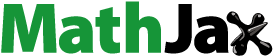
ABSTRACT
Gelatin is a famous gel-forming proteinaceous material with excellent hydrogelation properties. Herein, we report that protein nanofibrils (PNFs) can be employed to form hybrids with gelatin that can be converted to aerogels with attractive mechanical properties. Moreover, we are able to influence the gelation behavior of PNFs by mechanochemical processing. A combination of mechanochemistry and aqueous self-assembly is employed to prepare PNFs functionalized with hydrophobic dyes. These materials are then mixed with gelatin and converted into aerogels by freeze drying. We find that PNFs functionalized with PNFs lead to the formation of aerogels with more robust mechanical properties. Mechanochemical methodology as a green and scalable method can thus be employed for influencing the properties of protein-based aerogels. This represents a new and highly flexible and novel strategy for tuning both properties and functionality of protein materials. This work opens a simple and feasible way to produce nontoxic and biodegradable aerogel materials with favorable mechanical strength.
GRAPHICAL ABSTRACT
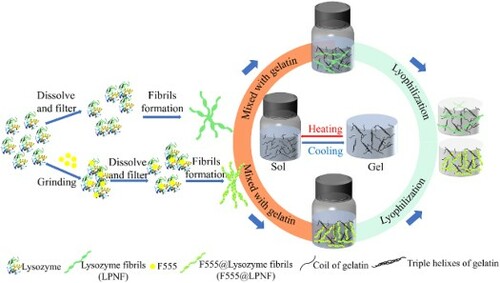
1. Introduction
An important research topic is the replacement of materials derived from petroleum with materials obtainable from renewable sources (Citation1). Many proteins or protein-derived materials are formed as side products from industrial processes and are hence potential candidates for renewable raw materials (Citation2–5). Proteins are extremely complex and have a rich supramolecular chemistry providing interesting opportunities when fabricating materials. Examples of such valuable characteristics employed in the present study are the ability of protein to self-assemble into fibrils and tendencies of proteins or protein-derived materials to form gels. Gelatin is a low cost and widespread proteinaceous material—famous for its gel-forming capacity—which is derived from skin, tendons, and bone. Gelatin has been widely used in the pharmaceutical, food, cosmetic, and photographic industries because of its biodegradability, biocompatibility, and non-immunogenicity (Citation6–9). Gelatin can easily dissolve in water and form a physical thermally reversible hydrogel at low temperature. However, the preparation of dry gelatin-based materials is challenging due to the brittleness of dry gelatin (Citation10). This is unfortunate as hydrogels are generally considered as attractive precursors for aerogels (Citation11–13). A common way to convert a hydrogel into an aerogel involves freeze drying (Citation14). The sample is first frozen and water is then removed at reduced pressure. During the freezing step, ice formation will lead to deformations of the gel network, which can present a problem, but also an opportunity for tuning of aerogel structure and porosity. The unique properties of aerogels include ultra-low-density, low optical index of refraction, low thermal conductivity, and low propagation speed of sound, and aerogels are investigated for a range of different applications, including as materials for energy storage and conversion (Citation15–17), sensors (Citation18, Citation19), catalyst supports (Citation20, Citation21), and environmental remediation materials (Citation22, Citation23). Aerogels have been formed from a wide range of materials including Kevlar (Citation24, Citation25) and bio-based materials (Citation26–28).
Many of the developed aerogels employ polymers ultimately derived from petroleum (Citation13). In recent years, there has been an increased interest in developing aerogels derived from biopolymers such as proteins or polysaccharides. However, the resulting aerogels will often be brittle, and it is hence important to develop methodology that can influence the mechanical properties of such materials. There are few reports presenting mechanically flexible gelatin-based aerogels (Citation29). It was demonstrated that chemical vapor deposition treatment with silanes resulted in flexible aerogels (Citation9, Citation30). Moreover, Ti3C2Tx MXene/ gelatin nanocomposite aerogel formed for electromagnetic absorption (Citation31). In addition, aerogels which gelatin cross-linked with polyvinyl alcohol to enhance the properties of compression and flame retardancy (Citation32). An interesting type of material for modification of the properties of gelatin aerogels is protein nanofibrils (PNFs) (Citation33–35). PNFs can be formed by self-assembly in vitro from a wide range of proteins. PNFs are high aspect ratio particles with a typical diameter of 5–10 nm and lengths in the μm range. PNFs are related to amyloid fibrils—a type of structure formed in vivo that can have both detrimental and beneficial effects on the organism (Citation36, Citation37). PNFs have been investigated regarding applications in different areas and applications, including LEDs (Citation38, Citation39), sensors (Citation40–42), and drug delivery (Citation43, Citation44). A recent example of the use of functional amyloid in materials science is the demonstration that genetically modified Escherichia coli (E. coli.) bacteria produced curli (a functional amyloid) containing biofilms that could be processed into bioplastics (Citation45). PNFs have also been converted into various types of aerogels (Citation46).
