and Norway. Colon cancer is more incident among men than women and is 3.5 times more common in developed countries. In the United States, 148,000 new cases and 53, 200 deaths per year were estimated (Citation13,Citation14), in Mexico, according to the Mexican Institute of Social Security (IMMS), it is estimated that 15,000 new cases are diagnosed each year in the country. It is ranked as the second cause of death due to malignant tumors in men after prostate cancer, and although the highest prevalence is located among the population aged 60–65 years, more cases are reported in people aged 40–30 years recently (Citation15). Generally, the treatment of patients with colon cancer includes surgery, chemotherapy, and radiotherapy. The latter two are associated with severe side effects: hematological toxicity, nausea, vomiting, pain, diarrhea, nervous system depression, allergic reactions, and alopecia (Citation16,Citation17). For these reasons, gold nanoparticles emerge as a viable alternative for use as a treatment against this disease, eradicating these side effects.
There is a great diversity of reports of the use of metallic nanoparticles to eliminate cancer cells. However, the mechanism of action is not yet clear; however, it is considered that initially, the AuNPs are endocytosed. Once inside the cell cytoplasm, they increase reactive oxygen species, move selectively to the periphery of the cell nucleus, and cause damage to the genetic material; the nucleus loses structure. Finally, the cells die by apoptosis; besides this mechanism, nanoparticles can also be functionalized on their surface by adding compounds such as peptides, antibodies, and DNA, making them more efficient and selective the reduce cancer cells (Citation18,Citation19). Despite the different reports, it is still necessary to carry out studies on how the elimination of cells is carried out and the possible effects of toxicity and accumulation in normal cells.
The current review article gives an insight into the synthesis of gold nanoparticles and their application as therapeutics in cancer. These are mainly focused on green synthesis with plant extracts, parameters of synthesis, characteristics of nanoparticles obtained, and the possible mechanism to induce cytotoxicity in colon cancer to differentiate which sizes and shapes have the best characteristics and how the various plant compounds used for synthesis influence the elimination of cancer cells.
1. Gold nanoparticles
In the past years, gold was used only as a metal for the fabrication of jewelry and utensils; however, with advances in nanotechnology, its physicochemical properties have been studied, making it an ideal material for nanoparticle manufacture. Gold nanoparticles, also called gold colloids, usually have a size between 1 and 100 nm, exist in different morphology types, including nanospheres, nanorods, nanoshells, and nanocages (Citation20). They are the most stable metal nanoparticles and display unique properties (depends on their size, shape, hollowness/porosity, and other properties) not found in bulk-size materials. They are preferred over other metal nanoparticles because they have different advantages, such as easy synthesis. The shape and size may be controlled by experimental parameters, present a large surface-to-volume ratio, possess unique optical properties, and exhibit excellent compatibility with almost chemically and biological molecules (Citation21,Citation22). Some examples of the applications of gold nanoparticles are sensors (Citation23), diagnostics (Citation24), therapeutic agents (Citation25), catalysis (Citation26), photodynamic therapy (Citation27), and enzymatic assays (Citation28).
2. Synthesis of gold nanoparticles
Gold nanoparticles are typically synthesized using two strategies, ‘top-down’ and ‘bottom-up’ (); for the first, the bulk material (gold) is systematically broken down to generate nanosized materials with different dimensions. A pattern matrix controls the particles arrays and shape; this approach includes methods like bulk metal grinding, sputtering, laser ablation, thermal decomposition, and lithography, whereas in the bottom up, the atoms are arranged to form nanosized molecular structures; this strategy uses colloidal techniques including chemical and green methods (Citation29,Citation30).
2.1. Chemical synthesis
Generally, chemical methods include two main steps: reduction and stabilization, in the first step reducing Au+1 or Au+3 to Au0 by adding an electron donor (reducing agent) in the reaction, exist different procedures for chemical synthesis (Citation22,Citation31), currently the most representative and popular methods are the following: Turkevich method introduced in 1951 by John Turkevich, uses trisodium citrate as a reducing and stabilizing agent and a solution of HAuCl4 as a precursor (Au+3), in 1973 Frens published an improvement to the method by varying the concentration of sodium citrate and gold, obtaining particles with a broad size range from 15 to 150 nm. This method is frequently applied because the rather loose shell of citrates on the particle surface is easily replaced by other desired ligands (antibodies, DNA, oligonucleotide) with valuable functions (Citation32). Another important method was Brust-Schiffrin discovered in 1994; in this method, HAuCl4 aqueous solution was transferred to organic phase (toluene) using phase transfer catalyst tetra-octyl-ammonium bromide (TOAB). Further reduction was obtained using sodium borohydride (NaBH4) in the presence of a thiol group derivative such as alkanethiol or dodecanethiol, which resulted in a change in color of the solution from orange to a deep brown. The seeded-growth method used a reducing agent to form Au0 nanoseeds from a gold precursor in the first stage. These seeds are induced to grow into gold nanorods in a second stage, using molecules (usually cationic surfactants) that are preferably adsorbed on specific crystalline facets showing high surface energies (Citation21,Citation30,Citation33).
2.2. Green synthesis
Traditional chemical methods have the principal disadvantage of using toxic chemicals and solvents for the synthesis added to the generation of toxic by-products to human health and the environment. Therefore to overcome these limitations, researchers have developed new routes to fabricated safe nanoparticles based on the reduction and capping potential of different metabolites from biological systems like plants, bacteria, yeast, and fungi (Citation29,Citation34,Citation35). Some reports of green synthesis of AuNPs are reported in .
Table 1. Green synthesis of gold nanoparticles with microorganisms and plants.
2.2.1. Microorganism-based AuNPs synthesis
The use of microorganisms to synthesize AuNPs have recently emerged because of the different advantages: low cost, production on a large scale, and non-toxic products. The most explored microorganisms in the synthesis of nanoparticles include bacteria, actinomycetes, viruses, and yeast (); they can produce AuNPs in two forms: ‘intracellular synthesis,’ consisting in the transportation of specific ions into the cell wall, then the enzymes present to convert the toxic metals into non-toxic nanoparticles with exact dimension depending on the localization of the reductive components; the other form ‘extracellular synthesis’ involves the secreted reductive enzymes like nitrate reductase, hydroquinone, hydrogenase (Citation10,Citation34). The extracellular production has wider application (optoelectronics, bioimaging, and sensor technology) than intracellular synthesis because they require additional processing steps for nanoparticle release this process includes ultrasound treatment or reaction with suitable detergents (Citation1,Citation36).
2.2.2. Plant, fruit, and waste extracts-based AuNPs synthesis
The synthesis of AuNPs with plants can be carried out by two means. The first of them is intracellular synthesis, in which the chosen plant grows in a medium rich in metal ions; The metal ions are transferred from the medium to the inside of the plant, and their metabolites reduce them to form metal nanoparticles (Citation37). Raju et al., Citation2012 report that the intracellular synthesis of gold nanoparticles ranged from 4 to 6 nm in size using living peanut seedlings. The nanoparticles formed were entrapped in the plant biomass, creating a film. To separate them requires additional processes which limit its applications, so it is not a widely used method, and synthesis by the second way is preferred (Citation38).
