ABSTRACT
It is imminent to explore new types of starting materials for natural deep eutectic solvents (NADESs). Herein, a series of natural aromatic organic acids as the hydrogen bond donor (HBD) and coumarin as the model hydrogen bond acceptor (HBA) were used to design and prepare NADESs. They are characterized by nuclear magnetic resonance (NMR) and attenuated total reflection flourier transformed infrared spectroscopy (ATR-FTIR), supported by quantum chemistry (QC) calculations. The liquid phase of the mixture is attributed to the non-ideal behavior of both components at most of the ratios. In addition, — presented the energy dynamic equilibrium of eutectic systems. Using solid–liquid phase diagrams and mean interaction energies obtained by the conductor like screening model for real solvent model (COSMO-RS), the predicting formability of DESs can be achieved by choosing the proper HBAs and HBDs. Finally, five green solvents, including mandelic acid-coumarin, salicylic acid-coumarin, benzoic acid-coumarin, hydroxybenzoic acid-coumarin, and cinnamic acid-coumarin, were obtained. Thus, the aromatic organic acid studied in this work is vital for the development of novel green eutectic systems.
GRAPHICAL ABSTRACT
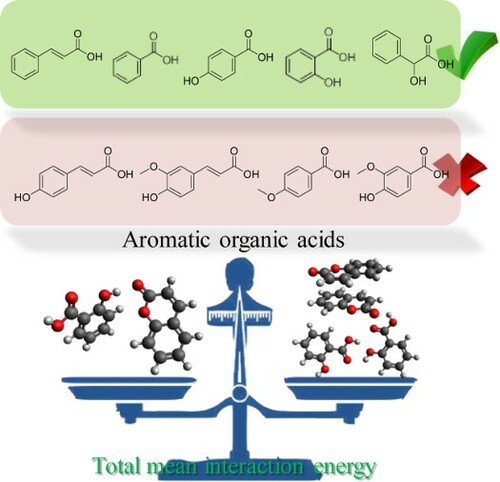
Introduction
With the development of green chemistry, designing a type of solvent that meets the 12 principles to replace traditional solvent is going to be a trend. Abbot et al. discovered that choline chloride and urea mixture could significantly decrease the melting point (Citation1). This type of solvent is called deep eutectic solvent (DES). Similar to DES, natural deep eutectic solvent (NADES) was also prepared by two components: hydrogen bond donor (HBD) and hydrogen bond acceptor (HBA) (Citation2). However, the NADES was a mixture of various abundant cellular constituents (metabolites), and many combinations of these compounds were liquids at low temperatures (Citation3). Compared with ionic liquid (IL), NADES is economic, biodegradable, and biocompatible, and it represents the green chemistry criteria fully (Citation4). It has become a new generation of solvents, and has a potential application in electrochemistry (Citation5), metal extraction (Citation5), material chemistry (Citation6), catalysis (Citation7), and organic synthesis (Citation8).
Eutectic solvents initially were divided into four main categories: metal chloride + quaternary ammonium salt (Type I), metal chloride hydrate + quaternary ammonium salt (Type II), HBD + quaternary ammonium salt (Type III), and HBD + metal chloride hydrate (Type IV). Recently, a new class has been identified that is composed of non-ionic compounds (Type V) (Citation9). Terpenes or terpenoids were usually employed as HBA or HBD, such as thymol, menthol, and other phenolic resources. This kind of NADES is often hydrophobic and can be utilized as a promising media in many water-contained applications, which is favorable for recycling and reusing solvents (Citation10). It is imminent to explore a new type of component in Type V DES. The characterization of their condensed phase and the investigation of their formation rule are also vital.
Herein, new NADES systems were designed and characterized. These solvents were prepared from a series of natural aromatic organic acids. Coumarin was applied as a representative HBA. The obtained eutectic systems were systematically characterized. Different theoretical models were used to predict thermo-physical properties of NADES and shed light on the inner liquefying mechanism. This work will pave the way for designing and screening novel green solvents.