As PNFs are built up by self-assembly their properties can be influenced by modifying the protein building block. There are many different methods for modification of proteins, for example being based on synthetic biology or click chemistry (Citation47, Citation48). From a sustainability perspective methodology based on mechanochemistry, (Citation49) should be considered. We have developed an alternative methodology for protein functionalization/valorization based on mechanochemistry. By co-grinding of a protein, capable of self-assembly into PNFs, with hydrophobic dyes, a water-soluble hybrid material is formed. Due to the hydrophobic effect the dye will stay associated with the protein during PNF formation, the end result being an aqueous dispersion of PNFs functionalized with the hydrophobic dye (Citation38, Citation39, Citation43, Citation50–Citation55). We have reported that such functionalized PNFs have an increased tendency to aggregate, for example by forming films at the air–water interface or forming abnormally large protein-spherulites (Citation52, Citation56). The functionalization PNFs by hydrophobic dyes accordingly presents a convenient way to modify the colloidal properties of PNF dispersions. The methodology thus presents opportunities to modify the properties of protein materials in a cost effective and green way—mill the protein with a hydrophobic molecule/material and then expose the protein to conditions that promote self-assembly of the protein into PNFs. The presence of the hydrophobic molecule/material may then influence the properties of the resulting macroscopic materials. Mechanochemical methodology can accordingly be employed to tune and valorize various renewable protein materials—the hydrophobic molecule/material may modify the physical properties of a protein while simultaneously valorizing the material by providing extra function. Herein we investigate if this methodology can be employed to influence the properties of gelatin: PNF hydrogels and aerogels.
As a model protein, we employ hen egg white lysozyme (HEWL). As hydrophobic dye component, we investigate the luminescent dyes (2-butyl-6-(butylamino)-1H-benz[de]isoquinoline-1,3(2H)-dione, abbreviated as F555); Fluorescent brighter 378 (F378); and 4-(dicyanomethylene)-2-methyl-6-(4-dimethylaminostyryl)-4H-pyran (DCM). The functionalized lysozyme protein nanofibrils (LPNFs) are labeled as F555@LPNF, F378@LPNF and DCM@LPNF. Of these different materials, the F555@LPNF material gives aerogels with the most attractive mechanical properties and our study therefore focus on the properties of the F555@LPNF system; however, for comparison, we also provide selected information for the gels derived from the F378@LPNF and DCM@LPNF systems. A schematic illustration of the materials preparation is shown in using F555 as an example. Briefly, F555@LPNF and gelatin dispersions/solutions are mixed by stirring while heated. The resulting mixture is allowed to cool which results in the formation of a hydrogel. Next, the hydrogels are converted into aerogels by a freeze-drying process. We find that the presence of hydrophobic dyes results in improved mechanical properties of the resulting aerogels. The fabricated F555@LPNF gel-based fluorescent aerogel retains the typical features such as the amyloid fibrils-based aerogels with lightweight (about 0.02 g·cm–3), high porosity (>93%), and specific surface area (>20 m2 g–1), and also exhibits the additional characteristic of shape recoverability.
2. Materials and methods
2.1. Materials
(2-butyl-6-(butylamino)-1H-benzo[de]isoquinoline-1,3(2H)-dione) (Fluorol 555, F555) was obtained from Exciton (Dayton, OH). 4-(dicyanomethylene)-2-methyl-6-(4-dimethylaminostyryl)-4H-pyran (DCM) and Fluorescent brighter 378 were obtained from TCl. HEWL and HCl (1M) were purchased from Sigma-Aldrich. Gelatin (GEL, Sinopharm Chemical Reagent Co., Ltd). All chemicals were used as received without further purification, and deionized water (18.2 Ω) was used throughout.
2.2. Preparation of functionalized LPNFs and LPNFs
The dye (one of F555, F378, or DCM) (1 mg) and HEWL (100 mg) were ground with ceramic mortar and pestle for 10 min. The resulting mixture was dissolved in 5 mL 25 mM hydrochloric acid followed by filtration through a 0.45 μm Polyethersulfone filter. The resulting dispersion was heated at 80°C with magnetic stirring at 1000 rpm for 24 h to obtain F555@Lysozyme protein fibrils (F555@LPNF). For comparison, the pure HEWL (100 mg) without F555 was ground for 10 min and treated by the procedure described above in order to obtain lysozyme fibrils (2% LPNF, w/v).