The second way to synthesize AuNPs is using plant extracts (of different parts: leaves, flowers, stems, and roots), which is a relatively new emerging research area. This synthesis has gained significant interest in recent years due to its potential to design and develop alternative, safer, cheaper, reliable, and less toxic nanoparticles set up towards the classic synthesis route. IT is more promising than the microorganisms because the plant extracts are easily obtainable and do not need to undergo the complex process of maintaining the cell cultures ().
Different plant extracts have been used to successfully synthesize AuNPs, instead of a great variety of shapes and sizes depending on the extract concentration, temperature, and pH at which the reaction is carried out (Citation39). An example of this type of synthesis are the AuNPs reported by Botteon et al., 2021 who used extracts of Salix alba leaves, obtaining particles with spherical morphology, diameters between 50 and 63 nm, and antifungal and muscle relaxant properties (Citation40); the aqueous extract of Simarouba glauca or Aceituno was used for the spherical and triangular nanoparticles synthesis, whose diameters decrease as the concentration of extract increases. These particles showed antimicrobial activity against different pathogens such as S. aureus (Citation41). AuNPs were synthesized with the extract of Hygrophila spinosa T. Anders, obtaining spherical particles with excellent stability and diameters of 68 nm approximately; these particles presented antioxidant capacity due to the extract molecules adhered to the surface and in vitro cytotoxic activity against different cell lines of breast and ovarian cancer (Citation42). Other examples are presented in .
Just as plant extracts, fruits possess large quantities of antioxidant compounds capable of reducing metal salts. For example, fruits such as blueberries, blackberries, grapes, pomegranate, and strawberries contain many anthocyanins, ascorbic acid, phenolic compounds, flavonoids polysaccharides, and other vitamins that can donate electrons that allow the synthesis of particles (Citation43). The synthesis with fruit involves the extraction of juice or pulp to be mixed with the metal salts. However, the fruit can be used to obtain antioxidant compounds from the shells; Hence, various metal nanoparticles have been synthesized from these types of extracts, some of which are mentioned below. Fruit extract of Chaenomeles sinensis was used to synthesize spherical particles with 20–40 nm of diameter. They present antimicrobial and cytotoxic activity against S. aureus, E. coli and MCF7 breast cancer cells, respectively (Citation44). In other cases, fruit waste such as husks and seeds are also used to produce gold nanoparticles, having even more value when using a residue for grape peel synthesis (Vitis vinifera). For the synthesis of gold nanoparticles with cytotoxic activity against A431 skin cancer cells, the particles obtained presented spherical morphologies with diameters of 20–80 nm (Citation45). The seed extract of mango (Mangifera indica), which is considered as waste and generally thrown away into the environment, was used for the synthesis of AuNPs with different morphologies (round, triangle, and irregular forms) and a diameter of 19.45 nm; they present a dose–response antimicrobial activity and cytotoxicity against HeLa cancer cell line, MCF-7 cells (Citation46).
2.2.2.1. Mechanistic aspects of green synthesis with plant, fruit and waste extracts
Generally, the synthesis of AuNPs with plant extracts involves the chemical reduction of Au+3 to Au0 by secondary metabolites present in the extract providing a rich arsenal of molecules with high redox potential (terpenoids, flavonoids, quinones, phytosterols, phenolic acids, aldehydes.), these components not only serve as reducing agents but also act as stabilizers to prevent agglomeration. Three phases in the AuNPs synthesis are recognized (): the ‘nucleation,’ where the Au0 atoms form small nuclei; the ‘growth phase,’ in which these small nuclei are grouped; and finally, the ‘capping’ where the oxidized secondary metabolites surround the surface of the AuNPs resulting in the stabilization of the nanoparticles (Citation34, Citation47, Citation48).
Liu et al., 2020 studied the effect of phenolic compounds and other components (protein and sugar), in reduction and capping on the green synthesis of small gold nanoparticles with 16 kinds of plants, the results showed that polyphenols present the strongest reductive and isotropic protective capability among all of the principal components, they present a concentration–response, high concentration increase the reductive capacity, but an excessively high polyphenols concentration was not conducive the synthesis of tiny particles because of the massive formation of carbonyl groups through the oxidation of phenolic hydroxyl groups (Citation49). They concluded that the optimal concentration of polyphenols is in a range of 0.04–0.08 mg/mL (Citation49).
As can be observed, there is a great variety of reports on the green synthesis of gold nanoparticles, in which different factors that influence the morphology and size of the particles obtained have been studied. Some of these factors are the concentration of precursor (HAuCl4), the concentration of the reductant (plant extract), and variations of pH and temperature; we can observe that different synthesis conditions will be required depending on the type of plant with which the extract is elaborated. However, summarizing the best conditions from other reports (Citation40–42, Citation7, Citation50–52), using HAuCl4 concentrations equal to or lower than 1 mM is recommended since the synthesis is little at lower concentrations and gold nanoparticles present small diameters. The added concentration of the extract will depend on the amount of reducing compounds present in the extract due to the synthesis include a redox reaction of gold (III) ions to gold atoms primarily by the polyphenols and amides present in the extract, whose concentration and form will have an impact on the synthesis time and the size of the nanoparticle obtained. The use of acid or neutral pH controlled the shape, size, and stability of AuNPs at temperatures between 60–90°C since temperature increased the nucleation of Gold nanoparticles, decreasing the diameters, as shown in ; these factors affect the morphology and size of the synthesized particles and, therefore, the biological activity they present (Citation41, Citation53).
The gold nanoparticles are used for different purposes like antimicrobial activity, sensors, and cancer therapy; in all the presented reports (Citation52–56), their activities are proved with excellent results. However, it is necessary to compare the antimicrobial or carcinogenic activity of the AuNPs obtained by green synthesis with nanoparticles obtained by traditional methods. A study of the stability of AuNPs in diverse conditions is needed to evaluate if the synthesis with natural compounds presents a synergistic effect with AuNPs, along with a complete study of the potential toxicity and accumulation of these particles in humans.
3. Principal advantages of the use of green methods for gold nanoparticles synthesis
Despite recent developments in green methods, chemical synthesis is still the most widely used. However, it has several disadvantages: the generation of toxic waste, expensive and laborious methods, and the necessary addition of a stabilizing agent to prevent agglomeration, sedimentation, and consequent loss of biological activity. In contrast, when green practices synthesize the AuNPs, there is no generation of toxic compounds since the plant's natural components are responsible for reducing the metal salts, being one of its main advantages. On the other hand, these compounds, besides lowering, are responsible for stabilizing the particles when they get to the surface. These methods are easy to scale and are relatively simple and economical. In addition to these advantages, there can be synergy to medicinal plant extracts that increase the cytotoxic activity and allow the AuNPs to enter more easily inside the cell (Citation57–59).