Materials and methods
Preparation of deep eutectic solvents
Benzoic acid (Ben, ≥ 99%), trans-cinnamic acid (Cin, 99%), p-coumaric acid (Coua, 97%), trans-ferulic acid (Fer, 99%), p-hydroxybenzoic acid (Hyd, 99.5%), salicylic acid (Sal, 99.5%), 4-anisic acid (Ani, 99%), vanillic acid (Van, 98%), mandelic acid (Man, 99%) and coumarin (Cou, 99%) were procured from Shanghai Macklin Biochemical Technology Co., Ltd. NADESs were prepared by mixing the above bio-renewable sources at certain molar ratios (1:1–1:5). The mixtures were constantly stirred at 100°C until the homogeneous transparent liquids formed.
Characterization of deep eutectic solvents
The solid–liquid phase equilibria can be described by the following equation:
(1) where
is the mole fraction of the component
, and
is its activity coefficient,
is the fusion enthalpy of the component,
is the ideal gas constant,
is the melting temperature, and
is the system temperature. If we assume that the solvent is ideal, then
=1, and the ideal solid–liquid equilibrium phase diagram is obtained (Citation11).
All NADESs and their components were measured by Attenuated Total Reflection Flourier Transformed Infrared Spectroscopy (ATR-FTIR, IRTracer-100, SHIMADAU, Japan). The functional groups’ spectra were recorded in the range of 4000–400 cm−1 using the absorption mode with 50 scans at room temperature. The structures of eutectic mixtures were further confirmed by 1H nuclear magnetic resonance (1H NMR) spectroscopy. An Agilent DD2 spectrometer operating at 600 MHz was used at 25°C. Deuterated chloroform (CDCl3) was used as the solvent. The melting temperature of each system with different molar ratios was measured using a melting point device model SGWX-4A with a resolution of 0.1 K and a gradient of 1 K min−1 (Shanghai INESA Optical Instrument Co., Ltd).
Computational details
Geometries of molecules and NADES complexes were fully optimized at the B3LYP/def2-TZVPD level with Grimme’s DFT-D3(BJ) empirical dispersion correction using BIOVIA Tmolex 2021 (version 21.0.0). The symmetry-adapted perturbation theory (SAPT) was used to decompose binding energy (BE) into various physical parts for an in-depth understanding the nature of hydrogen bonds (Citation12, Citation13). SAPT analyses were done at the SAPT2+/aug-cc-pVDZ level by the PSI4 code without basis set superposition error (BSSE) correction (Citation14). Different energies of interaction and thermodynamic property were calculated by the software BIOVIA COSMOtherm 2020 (version 20.0.0). The total mean interaction energy (E) can be described by Equations (2)–(5)
(2)
(3)
(4)
(5) where
,
, and
are misfit, hydrogen bonding, and van der Waals interaction energy, respectively;
is the screening charge density;
is the effective contact area between two surface segments, and
,
,
,
are adjustable parameters;
and
are the element-specific adjustable parameters (Citation15).
Results and discussion
Solid–liquid phase diagram analysis
Nine natural aromatic organic acids were chosen as HBDs: benzoic acid, trans-cinnamic acid, p-coumaric acid, trans-ferulic acid, p-hydroxybenzoic acid, salicylic acid, 4-anisic acid, vanillic acid, and mandelic acid. Coumarin was chosen as the model HBA. Their structures and melting properties are given in . The conductor like screening model for a real solvent model (COSMO-RS) was used to estimate the solid–liquid equilibrium conditions due to its accurate description of non-ideality in DES (Citation16). The predicted phase diagrams with the ideal liquidus curve are shown in . Moreover, the experimental eutectic points of Sal-Cou (salicylic acid-coumarin) and Ben-Cou (benzoic acid-coumarin) at different mole fractions were examined. Melting temperature depression in all systems generally can be found. More specifically, coumarin shows the melting temperature depression effect because of low melting enthalpy (the right side of diagrams). However, it is interesting to notice that four HBDs, including Coua (p-coumaric acid), Fer (trans-ferulic acid), Ani (4-anisic acid), and Van (vanillic acid, the left side of diagrams) behave ideally when mixed. These demonstrate that molecular interactions in the mixture are of the same or similar strength to that present in the pure substances (Citation17). Cin (trans-cinnamic acid), Ben (benzoic acid), Sal (salicylic acid), and Man (mandelic acid) interact with HBAs by causing deviations to thermodynamic ideality and Hyd (p-hydroxybenzoic acid) with mild deviations to ideality at high coumarin concentration. The negative deviations lead to phase diagrams with regions where the melting temperatures of the systems are close (Citation18). In particular, this results in a larger liquidus region (Citation18), as shown in the experimental data of mixtures Sal-Cou and Ben-Cou.