2.3. AFM characterization of LPNFs and functionalized LPNFs
Atomic force microscopy (AFM) measurements were obtained using a digital instruments dimension 3100 atomic force microscope. The initial reaction mixtures (LPNF, F555@LPNF, F378@LPNF, and DCM@LPNF) were diluted 100 times prior to applying to clean silica substrates (washed with TL-1 solution at 120°C for 20 min, Ammonia:H2O2:H2O = 1:1:5) and left to dry for 1 min. Excess fluid was removed by applying a nitrogen gas flow. The length distribution of PNFs was determined with the help of ImageJ software by counting 100 PNFs that were plotted as a histogram.
2.4. Preparation of LPNF:GEL hydrogels and aerogels
Solutions of gelatin (GEL) in water (2% w/v) was prepared by dissolving 0.4 g gelatin in 20 ml deionized water, and the mixture was stirred for 1 h at 60°C until the gelatin was completely dissolved. LPNF:GEL hydrogels named as LPNF:Gel-x (x represents the mass ratio of LPNF to gelatin, x = 2, 1.5, 1, 0.5, corresponding to ratios of 2:1, 1.5:1, 1:1, 0.5:1, respectively) were obtained by mixing the 2% LPNF solution and 2% gelatin solution in different ratios (while ensuring that the total amount of protein equaled 40 mg), then the mixture was stirred for 3 min at 60°C. The resulting mixture was maintained at 4°C for at least 2 h, forming emulsion-gels. F555@LPNF:GEL-x hydrogels were also prepared by mixing F555@LPNF dispersions and gelatin solutions in different ratios.
The resulting hydrogels (both LPNF:Gel and F555@LPNF:Gel) were placed on a steel plate containing with liquid nitrogen to directly freeze the hydrogels and were then dried under reduced pressure for 48 h to obtain the aerogels.
2.5. Rheology of LPNF:GEL hydrogels
Rheological tests were performed using a TA HR-2 rheometer equipped with 20 mm parallel stainless-steel plates. Rheological frequency sweeps from 0.1 to 100 Hz were performed with a shear strain of 1% in the parallel plate geometry at oscillatory rheology measurements. Rheological amplitude sweeps from 0.1% to 10,000% were assessed with a fixed oscillatory frequency of 1 Hz. All samples were run in at least duplicates.
2.6. Porosity, specific surface area, and microstructure of LPNF:GEL aerogels
The porosity of the obtained aerogels was calculated according to the following equation
(1)
(1) ρaerogel and ρs represent the apparent density and skeletal density, respectively, of the aerogel, which can be calculated by the following equations
(2)
(2)
(3)
(3) where m and V are the weight and volume, respectively, of the fluorescent aerogel. ρP represents the density of protein which was assumed to be 1.35 g cm–3 (Citation57).
The specific surface area of aerogels was measured at the relative pressure of P/Po = 0.995 by the Brunauer–Emmett–Teller (BET) method on a specific surface and pore analyzer (Micromeritics ASAP 2020). The nitrogen isothermal adsorption and desorption volumes were measured at −196°C with samples outgassed at 180°C for 5 h. The pore size distribution was calculated from the adsorption isotherm using the Barrett–Joyner–Halenda method (Citation58).
The micro-structures of the aerogels were imaged by scanning electron microscopy (SEM, Zeiss Sigma 300 VP, Germany) at an accelerating voltage of 2 kV, and the aerogels were cut with a scissor in the horizontal and vertical direction. After that the aerogels were sputter coated with Pt for 10 s at high vacuum (10−5 Pa), in order to avoid charge-up effects during scanning electron microscope (SEM) imaging.
2.7. Young’s modulus of LPNF:GEL aerogels
The characterization of Young’s modulus of LPNF matrix aerogels was performed by adding different weight on the aerogels and observing the deformation. The stress was calculated by the equation:
where F is the applied weight on the aerogel and A is the contact area. The deformation of the aerogel at a given stress was measured and the strain calculated. The Young’s modulus was then obtained from the slope of a plot of stress against strain.
2.8. Thermal stability of LPNF:GEL aerogels
A thermogravimetric analysis (TGA) instrument (Mettler Toledo) was used to evaluate the thermal properties of aerogel. The measurements were performed in the range of 30–900°C with a heating rate of 10°C/min in 900 μL alumina crucibles under nitrogen gas flow (N2 flow rate is 25 mL min−1). Before starting the measurements, the aerogel was kept at 100°C for 30 min to eliminate adsorbed water. Furthermore, to estimate the residue content of the aerogel, it was maintained at 900°C at isothermal conditions for 30 min.