Other important advantages of the use of plant extract are biocompatibility of the nanoparticles obtained, necessary for application in biology and medicine, availability of the plants, cost-effective method because of minimum or non-requirement of energy, simplicity in scaling up for large-scale synthesis, reproducibility in production and well-defined morphology of synthesized nanoparticles (Citation60). For all of this reason, in the last years, green synthesis to produce AuNPs is increasing.
4. Gold nanoparticles in cancer therapy
Cancer is a disease characterized by abnormal cell growth, unrestricted, and invasiveness. It is considered one of the leading causes of death worldwide, standing as the third leading cause of mortality. The World Health Organization (WHO) reported millions of deaths in 2017 and is expected to increase, with an estimated 12 million deaths by 2030 (Citation61,Citation62). Cancer has different causes, but the most often are mutations or alterations in the expression patterns of proto-oncogenes, tumor suppressor genes, and those involved in DNA repair, caused by environmental factors, such as exposure to radiation and pollutants, and unhealthy lifestyles, including lack of physical activity, unbalanced diet, smoking, stress, age and inherited genetics (Citation63). The treatment depends on the type of cancer, the stage of the patient, and the patient’s ability to withstand the given therapy. However, generally, the treatment is based on surgery and chemo or radiation therapy to kill the cancer cells; the last therapies often result in several side effects like anemia, appetite loss, delirium, diarrhea, fatigue, hair loss, pain, and nausea (Citation61,Citation64). In this sense, there is a high demand for the development of different treatments and strategies for cancer; in the last year, there is an exceptional growth in nanotechnology for this purpose. Gold nanoparticles are good candidates for cancer detection and therapy due to their physicochemical properties and innovative potentiality, different reports of using these particles for some cancer treatment (). It can be observed that diverse paths are followed for their use, either in combination with other treatments such as radiotherapy, drug carriers, or their use alone as a treatment; in each of these paths, they present a different mechanism that we can see in (Citation65–67).
Table 2. Use of gold nanoparticle for cancer treatment.
There are different reports of the use of gold nanoparticles made by green synthesis that have been used for different types of cancer. Like in the case of the use of an extract from the leaves of the Jasminum auriculatum shrub that served as a reducing and stabilizing agent in the development of spherical AuNPs with diameters of 8–37 nm, and that presented a dose-dependent inhibitory effect of cell proliferation of a cervical cancer line (Citation18). An extract of Anacardium occidentale or cashew leaves is used to synthesized 40 nm spherical AuNPs that decreased the cellular viability of the MCF-7 lines (breast cancer) and were selective for mononuclear blood cells (Citation68). AuNPs were synthesized with leaf extracts of Tasmannia lanceolata and Backhousia citriodora. For both extracts, spherical particles with diameters between 7–14 nm were obtained, both showed cytotoxic activity in breast, liver, and skin cancer cells; however, those synthesized with Tasmannia lanceolata were more effective (Citation69).
5. Mechanism of action of gold nanoparticles in cancer therapy
5.1. Photo-thermal therapy
Photothermal therapy is a method for cancer treatment in where the absorbed light turns into heat. This increment in temperature results in photoablation followed by cell death; different techniques can be used to generate heat, such as laser, microwave, ultrasound, and radiofrequency waves; the principal's limitations of this method is the damage in normal cells surrounding the tumor and the low absorption of radiation in tumors (Citation70,Citation71). The use of gold nanoparticles as photothermal agents is an emerging topic, and they are used because they have a unique characteristic of absorbing and scatter light in the visible region of the spectrum, which allows them to amplify the optical properties of the excitation light and thus increase the effectiveness of light-based photothermal tumor ablation (Citation72,Citation73). The mechanism of AuNPs enhance this therapy includes the collision of laser radiation and excitation of the particles; as a result of excitation, fast local heat is generated and transferred to the cell in picoseconds, the effectiveness of AuNPs depends on different factor like shape and size (Citation74,Citation75). Gold nanoparticles loaded with curcumin were developed to increase photothermal therapy's efficacy (808 nm diode) on breast cancer cell lines (4T1); combining AuNPs and 808-nm laser has a better destruction effect on 4T1 breast cancer compared to laser irradiation of 650 nm. The use of infrared lights in the range of 700–1000 nm in phototherapy is preferable to other lights because of the minimal absorption of proteins and DNA and deep penetration of lights in tissues (Citation65). represent the possible mechanism of AuNPs in the different treatments.
5.2. Radiotherapy
Radiotherapy is the method of ionizing radiation delivered to the tumor via an external beam (X-ray or γ-ray beam) or from an internally placed radiation source (brachytherapy). The exposure can directly induce DNA damage to suppress tumor growth and destroy tumor tissues, on the other hand, directly or indirectly via ionization of molecules (e.g. water) within the cells, generating a cascade of free radicals that conclude in cell death. The limitation of this method is the damage to healthy tissues that occur in parallel; as a result, the dose of radiation administrated must be limited to keep normal tissue toxicities at a tolerable level, the consequent insufficient DNA damage to tumors cannot destroy the tumor, which may result in RT resistance. To evade this limitation, can be use radiosensitizers that concentrate the radiation on the tumor (Citation64,Citation77), one of these sensitizers was the gold nanoparticles for their unique optical characteristics; different mechanism can be proposed for the enhanced effectiveness of radiotherapy by AuNPs: first, the Physical enhanced, based in the high atomic number of the AuNPs (Z = 79) that provides a large X-ray absorption cross-section; therefore can improve the effectiveness by increasing the radiation sensitivity of the tumor and granting the reduce of the radiation dose; second the Chemical sensitization of DNA to radiation, small AuNPs that are capable of nuclear localization and tight electrostatic binding to DNA were recommended to enable full exploitation of the chemical enhancement and finally AuNPs have been shown to induce the formation of Reactive Oxygen Species (ROS). The resulting oxidative stress has emerged as one of the central mechanisms of nanoparticle-induced cytotoxicity (Citation78). Polymeric gold-photoactive nanoparticles (PGPNPs) conjugated with folic acid (FA) was evaluated in combination with radiofrequency therapy against prostate cancer (LNCaP) cells line, there were treated with gold nanoparticles and ionizing radiation, and the synergistic effect of treatment methods was evaluated by colony formation assay (CFA) and Flow cytometry analysis. The results demonstrated that combinatorial therapy of polymeric gold nanoparticles and ionizing radiation at various doses (2, 4, and 6 Gy) had a synergistic effect on survival fraction and apoptotic induction necrotizing cell death (Citation79).