Figure 1. The predicted solid–liquid equilibrium phase diagrams (full line) by COSMO-RS, the ideal liquidus diagrams (dashed line) and the experimental eutectic points at different mole fractions (▪).
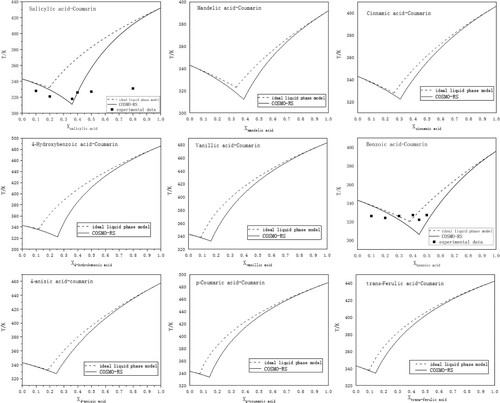
Table 1. Compounds used for the preparation of deep eutectic solvents and their melting properties.
After a batch of experimental screening, five aromatic organic acids (Cin, Ben, Hyd, Sal, Man) can be liquefied along with coumarin at the molar ratio of 1:3, and all the systems are liquids at relatively low temperatures. This is consistent with the solid–liquid phase diagram analysis. For comparing the results at the same conditions, the following characterization and quantum-chemistry calculations are conducted at the same molar ratio. The experimental melting temperatures of five systems at the molar ratio of 1:3, and at the predicted molar ratio of deep eutectic points are given in Table S1. The melting point of Sal-Cou is consistent with reported work (Citation9). The other four systems are new. DES based on cholinium chloride was studied due to its low melting enthalpy (4.3 kJ/mol), inducing the sharp melting temperature depression effect (Citation19). For the studied systems, Coua, Fer, and Van have high fusion enthalpy, but Ani has a relatively low value. Therefore, the liquid phase of eutectic system arises owing to the non-ideal behavior of all sources, which depends on the enthalpy and melting temperature of its raw materials and their intermolecular interactions. Additionally, introducing the methoxy or hydroxy group affects the charge distribution of aromatic ring that could influence the formation of eutectic systems.
FTIR analysis
FTIR spectra for Cin-Cou, Ben-Cou, Sal-Cou, Man-Cou, Hyd-Cou, and their pure sources are presented in . The characteristic bands of coumarin are observed at 1280–1625, 1210–1320, 670–900, and 950-1225 cm−1 corresponding to C = C stretching vibrations, C–O stretching vibrations, C–H bending out of the plane, and bending in plane, respectively (Citation20). The strong band arising from C = O appears in 1690 cm−1. Its shift (to 1688 cm−1 for Man, 1683 cm−1 for Cin, 1686 cm−1 for Ben, 1687 cm−1 for Sal, and 1675 cm−1 for Hyd) is obvious after preparing DESs with aromatic organic acids, illustrating that the hydrogen bonds are formed. The vibration of the alcoholic group in mandelic acid is around 3388 cm−1. The OH band shifts to 3397 cm−1 after the formation of eutectic systems, and the same trend is also founded in p-hydroxybenzoic acid (shifts to 3399 cm−1). These phenomena indicate that the interaction sites of associated complex in the two DESs are located at the alcoholic groups, which are different from other three solvent systems (Citation21).