3. Results and discussion
3.1. Morphology of LPNFs
In order to characterize the length distribution of functionalized PNFs and normal PNFs (without hydrophobic dye), samples were prepared in an identical way (heating at 80°C with magnetic stirring at 1000 rpm for 24 h). Samples were then drop-casted onto substrates and AFM micrographs were recorded. The morphology of unmodified LPNFs as well as LPNFs functionalized with dyes (F555@LPNF, F378@LPNF, and DCM@LPNF) was investigated by AFM. As shown in , the LPNF and F555@LPNF samples both have PNFs with lengths varying between 0.5 and 4 μm. The most common length of LPNF is about 1 μm, whereas the F555@LPNF sample shows a distribution shifted towards longer lengths, with the most common length being about 1.5 μm, meaning that F555 functionalized fibrils tend to be longer than pure LPNFs. The length distributions of F378@LPNF and DCM@LPNF is more similar to that of the LPNF sample, with the most common length being about 1 μm (Figure S1, supporting information).
3.2. Initial tests of gelation and aerogel properties
To determine a suitable ratio between LPNF (and dye@LPNF) and gelatin, the two components were mixed at different ratios and the sol-to-gel transition of LPNF:GEL was examined by the test tube inversion method ((a,b)). Note that for the samples in (a,b), the total amount of protein is always 40 mg, and the x:y ratio indicates the LPNF:GEL ratio. The mixtures employing unmodified LPNFs tend to form a gel when the LPNF:GEL ratio of is close to 1:1. On the other hand, for the samples functionalized with hydrophobic dyes, they tend to form gels also at a higher fibril to gelatin ratio. F555@LPNF samples form gels at the F555@LPNF:GEL ratio of 1.5:1. However, during preliminary tests of the properties of the aerogels obtainable from the different compositions (see below for further discussion of aerogel formation and structure), it was found that a too high PNF ratio led to brittle samples. Therefore, the LPNF:GEL ratio of 0.5:1 (abbreviated as LPNF:GEL-0.5) was chosen for investigations involving aerogels ((c)). In preliminary tests of the mechanical properties of the resulting aerogels, a dramatic difference was found between aerogels made from LPNFs and aerogels made from LPNFs functionalized with hydrophobic dyes. All aerogels can withstand modest deformations but if the LPNF:GEL-0.5 aerogel is compressed to about 50% of its original length cracks start to form, and if compressed to about 75% the sample shatters ((e)). If the F555@LPNF:GEL-0.5, F378@LPNF:GEL-0.5 and DCM@LPNF:GEL-0.5 are compressed to 50% of their original length and the force is removed, the aerogels will revert to their original shape ((d)). However, if compressed above about 75%, they will undergo a permanent deformation, but without breakage ((e)).
Figure 3. (a–b) Photographs of samples of LPNF (or F555@LPNF) and gelatin mixed in different ratios. (c) Photographs of aerogels formed from LPNF:GEL-0.5 and F555@LPNF:GEL-0.5. (d) Compression behavior of the F555@LPNF:GEL-0.5 aerogel. (e) Deformation behavior of LPNF:GEL-0.5 and dyes@LPNF:GEL-0.5 aerogels.
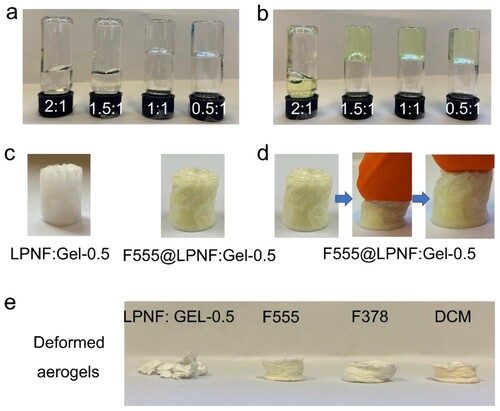
3.3. Rheological characterization of LPNF:GEL hydrogels
Rheological measurements were performed in order to evaluate the mechanical properties of the LPNF:gelatin gels and the F555@LPNF:gelatin gels. Frequency sweep tests are widely used to obtain information about the stability of the gel networks and to verify if their structures correspond to the rheological definition of a gel. The storage (G′) and loss modulus (G″) of a viscoelastic material, representing the deformation energy stored and dissipated during the shear process, respectively, were measured. Frequency sweep, measurements were carried out at different oscillation frequencies with a constant oscillation amplitude (1%) and temperature (25°C). Within the angular frequency of 0.1–100 rad s−1, the value of the storage modulus (G′) is greater than the loss modulus (G″) for both the F555@LPNF and LPNF hydrogel, indicating the formation of an elastic solid-like hydrogel (). Interestingly, the stiffness of F555@LPNF hydrogel was about 10 times higher than that of pure LPNF hydrogel at the ratio of 2:1, and four times and two times higher for the 1.5:1 and 1:1 ratio separately. For the 0.5:1 ratio sample, the difference is small with a slightly higher stiffness for the F555@LPNF sample. PNFs can accordingly be employed to influence the mechanical properties of the hydrogels.