5.3. Drug delivery
Chemotherapy is one of the most commonly used treatments for cancer but presents different limitations, mainly due to the several side effects resulting from the non-specific interactions of drugs with tumor and normal cells, low solubility, and poor biodistribution. One of the promising approaches for the disadvantages is applying drug delivery systems, which could provide a more efficient targeted transport of molecules, increase the solubility, improve the biodistribution and prevent fast removal of the therapeutic from the organism (Citation63,Citation80,Citation81). AuNPs represent an excellent alternative for delivering different anticancer drugs because of specific properties that include chemical resistivity, enzymatic stability, and low cytotoxicity. On the other hand, AuNPs are biocompatible, can form stable complexes with DNA and small interfering RNA (siRNA), and present the possibility of covalent bonding with therapeutics and targeting molecules (enzymes, peptides) on the surface (Citation62,Citation82). L-asparaginase was loaded on AuNPs (prepared by chemical synthesis) along with Arg-Gly-Asp (RGD) peptide, with the aim to enhancing the anticancer efficiency of the compound in the MCF-7 breast cancer cell line. The conjugate exhibited high tumor-targeting efficacy and distribution in the tumor cells, caused a significant decrease in cell proliferation rate and clonogenicity, initiated apoptosis, and promoted cell cycle arrest. In contrast, the conjugated lower cytotoxicity in a regular cell line represents a significant advantage in using AuNPs as drug delivery systems (Citation83).
5.4. Therapeutic
The AuNPs can be used as therapeutic molecules for the medical treatment of cancer; in different studies, gold nanoparticles exhibit cytotoxic properties against certain types of cancer cell lines, the mechanism of this is not fully described, and their use as anticancer therapeutics requires further research. AuNPs are considered as a carrier for photo components and may act as an anticancer agent. On the other hand, AuNPs can interact with the cell membrane and internalize in the cell, showed cytotoxic activity via ROS production, DNA damage and activation caspase cascade of apoptosis, mitochondrial dysfunction, and some changes in the structure by cell clumping, cell rupture, inhibition of cell growth and loss of membrane stability (). Another important mechanism of the anticancer properties of AuNPs is the inhibition of angiogenesis in cells; Angiogenesis is the formation of new blood vessels from pre-existing vessels and activation, migration, proliferation, and differentiation of endothelial cells. Angiogenesis is important for tumor growth because it depends on the blood vessel to provide a constant flow of nutrients and oxygen, crucial for cancer progression and metastasis (Citation84–86), presents a brief representation of the possible mechanisms of cytotoxic action in cells that were mentioned. When AuNPs are used as a therapeutic agent per se, green synthesis is preferred over to the traditional methods because the material obtained with traditional synthesis can be highly toxic for living organisms in contrast with green procedures that reduce waste production and increase their safety (Citation87,Citation88). Hence, in recent years, there has been an increase in research focusing on developing gold nanoparticles mediated with plant extracts, and there was probed with different types of cancer cell lines, as can be seen in (Citation89). One example of that is reported by Uzma et al. in 2020, who developed AuNPs using the aqueous extract of Commiphora wightii. These nanoparticles were evaluated for the anticancer activity against breast cancer cell MCF-7, finding that they present cytotoxicity at low concentrations with an IC50 of 66.11 μg/mL and significantly affect the induction of apoptosis (Citation90).
Table 3. Gold nanoparticles synthesized with plant extracts used in different types of cancer.
6. Application of green synthesis of gold nanoparticles in colon cancer
Colon cancer is one of the majority prevailing forms of cancer worldwide, and one of the leading causes of cancer relates to death, despite all the efforts and advances in diagnostics and treatment. Colon carcinogenesis is separated into three stages: initiation, promotion, and progression, during which the normal colonic cells undergo a pathological conversion into an adenoma, carcinoma, and ultimately invasive and metastatic cancer. Colon cancer has been associated with numerous lifestyle factors, including a sedentary lifestyle, a low-fiber and/or high fat diet, heavy alcohol consumption, smoking, obesity. People with type 2 diabetes or insulin resistance and chronic inflammatory diseases such as ulcerative colitis and Crohn's disease are at greater risk of developing colon cancer (Citation50,Citation51); for this reason the development of therapies that promote apoptosis and inhibit cell proliferation with minimal or no side effects and toxicities are of great importance, one of this new treatment was the metallic nanoparticles, principally gold nanoparticles have been broadly examined because their multiples characteristics in colon cancer therapy, different research present excellent results of the use of this nanoparticles in colon cancer cell lines, this nanoparticles were synthetized with medicinal plants that present different compounds serving as reducing and stabilizing agents, as mentioned above (Citation52–54), some examples of this are the AuNPs synthetized by the reduction of gold ions with Cystoseira baccata extracts, the nanoparticles obtained was spherical, stable, polycrystalline nanoparticles with mean diameter of 8.4 nm, this nanoparticles was probed against colon cancer cell lines HT-29 and Caco-2, the results exhibit a high cytotoxic effect in this cell line Caco-2 in different doses (400, 200, 100 and 50 μM) obtained a percentages of cell viability of 38% with the higher concentration and IC50 value of 79.03 μM; in the case of HT29 the cytotoxicity was significant with a rates of cell viability of 11.75% and a IC50 value of 49.61 μM; the principal mechanism of cytotoxicity is the apoptotic activation by the extrinsic and mitochondrial pathway (Citation55). Another example is the reported spherical AuNPs synthesis with Albizia lebbeck leaf extract. They presented a 20–30 nm diameter and probed against HCT-116 colon cancer cell line; the cell viability was reduced with rising concentrations of the biosynthesized AuNPs. The inhibitory concentration (IC50) level of the HCT-116 cells was identified as 48 μg/ml, the mechanism of cytotoxicity corresponds to the production of ROS and leading to cellular deaths. ROS overproduction was disturbing the signal transduction pathways, which also leads to cellular apoptosis (Citation56). Abutilon indicum leaf extract was used to synthesize gold nanoparticles with spherical shape and diameters of 1–20 nm; the particles exhibit a high cytotoxicity effect against HT-29 colon cancer cells IC50 value of 210 and 180 μg/mL after 24 and 48 h of treatment. The cytotoxicity effect is principally defined by the production of reactive oxygen species and the simultaneous reduction in cellular antioxidants, which might have caused mitochondrial membrane potential loss, DNA damage and G1/S phase cell cycle arrest. On the other hand, the particles can induce nuclear morphological changes, cell membrane damage, and different enzymes expression in the apoptosis pathways (Citation91). Other important examples were presented in .
Table 4. Green gold nanoparticles tested in colon cancer cell lines.
In most of the reports, colon cancer inhibition's typical mechanism is ROS and oxidative stress production, which induce the upregulation of caspase-3 and oxidation of glutathione (GSH) to glutathione disulfide (GSSG). Moreover, in some reports, the G2/M or sub-G1 cell cycle arrest has been proposed to induce apoptosis upon treatment with biogenic AuNPs, which may further help explain the anticancer mechanism in depth. The shape, size, and plant extract used for the synthesis have an essential role in the anticancer activity, principally for the interaction with cell membrane and internalization, in this sense is preferred spherical nanoparticles with small sizes, similar to the obtained in the majority of the reports described in this review (Citation92,Citation93). It is vital to carry out comparison studies with nanoparticles produced by chemical means and the bulk extract to determine an increase in activity and improvements in the internalization and delivery of compounds to the cell's interior.