NMR analysis
The chemical structures of starting materials and obtained NADES systems were studied by 1H NMR spectroscopy, as shown in . The signal of the H atom in the –COOH group in salicylic acid from 10.35 ppm to 10.53 ppm, demonstrating a strong interaction between the –COOH group in salicylic acid and ketone unit in coumarin. Similar observations are found for the Man-Cou, Cin-Cou, Ben-Cou, and Hyd-Cou systems, and their signals of active hydrogen shift from 4.41 to 5.82 ppm, from 8.66 to 8.80 ppm, from 9.80 to 12.11 ppm, from 3.90 to 5.50 ppm, respectively. The chemical shifts of corresponding protons (ΔδH) decrease in the order: Ben > Hyd > Man > Sal > Cin. An increase in hydrogen-bond strength removes electron density from the local vicinity of the nucleus, keeping the proton in a less shielded environment with respect to the applied magnetic field (Citation16). There is no critical change in the field shift for hydrogen in the hydroxyl or carboxyl group of pure component, indicating that the interaction site mainly locates at the carboxyl or hydroxyl group. This is consistent with the formation of 3D hydrogen-bonding network in the liquid phase of the mixture driven by groups with the greater acidity (Citation9, Citation22).
Non-bonded interaction energy
The optimized structures of NADES systems and pure constituents are shown in Figure S1-S2. SAPT was used to evaluate BE; moreover, it is capable of decomposing BE into different physical components, which facilitates deep understanding of the underlying nature of non-bonded interactions. The BE can be decomposed into four physically meaningful components: electrostatic, exchange-repulsion, induction, and dispersion interaction. (A,B) represents the values of BE and different components in the SAPT-derived energy. From these data, it is quite obvious that the sum of dispersion, induction, and electrostatic terms outweigh the exchange-repulsion part. This leads to the negative values of interaction energies in mixture systems or pure sources. Furthermore, it could be found that attractive electrostatics always plays a major role, except for the cou-cou interaction. It cannot form a hydrogen bond in its pure state, and dispersion is the main interaction due to π-π stacking. The BE values of the prepared systems increase in the order: Cin < Sal < Man < Hyd < Ben, which is consistent with the result of the NMR analysis.
Figure 4. Binding energy and its physical components calculated at SAPT2+/aug-cc-pVDZ level in the pure substances (A) and the eutectic solvent systems (B).
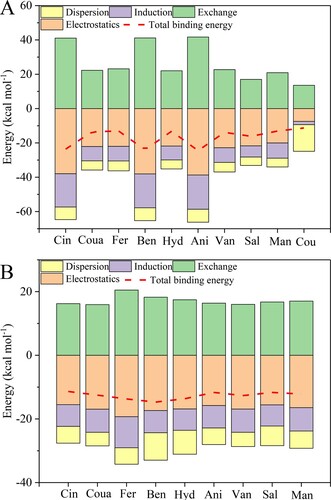
In addition, the interaction energy of HBD-HBD is almost larger than that of HBA-HBD, indicating that the molecular interactions between aromatic organic acids and coumarin in these systems are weaker than those found in the pure materials. For the obtained five eutectic systems, this is not in line with the formation rules of DES described by the reported work (Citation23): (1) For the formation of eutectic systems, the non-covalent interactions established between HBA and HBD should be much stronger than those presented in any of their pure liquid phases; (2) the hydrogen-bond interactions between the two components of DES do not guarantee the negative deviations to ideality in the solid–liquid phase diagram analysis. Actually, a set of intermolecular hydrogen bonding between HBA-HBA, HBA-HBD, and HBD-HBD is present in the DES systems. To some extent, HBD-HBD interactions are either strong or dominant in the hydrogen-bond network (Citation24). There are competitions between them (Citation25). According to this, the following equation can be proposed.