Figure 4. Rheological measurements of dynamic frequency sweep of the LPNF:GEL (a) and F555@LPNF:GEL (b) co-assembled gels at a strain of 1% over a range of 0.1–100 Hz.
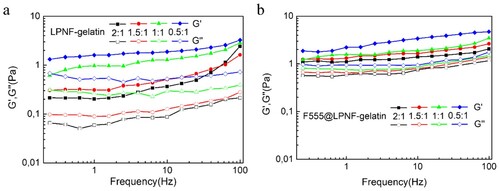
In addition, strain sweep experiments were performed to determine the linear viscoelastic regime of the hydrogels (). The breakage strain of the LPNF:GEL hydrogels increased from 235% to 553% by decreasing the ratio of LPNF to gelatin from 2:1 to 0.5:1. Interestingly, for the F555@LPNF:GEL hydrogels, the breakage strain increased from 29% to 221% when the PNF:GEL ratio was decreased from 2:1 to 0.5:1. The breakage strain of the F555@LPNF:GEL hydrogels exhibited lower value compared to the LPNF:GEL samples indicating F555 doping increased the mechanical properties of the hydrogels. This behavior may indicate that the F555@LPNF:GEL co-assembled structures are likely more rigid and hence unable to withstand higher strain values. The difference in modulus values indicated different networks of micro/nanostructures inside the gels, potentially influencing their mechanical properties (Citation59).
3.4. Porosity, specific surface area, and microstructure of LPNF:GEL aerogels
In the description below, the composite aerogels are coded as LPNF:GEL-x (where x is the mass ratio between LPNF (or dye@LPNF) and gelatin—the sample with a LPNF:GEL ratio of 0.5:1 is accordingly labeled as LPNF:GEL-0.5) and key parameters are summarized in . In order to convert the hydrogels into aerogels, we employed freeze drying. The samples were first frozen by immersing the sample liquid nitrogen, and water was then removed under reduced pressure, resulting in free standing aerogels. In is shown the SEM images of aerogels formed from gelatin, LPNF:GEL-0.5 as well as F555@LPNF:GEL-0.5 samples. The gelatin aerogel is built up from large flake-like structures ((a)). In the LPNF:GEL-0.5 sample there is still a flake-like morphology but with some porosity. The morphology of the F555@LPNF:GEL-0.5 is dramatically different with a relatively uniform and compact network with highly porous structures ((c)). SEM images of the F378@LPNF:GEL-0.5 and DCM@LPNF:GEL-0.5 samples are shown in the supporting information (Figure S2), and in both cases, a flake-like morphology is observed but with much smaller dimensions than for the LPNF:GEL-0.5 sample.
Figure 6. SEM images of aerogels. (a–c) Top-view of gelatin (a) LPNF:GEL-0.5 (b) F555@LPNF:GEL-0.5 (c) aerogels. (d–f) Cross-section of gelatin (d) LPNF:GEL-0.5 (e) F555@LPNF:GEL-0.5 (f) aerogels, scale bar is 10 μm.
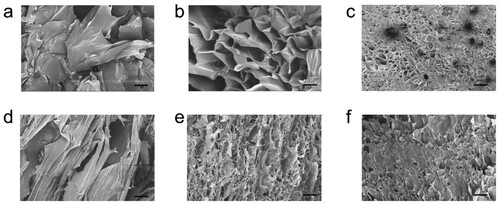
Table 1. Comparative results aerogels.
The density of aerogels with a different amount of LPNF is shown in (a). The densities are 0.022 g cm−3 for the gelatin aerogel and 0.023 g cm−3 for LPNF:GEL-0.5 and 0.024, 0.025, and 0.026 g cm−3 for F378@LPNF:GEL-0.5, F555@LPNF:GEL-0.5, and DCM @LPNF:GEL-0.5 aerogels, respectively. The density of the aerogels incorporating various types and amounts of LPNF display only slight variations. The reason for the lower densities of gels incorporating LPNF is due to smaller volume shrinkage than for the GEL sample. The aerogels incorporating LPNF have a high porosity—up to 93% for the LPNF:GEL-0.5 and F555@LPNF:GEL0.5. In (b) is shown photos of the different aerogels under ambient light and under UV-light (365 nm), demonstrating the gels have fluorescent properties.
Figure 7. (a) Density of the aerogels with the different ratio of LPNF or Dyes@LPNF (F555, F378, DCM) and gelatin. (b) Photos of aerogels under UV light (365 nm) and ambient light samples. (c) Nitrogen gas absorption–desorption curve of gelatin and LPNF matrix aerogels. (d) TGA curves of amyloid fibril aerogel under nitrogen atmospheres. The residue obtained after the complete decomposition is remaining salts in the system.