7. Toxicity
Due to the diverse application of AuNPs, the study of their toxicity and accumulation in different human organs has become paramount. To assure consumers that their use is safe, several authors have studied the toxicity AuNPs (size and morphology) in other models (Citation94). Enea et al., Citation2021 evaluated the safety of different shapes and sizes of gold nanoparticles in human HepaRG cells and primary rat hepatocytes (PRH). Among all the tested nanoparticles, the smaller 15 nm spheres displayed the highest toxicity and increment when the nanoparticles were capping with citrate, the AuNPs that present the lower toxicity are the nanostars with 60 nm of diameter; the results obtained suggest that it is essential to use appropriate in vitro cell models for testing the liver biocompatibility. PRH is more efficiently internalized AuNPs when compared with HepaRG cells, and the uptake proved to be time-dependent and highly dependent on the shape, size, and capping agent (Citation95). Engstrom et al., 2020 reported the toxicity in vivo of three sizes of AuNPs (5, 10 nm y 20 nm) in a model of Embryonic Zebrafish. The result showed that 5 nm AuNPs present rapid toxicity with significant 24 hpf mortality occurring at concentrations ≥20 mg/L, whereas the 10 and 20 nm AuNPs showed no considerable mortality throughout the five-day experiment (Citation96). Khoshnamvand et al., 2020 evaluated the toxicity of green AuNPs synthesized with an aqueous extract of Saccharina japonica in an In vivo model of Daphnia magna, the nanoparticles obtained present two sizes 72 and 10 nm and showed an IC50 of 1.57 ± 0.07 y 2.69 ± 0.12 mg/L respectively, indicating that toxicity is closely related to size. After exposure of daphnids to treatments, AuNPs were accumulated in the gut tract, and lipid droplets under the Daphnia carapace (Citation97). Isoda et al., 2020 reported the toxicity of AuNPs in combination with the administration of drugs (cisplatin, paraquat, and 5-amino salicylic acid) in a Mice model; the sizes evaluated were 10, 50, and 100 nm and the drugs. The AuNPs administered alone no exhibit hepatotoxicity or nephrotoxicity; in contrast, coadministration of gold nanoparticles (10 nm) with cisplatin, paraquat, or 5-aminosalicylic acid caused side-effect damage to the kidney (Citation98). In these studies, we can observe that the toxicity is very dependent on the morphological characteristics of the nanoparticles obtained, and it is possible to follow cell death and accumulation of the same in the organisms used. For this reason, toxicity studies are essential to use this type of particle in different areas such as cancer treatment.
8. Conclusion and future perspectives
Gold nanoparticles are one of the most used metal particles due to their unique biocompatibility, optical and cytotoxic characteristics; different methods can manufacture these particles. However, green synthesis with plant extracts has become one of the main routes for their synthesis, being an easy and friendly method with the environment. Various plant extracts have been used to develop AuNPs with multiple applications from antimicrobial, catalytic, and anticancer; in the latter, the particles have different areas of opportunity when used as photo-thermal agents, radiation sensitizers, drug carriers, and as therapeutic agents on their own. Several studies have been carried out on the synthesis of AuNPs with plant extracts for their use against different cancer lines, observing cytotoxic effects at low concentrations and differentiation with healthy cells. However, the mechanisms that carry out this decrease in Cell viability have not yet been fully elucidated, so new studies have been required that focus on the mechanism of action.
Acknowledgements
The authors thank to Mexican National Council for Science and Technology (CONACYT) for a postgraduate fellowship to Karen M. Soto.
Disclosure statement
No potential conflict of interest was reported by the authors.
References
- Rana, A.; Yadav, K.; Jagadevan, A. J. Clean. Prod. 2020, 272, 122880.
- Kamal, R.; Chadha, V.D.; Dhawan, D.K. Nanomed-nanotechnol. 2018, 14 (3), 1059–1071.
- AlSalhi, M.S.; Aziz, M.H.; Atif, M.; Fatima, M.; Shaheen, F.; Devanesan, S.; Aslam Farooq, W. J. King Saud Univ. Sci. 2020, 32, 1395–1402.
- Venkatadri, B.; Shanparvish, E.; Rameshkumar, M.R.; Arasu, M.V.; Al-Dhabi, N.A.; Ponnusamy, V.K.; Agastian, P. Saudi J. Biol. Sci. 2020, 27, 2980–2986.
- Manikandan, M.; Hasan, N.; Wu, H.F. Biomaterials. 2013, 34, 5833–5842.
- Zhang, C.; Bu, W.; Ni, D.; Zhang, S.; Li, Q.; Yao, Z.; Zhang, J.; Yao, H.; Wang, Z.; Shi, J. Angew. Chem. Int. Ed. 2016, 55, 2101–2106.
- Sadhukhan, P.; Kundu, M.; Chatterjee, S.; Ghosh, N.; Manna, P.; Das, J.; Sil, P.C. Mater. Sci. Eng., C. 2019, 100, 129–140.
- Wang, Y.; Xu, J.; Shi, L.; Yang, H. J. Cell. Physiol. 2020, 235, 8951–8957.
- De Matteis, V.; Rizzello, L.; Cascione, M.; Liatsi-Douvitsa, E.; Apriceno, A.; Rinaldi, R. Nanomaterials. 2020, 10, 10061083.
- Menon, S.; Rajeshkumar, S.; Kumar, V. Resour. Technol. 2017, 3, 516–527.
- Khan, T.; Ullah, N.; Khan, M.A.; Mashwani, Z.; Nadhman, A. Adv. Colloid Interface Sci. 2019, 272, 102017.
- Bapat, R.A.; Chaubal, T.V.; Dharmadhikari, S.; Abdulla, A.M.; Bapat, P.; Alexandar, A.; Dubey, S.K.; Kesharwani, P. Int. J. Pharm. 2020, 586, 119596.
- Wang, Y.; Wang, Y.; Xu, C.; Liu, Y.; Huang, Z. Mol. Ther. Nucleic Acid. 2020, 22, 236–250.
- Venkatadri, B.; Shanparvish, E.; Rameshkumar, M.; Arasu, M.; Al-Dhabi, N.A.; Ponnusamy, V.K.; Agastian, P. Saudi J. Biol. Sci. 2020, 27, 2980–2986.
- García-Osogobio, S.; Téllez-Ávila, F.; Méndez, N.; Uribe-Esquivel, M. Endoscopia. 2015, 27 (2), 59–63.
- Lazzarini, R.; Alcántar-Ramírez, O.; Jaime-Cruz, R. Mundo Nano UNAM. 2015, 8, 96–117.