(6) where
and
are the total mean interaction energies of HBD and HBA in mixtures, respectively;
and
are total mean interaction energies in their pure sources. All total mean interaction energies were obtained by COSMO-RS.
and
have different values, so they show different probable contacts with other molecules in the system. Thus, they are the average energies of HBA-HBA, HBA-HBD, and HBD-HBD.
is the energy difference between the eutectic system and the pure substances, which demonstrates a dynamic equilibrium. Interestingly,
of the liquefying mixture is negative, otherwise positive
is unfavorable for the formation of DES (). It is worthy to mention that this equation would be used to predict the formation of eutectic solvents preliminarily. The proposed NADES offers many advantages. Although their components are potentially reactive chemicals, the auto-association by hydrogen bonds will limit their reactivity in some fields.
Table 2. Total mean interaction energy (E) obtained by COSMO-RS.
Conclusion
The melting point depression of the studied systems depends on the interactions network in the systems and melting temperatures of starting materials and their enthalpy. Furthermore, the liquid phase of the mixture arises due to the non-ideal behavior of both components at most of the ratios. Most importantly, presented the energy dynamic equilibrium of eutectic systems. Using solid–liquid phase diagrams and mean interaction energies obtained by COSMO-RS, the predicting formability of DESs can be achieved by choosing the proper HBAs and HBDs. Finally, five green solvents were obtained: Man-Cou, Sal-Cou, Ben-Cou, Hyd-Cou, and Cin-Cou. Four of them have not been reported. The aromatic organic acid studied in this work using the coumarin as the model HBA thus is vital for the development of novel NADESs.
Supplemental Material
Download MS Word (987 KB)Disclosure statement
No potential conflict of interest was reported by the author(s).
Additional information
Funding
References
- Abbott, A.P.; Capper, G.; Davies, D.L.; Rasheed, R.K.; Tambyrajah, V. Novel Solvent Properties of Choline Chloride/Urea Mixtures. Chem. Commun. 2003, 1, 70–71.
- Paiva, A.; Craveiro, R.; Aroso, I.; Martins, M. Natural Deep Eutectic Solvents - Solvents for the 21st Century. ACS Sustain. Chem. Eng. 2014, 2, 1063–1071.
- Liu, Y.; Friesen, J.B.; McAlpine, J.B.; Lankin, D.C. Natural Deep Eutectic Solvents: Properties, Applications, and Perspectives. J. Nat. Prod. 2018, 81, 679–690.
- Fernández, M.; Boiteux, J.; Espino, M.; Gomez, F.V.; Silva, M.F. Natural Deep Eutectic Solvents-Mediated Extractions: The Way Forward for Sustainable Analytical Developments. Anal. Chim. Acta 2018, 1038, 1–10.
- Smith, E.L.; Abbott, A.P.; Ryder, K.S. Deep Eutectic Solvents (DESs) and Their Applications. Chem. Rev. 2014, 114, 11060–11082.
- Tome, L.I.N.; Baiao, V.; da Silva, W.; Brett, C.M.A. Deep Eutectic Solvents for the Production and Application of New Materials. Appl. Mater. Today. 2018, 10, 30–50.
- Chen, B.L.; Peng, Z.Q.; Li, C.; Feng, Y.C. Catalytic Conversion of Biomass to Furanic Derivatives with Deep Eutectic Solvents. ChemSusChem. 2021, 14, 1496–1506.
- Clarke, C.J.; Tu, W.C.; Levers, O.; Brohl, A.; Hallett, J.P. Green and Sustainable Solvents in Chemical Processes. Chem. Rev. 2018, 118, 747–800.
- Abranches, D.O.; Martins, M.A.R.; Silva, L.P.; Schaeffer, N. Phenolic Hydrogen Bond Donors in the Formation of Non-Ionic Deep Eutectic Solvents: The Quest for Type V DES. Chem. Commun. 2019, 55, 10253–10256.
- Fan, C.; Liu, Y.; Sebbah, T.; Cao, X.L. A Theoretical Study on Terpene-Based Natural Deep Eutectic Solvent: Relationship Between Viscosity and Hydrogen-Bonding Interactions. Glob. Chall. 2021, 5, 1–8.