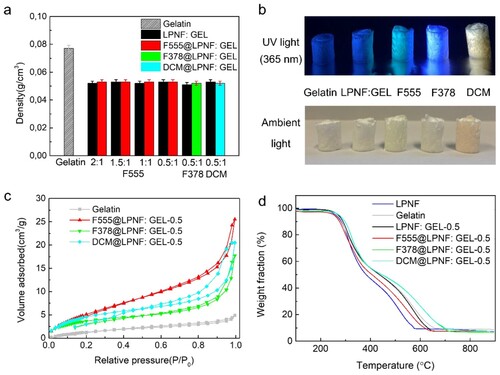
The surface area and pore volume of the aerogels were investigated by N2 adsorption−desorption isotherm analysis by using the BET method. As shown in (c), the gelatin aerogel had a specific surface area of 6.7 m2 g−1, and for LPNF functionalized with F555 (F555@LPNF), it increased to 22.36 m2 g−1. Furthermore, the specific surface area of F378@LPNF:GEL-0.5 and DCM@LPNF:GEL-0.5 aerogels were 14.59 and 18.37 m2 g−1, respectively. The average pore diameters of gelatin, F378@LPNF:GEL-0.5, DCM@LPNF:GEL-0.5, and F555@LPNF: GEL-0.5 aerogels were 377, 336, 312, and 349 nm, respectively.
The thermal stability of the aerogels was investigated by TGA. As show in (d), pristine gelatin and gelatin with protein matrix aerogels gave <10% weight loss at the beginning up to 100°C which can be attributed to loss of water. The LPNF:GEL-0.5 aerogel and F555@LPNF:Gel-0.5 aerogel started to lose mass at about 270°C, indicating a high thermal stability. The onset temperature (Tonset) and peak temperature (Tp) of different materials are listed in (DTG curves were shown in supporting information, Figure S3). We further investigated the thermal stability by storing the different samples at 200°C under air atmosphere for 24 h (See supporting information in Figure S4). This leads to an obvious discoloration but the aerogels containing LPNFs are able to retain their shape, showing a superior stability to temperature-induced deformation than many commercial-grade petroleum-based thermoplastic foams that collapsed/melted after being subjected to a temperature of 150°C (Citation60).
The pure gelatin aerogel is hard and we were not able to determine the Young’s modulus. The LPNF:GEL-0.5 aerogel has a Young’s modulus of 3.27 kPa. For aerogels obtained by mixing gelatin with LPNFs functionalized with the different dyes (F555, F378 and DCM), the Young’s modulus were 2.94 kPa (F555@LPNF:GEL-0.5), 3.53 kPa (F378@LPNF:GEL-0.5), and 3.28 kPa (DCM@LPNF:GEL-0.5) The Young’s modulus, pore size and specific surface area as well as density for the different aerogels are summarized in .
LPNFs accordingly present an interesting material for tuning the properties of gelatin-derived aerogels. The mechanochemical processing provides an additional parameter to tune the properties of the aerogels. For the systems examined herein, the employment of different dye@LPNF materials allows the formation of mechanically more robust aerogels that in contrast to aerogels incorporating LPNF (without dyes) do not undergo fracturing at large deformations.
4. Conclusion
Herein, we have developed an all protein-based aerogel with favorable mechanical properties in a green and sustainable method by mechanochemistry. The properties of the aerogels can be adjusted by varying the relative ratio of PNFs and gelatin. The properties of PNFs can in turn be influenced by mechanochemical functionalization with hydrophobic dyes (dye@PNF). Aerogels prepared from gelatin and dye@PNF materials have better mechanical properties than aerogels prepared from gelatin and unmodified PNFs. These protein-based aerogels are prepared from edible components and may therefore find applications related to e.g. food science. In addition, as the aerogels are functionalized with luminescent molecules, they may find applications as e.g. sensors or photonic materials.
Supplemental Material
Download MS Word (1.4 MB)Acknowledgements
We acknowledge Daniel Aili and the Laboratory of Molecular Materials at IFM, LiU for access to their rheometer.
Disclosure statement
No potential conflict of interest was reported by the author(s).
Additional information
Funding
References
- Tang, X.Z.; Kumar, P.; Alavi, S.; Sandeep, K.P. Recent Advances in Biopolymers and Biopolymer-based Nanocomposites for Food Packaging Materials. Crit. Rev. Food Sci. 2012, 52, 426–442.
- Gautam, S.; Sharma, B.; Jain, P. Green Natural Protein Isolate Based Composites and Nanocomposites: A Review. Polym. Test 2021, 99, 106626.