- Weng, W.; Goel, A. Semin. Cancer Biol. 2020, 20, S1044–579X.
- Balasubramanian, S.; Jelastin, S.M.; Pushparaj, T.L. J. Drug Deliv. Sci. Technol. 2020, 57, 101620.
- Hosseinzadeh, N.; Shomali, T.; Hosseinzadeh, S.; Raouf, F.; Pourmontaseri, M.; Fazeli, M. J. Pharm. Pharmacol. 2020, 72, 1013–1025.
- Kyziol, A.; Lukasiewicz, S.; Sebastian, V.; Kuśtrowski, P.; Koziel, M.; Majda, D.; Cierniak, A. J. Inorg. Biochem. 2021, 214, 111300.
- Chen, H.; Zhou, K.; Zhao, G. Trends Food Sci. Technol. 2018, 78, 83–94.
- Kalimuthu, K.; Cha, B.S.; Kim, S.; Park, K.S. Microchem. J. 2020, 152, 104296.
- Zhang, S.; Geng, Y.; Ye, N.M.; Xiang, Y. Microchem. J. 2020, 158, 105190.
- Agrawal, A.; Varshney, R.; Gattani, A.; Kirthika, P.; Khan, M.H.; Singh, R.; Kodape, S.; Patel, S.; Singh, P. J. Microbiol. Methods. 2020, 177, 106024.
- Khalid, S.; Parveen, S.; Shah, M.; Rahim, S.; Ahmed, S.; Imram, M. Arab. J. Chem. 2020, 13, 3988–3996.
- Lokesh, K.; Shambhulinga, A.; Manjunatha, N.; Imadadulla, M.; Hojamberdiev, M. Dye. Pigment. 2015, 120, 155–160.
- García Calavia, P.; Chambrier, I.; Cook, M.; Haines, A.; Field, R.; Russell, D. J. Colloid Interface Sci. 2018, 512, 249–259.
- Boonyuen, U.; Praoparotai, A.; Chamchoy, K.; Swangsri, T.; Warakulwit, C.; Suteewong, T. Anal. Chim. Acta. 2020, 1122, 61–69.
- Kumari, Y.; Kaur, G.; Kumar, R.; Singh, S.K.; Gulati, M.; Khursheed, R.; Clarisse, A.; Gowthamarajan, K.; Karri, V.; Mahalingam, R.; Ghosh, D.; Awasthi, A.; Kumar, R.; Yadav, A.; Kapoor, B.; Singh, P.; Dua, K.; Porwal, O. Adv. Colloid Interface Sci. 2019, 274, 102037.
- Zhao, P.; Li, N.; Astruc, D. Coord. Chem. Rev. 2013, 257, 638–665.
- Daruich De Souza, C.; Ribeiro, B.; Rostelato, M. J. Alloys Compd. 2019, 798, 714–740.
- Liu, Z.; Lanier, O.L.; Chauhan, A. Nanomaterials. 2020, 10, 1–16.
- Dou, X.; Wang, X.; Qian, S.; Liu, N.; Yuan, X. Nanoscale. 2020, 12, 19855–19860.
- Teimouri, M.; Khosravi-Nejad, F.; Attar, F.; Akbar, A. J. Clean. Prod. 2018, 184, 740–753.
- Majoumouo, M.S.; Sharma, J.R.; Sibuyi, N.R.; Tincho, M.B.; Boyom, F.; Meyer, M. Molecules. 2020, 25, 1–18.
- Rajasekar, T.; Karthika, K.; Muralitharan, G.; Maryshamya, A.; Sabarika, S.; Anbarasu, S.; Revathy, K.; Prasannabalaji, N.; Kumaran, S. Brazilian J. Microbiol. 2020, 51, 957–967.
- Noruzi, M. Bioprocess Biosyst. Eng. 2015, 38, 1–14.
- Raju, D.; Mehta, U.J.; Ahmad, A. Biotechnol. Appl. Biochem. 2012, 59, 471–478.
- Islam, N.; Jalil, K.; Shahid, M.; Rauf, A.; Muhammad, N.; Khan, A.; Shah, M.; Khan, M. Arab. J. Chem. 2019, 12, 2914–2925.
- Botteon, C.E.; Silva, L.B.; Ccana-Ccapatinta, G.; Silva, T.; Ambrosio, S.; Veneziani, R.; Bastos, J.; Marcato, P. Sci. Rep. 2021, 11, 1–16.
- Thangamani, N.; Bhuvaneshwari, N. Chem. Phys. Lett. 2019, 732, 136587.
- Satpathy, S.; Patra, A.; Ahirwar, B.; Hussain, M. Phys. E Low-Dimens. Syst. Nanostructures 2020, 121, 113830.
- Kumar, H.; Bhardwaj, K.; Dhanjal, D.S.; Nepovimova, E.; Șen, F.; Regassa, H.; Singh, R.; Verma, R.; Kumar, V., Kumar, D., et al. Int. J. Mol. Sci. 2020, 21, 1–18.
- Hyun, K.; Soshnikova, V.; Markus, J.; Ju, Y.; Chul, S.; Singh, P.; Castro-Aceituno, V.; Ahn, S.; Hyun, D.; Shim, Y.; Jin, K.; Chun, D. Artif. Cells Nanomed. 2018, 46 (3), 599–606.
- Nirmala, J.G.; Akila, S.; Narendhirakannan, R.T.; Chatterjee, S. Adv. Powder Technol. 2017, 28, 1170–1184.
- Savan, D.; Ram, G.; Chanda, S. Artif. Cells Nanomed. 2020, 48 (1), 1315–1325.
- Soto, K.M.; Quezada-Cervantes, C.; Hernández-Iturriaga, M.; Luna-Bárcenas, G.; Vazquez-Duhalt, R.; Mendoza, S. LWT-Food Sci and Tech. 2019, 103, 193–300.
- Xin, L.K.; Shameli, K.; Miyake, M.; Kuwano, N.; Ahmad, N.; Mohamad, S.; Yew, Y. J. Nanomater. 2016, 2016, 1–7.
- Liu, H.; Zhang, X.; Xu, Z.; Wang, Y.; Ke, Y.; Jiang, Z.; Yuan, Z.; Li, Y. Nanotechnology. 2020, 31, 1–12.
- Shrivastava, P.; Sharma, R.; Gautam, L.; Vyas, S.; Vyas, P.; Awesh, K.; Gupta, U.; Sharma, R. Elsevier Academic Press. 2020, Chapter 8, 191–223.
- Ruan, H.; Leibowitz, B.J.; Zhang, L.; Yu, J. Mol. Carcinog. 2020, 59, 783–793.
- Firdhouse, M.J.; Lalitha, P. IET Nanobiotechnology. 2020, 14 (3), 224–229.
- Kumar, G.; Ghosh, M.; Pandey, D. IET Nanobiotechnology. 2019, 13, 626–633.