- Prausnitz, J.M.; Lichtenthaler, R.N.; Azevedo, E.G. Molecular Thermodynamics of Fluid-Phase Equilibria; Prentice Hall PTR: Upper Saddle River, NJ, 1999.
- Parker, T.M.; Burns, L.A.; Parrish, R.M.; Ryno, A.G.; Sherrill, C.D. Levels of Symmetry Adapted Perturbation Theory (SAPT). I. Efficiency and Performance for Interaction Energies. J. Chem. Phys. 2014, 140, 1–16.
- Parrish, R.M.; Burns, L.A.; Smith, D.G.A.; Simmonett, A.C. PSI4 1.1: An Open-Source Electronic Structure Program Emphasizing Automation, Advanced Libraries, and Interoperability. J. Chem. Theory Comput. 2017, 13, 3185–3197.
- Riley, K.E.; Hobza, P. Noncovalent Interactions in Biochemistry. Wires Comput. Mol. Sci. 2011, 1, 3–17.
- Eckert, F.; Klamt, A. Fast Solvent Screening Via Quantum Chemistry: COSMO-RS Approach. AIChE J. 2002, 48, 369–385.
- Schaeffer, N.; Abranches, D.O.; Silva, L.P.; Martins, M.A.R. Non-ideality in Thymol Plus Menthol Type v Deep Eutectic Solvents. ACS Sustain. Chem. Eng. 2021, 9, 2203–2211.
- Abranches, D.O.; Martins, R.O.; Silva, L.P.; Martins, M.A.R. Liquefying Compounds by Forming Deep Eutectic Solvents: A Case Study for Organic Acids and Alcohols. J. Phys. Chem. B 2020, 124, 4174–4184.
- Martins, M.A.R.; Silva, L.P.; Schaeffer, N.; Abranches, D.O.; Maximo, G.J.; Pinho, S.P.; Coutinho, J.A.P. Greener Terpene–Terpene Eutectic Mixtures as Hydrophobic Solvents. ACS Sustain. Chem. Eng. 2019, 7, 17414–17423.
- Omar, K.A.; Sadeghi, R. Can Isopiestic Method Predict the Formation of Deep Eutectic Solvents? J. Mol. Liq. 2021, 333, 1–9.
- Al-Kadhemy, M.F.H.; Rasheed, Z.S.; Salim, S.R. Fourier Transform Infrared Spectroscopy for Irradiation Coumarin Doped Polystyrene Polymer Films by Alpha ray. J. Radiat. Res. Appl. Sci. 2019, 9, 321–331.
- Babij, M.; Mondry, A. Synthesis, Structure and Spectroscopic Studies of Europium Complex with S(+)-Mandelic Acid. J. Rare Earth 2011, 29, 1188–1191.
- Fan, C.; Sebbah, T.; Liu, Y.; Cao, X. Terpenoid-Capric Acid Based Natural Deep Eutectic Solvent: Insight Into the Nature of Low Viscosity. Cleaner Eng. Technol. 2021, 3, 1–9.
- Abranches, D.O.; Silva, L.P.; Martins, M.A.R.; Pinho, S.P.; Coutinho, J.A.P. Understanding the Formation of Deep Eutectic Solvents: Betaine as a Universal Hydrogen Bond Acceptor. ChemSusChem. 2020, 13, 4916–4921.
- Turner, A.H.; Holbrey, J.D. Investigation of Glycerol Hydrogen-Bonding Networks in Choline Chloride/Glycerol Eutectic-Forming Liquids Using Neutron Diffraction. Phys. Chem. Chem. Phys. 2019, 21, 21782–21789.
- Malik, A.; Kashyap, H.K. Heterogeneity in Hydrophobic Deep Eutectic Solvents: SAXS Prepeak and Local Environments. Phys. Chem. Chem. Phys. 2021, 23, 3915–3924.