- Jafarzadeh, S.; Forough, M.; Amjadi, S.; Javan Kouzegaran, V.; Almasi, H.; Garavand, F.; Zargar, M. Plant Protein-Based Nanocomposite Films: A Review on the Used Nanomaterials, Characteristics, and Food Packaging Applications. Crit. Rev. Food Sci. 2022. doi:10.1080/10408398.2022.2070721
- Mihalca, V.; Kerezsi, A.D.; Weber, A.; Gruber-Traub, C.; Schmucker, J.; Vodnar, D.C.; Dulf, F.V.; Socaci, S.A.; Fărcaș, A.; Mureșan, C.I.; Suharoschi, R.; Pop, O.L. Protein-based Films and Coatings for Food Industry Applications. Polymers (Basel) 2021, 13, 769.
- Giaveri, S.; Schmitt, A.M.; Roset Julia, L.; Scamarcio, V.; Murello, A.; Cheng, S.; Menin, L.; Ortiz, D.; Patiny, L.; Bolisetty, S. et al. Nature-inspired Circular-economy Recycling for Proteins: Proof of Concept. Adv. Mater. 2021, 33, 2104581.
- Douglas, S.J.; Davis, S.S.; Illum, L. Nanoparticles in Drug Delivery. Crit. Rev. Ther. Drug Carrier Syst. 1987, 3, 233–261.
- Kim, H.W.; Kim, H.E.; Salih, V. Stimulation of Osteoblast Responses to Biomimetic Nanocomposites of Gelatin-Hydroxyapatite for Tissue Engineering Scaffolds. Biomaterials 2005, 26, 5221–5230.
- Liu, Y.; Chan-Park, M.B. Hydrogel Based on Interpenetrating Polymer Networks of Dextran and Gelatin for Vascular Tissue Engineering. Biomaterials 2009, 30, 196–207.
- Wang, J.; Zhao, D.; Shang, K.; Wang, Y.T.; Ye, D.D.; Kang, A.H.; Liao, W.; Wang, Y.Z. Ultrasoft Gelatin Aerogels for Oil Contaminant Removal. J. Mater. Chem. A. 2016, 4, 9381–9389.
- Kozlov, P.V.; Burdygina, G.I. The Structure and Properties of Solid Gelatin and the Principles of Their Modification. Polymer 1983, 24, 651–666.
- Zhao, S.; Malfait, W.J.; Guerrero-Alburquerque, N.; Koebel, M.M.; Nyström, G. Biopolymer Aerogels and Foams: Chemistry, Properties, and Applications. Angew. Chem. Int. Ed. 2018, 57, 7580–7608.
- Qian, Z.; Wang, Z.; Zhao, N.; Xu, J. Aerogels Derived from Polymer Nanofibers and Their Applications. Macromol. Rapid Commun. 2018, 39, 1700724.
- Wei, G.; Zhang, J.; Usuelli, M.; Zhang, X.; Liu, B.; Mezzenga, R. Biomass vs Inorganic and Plastic-Based Aerogels: Structural Design: Functional Tailoring, Resource-Efficient Applications and Sustainability Analysis. Prog. Mater. Sci. 2022, 125, 100915.
- Nyström, G.; Fong, W.K.; Mezzenga, R. Ice-Templated and Cross-Linked Amyloid Fibril Aerogel Scaffolds for Cell Growth. Biomacromolecules 2017, 18, 2858–2865.
- Wang, S.; Wang, R.; Zhao, Q.; Ren, L.; Wen, J.; Chang, J.; Fang, X.; Hu, N.; Xu, C. Freeze-Drying Induced Self-Assembly Approach for Scalable Constructing MoS2/Graphene Hybrid Aerogels for Lithium-Ion Batteries. J. Colloid Interface Sci. 2019, 544, 37–45.
- Xiong, C.; Zou, Y.; Peng, Z.; Zhong, W. Synthesis of Morphology-Tunable Electroactive Biomass/Graphene Composites Using Metal Ions for Supercapacitors. Nanoscale 2019, 11, 7304–7316.
- Wang, D.C.; Yu, H.Y.; Qi, D.; Ramasamy, M.; Yao, J.; Tang, F.; Tam, K.M.C.; Ni, Q. Supramolecular Self-Assembly of 3D Conductive Cellulose Nanofiber Aerogels for Flexible Supercapacitors and Ultrasensitive Sensors. ACS Appl. Mater. Interfaces 2019, 11, 24435–24446.
- Xiao, Z.; Zhou, W.; Zhang, N.; Zhang, Q.; Xia, X.; Gu, X.; Wang, Y.; Xie, S. All-Carbon Pressure Sensors with High Performance and Excellent Chemical Resistance. Small 2019, 15, 1804779.
- Yang, L.; Liu, Y.; Filipe, C.D.; Ljubic, D.; Luo, Y.; Zhu, H.; Yan, J.; Zhu, S. Development of a Highly Sensitive, Broad-Range Hierarchically Structured Reduced Graphene Oxide/PolyHIPE Foam for Pressure Sensing. ACS Appl. Mater. Interfaces 2019, 11, 4318–4327.