- Klebowski, B.; Depciuch, J.; Stec, M.; Krzempek, D.; Komenda, W.; Baran, J.; Parlinska-Wojtan, M. Int. J. Mol. Sci. 2020, 21 (24), 9610.
- González-Ballesteros, N.; Prado-López, S.; Rodríguez-González, J.B.; Lastra, M.; Rodríguez-Argüelles, M.C. Colloids Surf. B: Biointerfaces. 2017, 153, 190–198.
- Malaikolundhan, H.; Mookkan, G.; Matheswaran, N.; Alsawalha, M.; Priya, V.; Mohan, S.K.; Di, A. Artif. Cells, Nanomedicine, Biotechnol. 2020, 48, 1206–1213.
- Ovais, M.; Khalil, A.; Raza, A.; Islam, N.; Ayaz, M.; Saravanan, M.; Ali, M.; Ahmad, I.; Shahid, M.; Shinwari, Z. Appl. Microbiol. Biotechnol. 2018, 102, 4393–4408.
- Nadeem, M.; Abbasi, B.; Younas, M.; Ahmad, W.; Khan, T. Green Chem Lett Rev. 2017, 10, 216–227.
- Barabadi, H.; Kamali, D.; Shoushtari, F.; Tajani, B.; Mahjoub, M.; Alizadeh, A.; Saravanan, M. J. Clust. Sci. 2019, 30, 1375–1382.
- Baruaha, D.; Goswami, M.; SinghYadav, R.; Yadav, A.; Das, A. J. Photochem. Photobiol. B. 2018, 186, 51–58.
- Singh, P.; Pandit, S.; Mokkapati, V.M.; Garg, A.; Ravikumar, V.; Mijakovic, I. Int J Mol Sci. 2018, 19, 1979.
- Peng, J.; Liang, X. Medicine. 2019, 98, 15313.
- Sztandera, K.; Klajnert-Maculewicz, B. Mol. Pharmaceutics. 2019, 16, 1–23.
- Nagi, N.M.S.; Khair, Y.; Abdalla, A.M.E. Jpn. J. Radiol. 2017, 35, 555–561.
- Bai, X.; Wang, Y.; Song, Z.; Feng, Y.; Chen, Y.; Zhang, D.; Feng, L. Int. J. Mol. Sci. 2020, 21, 72480.
- Park, S.Y.; Beomjim, K.; Cui, Z.; Park, G.; Choi, Y.W. Int. J. Nanomedicine. 2020, 15, 5317–5331.
- Fan, M.; Han, Y.; Gao, S.; Yan, H.; Cao, L.; Li, Z.; Liang, X.J.; Zhang, J. Theranostics. 2020, 10, 494–497.
- Sunderam, V.; Thiyagarajan, D.; Lawrence, A.V.; Mohammed, S.; Selvaraj, A. Saudi J. Biol. Sci. 2018, 26, 455–459.
- Khandanlou, R.; Murthy, V.; Wang, H. Mater. Sci. Eng. C. 2020, 112, 110922.
- Erkol, H.; Yelken, S.Z.; Algarawi, M.; Gulsen, G.; Nouizi, F. Int. J. Heat Mass Transf. 2020, 163, 120438.
- Vines, J.B.; Yoon, J.H.; Ryu, N.E.; Lim, D.J.; Park, H. Front. Chem. 2019, 7, 167.
- Liu, Y.; Chongssathid, P.; Crawford, B.M.; Odin, R.; Dechant, C.A.; Kemeny, H.; Cui, X.; MacCarini, P.; Lascola, C.; Fecci, P.; Vo-Dinh, T. Immunotherapy. 2019, 11, 1293–1302.
- Zhang, R.; Wang, L.; Wang, X.; Jia, Q.; Chen, Z.; Yang, Z.; Ji, R.; Tian, J.; Wang, Z. Adv. Healthc. Mater. 2020, 9, 1–10.
- Norouzi, H.; Khoshgard, K.; Azbarzadeh, F. Lasers Med. Sci. 2018, 33, 917–926.
- Kang, M.S.; Lee, S.; Kim, K.; Han, D. Pharmaceutics. 2020, 12, 1–22.
- Rahimi-Moghaddam, F.; Sattarahmady, N.; Azarpira, N. J. Biomed. Phys. Eng. 2019, 9, 473–482.
- Lu, S.L.; Liu, W.; Cheng, J.C.; Lin, L.C.; Wang, C.R.; Li, P. Adv. Drug Deliv. Rev. 2020, 21, 1–16.
- Her, S.; Jaffray, D.; Allen, C. Adv. Drug Deliv. Rev. 2017, 109, 84–101.
- Changizi, O.; Khoei, S.; Mahdavian, A.; Shirvalilou, S.; Mahdavi, S.; Keyvan, J. Photodiagnosis Photodyn. Ther. 2020, 29, 101602.
- Khoshnevisan, K.; Daneshpour, M.; Barkhi, M.; Gholami, M.; Samadian, H.; Maleki, H. J. Drug Target. 2018, 26, 525–532.
- Masse, F.; Desjardins, P.; Ouellette, M.; Couture, C.; Mahmoud, O.; Pernet, V.; Guérin, S.; Boisselier, E. Molecules. 2019, 24, 2929.
- Goddard, Z.R.; Marín, M.; Rusell, D.; Searcey, M. Chem. Soc. Rev. 2020, 49, 8774–8789.
- Al-dulimi, A.; Al-saffar, A.; Sulaiman, G.; Khalil, K.; Khawla, S.; Al-shmgani, H.; Ahmed, E. J. Mater. Res. Technol. 2020, 9, 15394–15411.
- Darweesh, R.; Ayoub, N.; Nazzal, S. Int. J. Nanomedicine. 2019, 14, 7643–7663.
- Ferreira, D.; Fontinha, D.; Martins, C.; Pires, D.; Fernandes, A.; Baptista, P. Molecules. 2020, 25, 153489.
- Donga, S.; Bhadu, G.; Chanda, S. Artif. Cells, Nanomedicine Biotechnol. 2020, 48, 1315–1325.
- Yadi, M.; Mostafavi, E.; Saleh, B.; Davaran, S.; Aliyeva, I.; Khalilov, R.; Nikzamir, M.; Akbarzadeh, A.; Panahi, Y.; Milani, M. Artif. Cells Nanomed. Biotechnol. 2019, 43, S336–S343.
- Gour, A.; Kumar, N. Artif. Cells Nanomed. Biotechnol. 2019, 47 (1), 844–851.
- Siddiqi, K.S.; Husen, A. J. Trace Elem. Med. Biol. 2017, 40, 10–23.
- Uzma, M.; Sunayana, N.; Raghavendra, V.B.; Madhu, C.S.; Shanmuganathan, R.; Brindhadevi, K. Process Biochem. 2020, 92, 269–276.
- Mata, R.; Nakkala, J.R.; Sadras, S. Colloids Surf. B: Biointerfaces. 2016, 143, 499–510.
- Tian, L.; Lu, L.; Qiao, Y.; Ravi, S.; Salatan, F.; Melacon, M. J. Funct. Biomater. 2016, 7, 19.
- Surapaneni, S.K.; Bashir, S.; Tikoo, K. Sci. Rep. 2018, 8, 12295.
- Moreno-Garrido, I.; Pérez, S.; Blasco, J. Mar. Environ. Res. 2015, 111, 60–73.
- Enea, M.; Pereira, E.; Costa, J.; Soares, M.E.; Dias da Silva, D.; Bastos, M.; Carmo, H.F. Toxicol. Vitr. 2021, 70, 105046.
- Engstrom, A.M.; Faase, R.; Marquart, G.; Baio, J.E.; Mackiewicz, M.R.; Harper, S.L. Int. J. Nanomedicine. 2020, 15, 4091–4104.
- Khoshnamvand, M.; Ashtiani, S.; Liu, J. Environ. Sci. Pollut. Res. 2020, 27, 22120–22126.
- Isoda, K.; Tanaka, A.; Fuzimori, C.; Echigoya, M.; Taira, Y.; Taira, I.; Shimizu, Y.; Akimoto, Y.; Kawakami, H.; Ishida, I. Nanoscale Res. Lett. 2020, 15, 033714.
- Bu, T.; Zhang, M.; Sun, X.; Tian, Y.; Bai, F.; Jia, P.; Bai, Y.; Zhe, T.; Wang, L. Sensors Actuators B. Chem. 2019, 299, 126969.
- Hulikere, M.; Joshi, C.; Danagoudar, A.; Poyya, J.; Kudva, A.K.; Dhananjaya, B. Process Biochem. 2017, 63, 137–144.
- Prakash, M.; Kang, M.; Niyonizigiye, I.; Singh, A.; Kim, J.; Bae, Y.; Kim, G. Colloids Surf. B: Biointerfaces. 2019, 183, 110455.
- Mubarakali, D.; Arunkumar, J.; Nag, K.H.; Sheiksyedishack, K.; Baldev, E.; Pandiaraj, D.; Thajuddin, N. Colloids Surf. B: Biointerfaces. 2013, 103, 166–173.
- Anad, K.; Gengan, R.M.; Phulukdaree, A.; Chuturgoon, A. J. Ind. Eng. Chem. 2015, 21, 1105–1111.
- Dhayalan, M.; Immanuel, M.; Denison, J.; Ayyar, M.; Gandhi, N.N. J. Photochem. Photobiol. B Biol. 2018, 183, 251–257.
- El-borady, O.M.; Ayat, M.S.; Shabrawy, M.A.; Millet, P. Adv. Powder Technol. 2020, 31, 4390–4400.
- Baruah, D.; Goswami, M.; Narayan, R.; Yadav, S.; Yadav, A. J. Photochem. Photobiol. B Biol. 2018, 186, 51–58.
- Vinosha, M.; Palanisamy, S.; Muthukrishnan, R. Process Biochem. 2019, 85, 219–229.
- Thounaojam, A.; Shaikh, I.N.; Vijay, M. Mater. Today Proc. 2020, 43, 2805–2809.
- Sk, I.; Khan, M.; Haque, A.; Ghosh, S.; Roy, D.; Homechuadhuri, S.; Alam, A. Curr. Res. Green Sustain. Chem. 2020, 3, 100006.
- Singh, H.; Du, J.; Yi, T. Artif. Cells, Nanomedicine Biotechnol. 2017, 45, 1310–1316.
- Boomi, P.; Ganesan, R.M.; Poorani, G.; Gurumallesh, H.; Ravikumar, S.; Jeyakanthan, J. Mater. Sci. Eng. C. 2019, 99, 202–210.
- Liu, Y.; Song, X.; Cao, F.; Li, F.; Wang, M.; Yang, Y.; Liu, M.; Liu, A.; Xin, H.; Wang, X. Int. J. Nanomedicine. 2020, 15, 2315–2322.
- Lydia, D.; Khusro, A.; Immanuel, P.; Ali, G.; Al-dhabi, G. J. Photochem. Photobiol. B Biol. 2020, 206, 111868.
- Kalimuthu, K.; Lubin, B.C.; Bazylevich, A.; Gellerman, G.; Shpilberg, O.; Luboshits, G.; Firer, M. J. Nanobiotechnology. 2018, 16, 1–13.
- Ji, Y.; Cao, Y.; Song, Y. Artif. Cells, Nanomedicine, Biotechnol. 2019, 47, 2737–2745.
- Wang, L.; Xu, J.; Yan, Y.; Liu, H.; Karunakaran, T.; Li, F. Artif. Cells, Nanomedicine, Biotechnol. 2019, 47, 1617–1627.
- Chahardoli, A.; Karimi, N.; Sadeghi, F.; Fattahi, A. Artif. Cells, Nanomedicine, Biotechnol. 2018, 46, 579–588.
- Zhang, X.; Tan, Z.; Jia, K.; Zhang, W.; Dang, M. Artif. Cells, Nanomedicine, Biotechnol. 2019, 47, 2171–2178.
- Soshnikova, V.; Kim, Y.J.; Singh, P.; Huo, Y.; Ahn, S.; Castro-Aceituno, V.; Kang, J.; Mathiyalagan, R.; Yang, D. Artif. Cells, Nanomedicine, Biotechnol. 2018, 46, 108–117.
- Liu, R.; Pei, Q.; Shou, T.; Zhang, W.; Hu, J.; Li, W. Int J Nanomedicine. 2019, 14, 4091–4103.
- Ismail, E.; Saqer, A.; Assirey, E.; Naqvi, A.; Okasha, R. Int. J. Mol. Sci. 2018, 19, 1–14.
- Benedec, D.; Oniga, I.; Cuibus, F.; Sevastre, B.; Stiufiuc, G.; Duma, M.; Hanganu, D.; Iacovita, C.; Stiufiuc, R.; Lucaciu, C. Int. J. Nanomedicine. 2018, 13, 1041–1058.
- Mohammed, A.; Thangam, R.; Madhan, B.; Alam, M. Nano-Structures and Nano-Objects. 2019, 19, 1–11.
- Chaturvedi, V.K.; Yadav, N.; Rai, N.; Ellah, N.; Hetta, H.; Singh, M. Molecules. 2020, 25, 133091.
- Han, X.; Jiang, X.; Guo, L.; Wang, Y.; Veeraraghavan, V.; Krishna, S.; Wang, Z.; Cao, D. Artif. Cells, Nanomedicine Biotechnol. 2019, 47, 3577–3584.
- Alwhibi, M.; Soliman, D.; Al-Khaldy, H.; Alonaizan, A.; Abdulhaq, N.; El-Zaidu, M.; AlSubeie, M. J. King Saud Univ.-Sci. 2020, 32, 3372–337.