- Qiu, J.; Fan, P.; Yue, C.; Liu, F.; Li, A. Multi-networked Nanofibrous Aerogel Supported by Heterojunction Photocatalysts with Excellent Dispersion and Stability for Photocatalysis. J. Mater. Chem. A. 2019, 7, 7053–7064.
- Wu, Z.S.; Yang, S.; Sun, Y.; Parvez, K.; Feng, X.; Müllen, K. 3D Nitrogen-Doped Graphene Aerogel-Supported Fe3O4 Nanoparticles as Efficient Electrocatalysts for the Oxygen Reduction Reaction. J. Am. Chem. Soc. 2012, 134, 9082–9085.
- Feng, Z.Q.; Wu, F.; Jin, L.; Wang, T.; Dong, W.; Zheng, J. Graphene Nanofibrous Foam Designed as an Efficient Oil Absorbent. Ind. Eng. Chem. Res. 2019, 58, 3000–3008.
- Lin, Y.Z.; Zhong, L.B.; Dou, S.; Shao, Z.D.; Liu, Q.; Zheng, Y.M. Facile Synthesis of Electrospun Carbon Nanofiber/Graphene Oxide Composite Aerogels for High Efficiency Oils Absorption. Environ. Int. 2019, 128, 37–45.
- Liu, Z.; Lyu, J.; Fang, D.; Zhang, X. Nanofibrous Kevlar Aerogel Threads for Thermal Insulation in Harsh Environments. ACS Nano. 2019, 13, 5703–5711.
- Cheng, B.; Wu, P. Scalable Fabrication of Kevlar/Ti3C2Tx MXene Intelligent Wearable Fabrics with Multiple Sensory Capabilities. ACS Nano. 2021, 15, 8676–8685.
- Si, Y.; Wang, X.; Dou, L.; Yu, J.; Ding, B. Ultralight and Fire-Resistant Ceramic Nanofibrous Aerogels with Temperature-Invariant Superelasticity. Sci. Adv. 2018, 4, 8925.
- Song, J.; Chen, C.; Yang, Z.; Kuang, Y.; Li, T.; Li, Y.; Huang, H.; Kierzewski, I.; Liu, B.; He, S. et al. Highly Compressible, Anisotropic Aerogel with Aligned Cellulose Nanofibers. ACS Nano. 2018, 12, 140–147.
- Li, Y.Q.; Samad, Y.A.; Polychronopoulou, K.; Liao, K. Lightweight and Highly Conductive Aerogel-Like Carbon from Sugarcane with Superior Mechanical and EMI Shielding Properties. ACS Sustainable Chem. Eng. 2015, 3, 1419–1427.
- Rigueto, C.V.T.; Nazari, M.T.; Massuda, L.Á.; Ostwald, B.E.P.; Piccin, J.S.; Dettmer, A. Production and Environmental Applications of Gelatin-Based Composite Adsorbents for Contaminants Removal: A Review. Environ. Chem. Lett. 2021, 19, 2465–2486.
- Li, S.L.; Wang, J.; Zhao, H.B.; Cheng, J.B.; Zhang, A.N.; Wang, T.; Cao, M.; Fu, T.; Wang, Y.Z. Ultralight Biomass Aerogels with Multifunctionality and Superelasticity Under Extreme Conditions. ACS Appl. Mater. Interfaces 2021, 13, 59231–59242.
- Yang, M.; Yuan, Y.; Li, Y.; Sun, X.; Wang, S.; Liang, L.; Ning, Y.; Li, J.; Yin, W.; Li, Y. Anisotropic Electromagnetic Absorption of Aligned Ti3C2Tx MXene/Gelatin Nanocomposite Aerogels. ACS Appl. Mater. Interfaces 2020, 12, 33128–33138.
- Wang, Y.T.; Zhao, H.B.; Degracia, K.; Han, L.X.; Sun, H.; Sun, M.; Wang, Y.Z.; Schiraldi, D.A. Green Approach to Improving the Strength and Flame Retardancy of Poly(Vinyl Alcohol)/Clay Aerogels: Incorporating Biobased Gelatin. ACS Appl. Mater. Interfaces 2017, 9, 42258–42265.
- Lendel, C.; Solin, N. Protein Nanofibrils and Their Use as Building Blocks of Sustainable Materials. RSC Adv. 2021, 11, 39188–39215.
- Knowles, T.P.; Mezzenga, R. Amyloid Fibrils as Building Blocks for Natural and Artificial Functional Materials. Adv. Mater. 2016, 28, 6546–6561.
- Ke, P.C.; Zhou, R.; Serpell, L.C.; Riek, R.; Knowles, T.P.; Lashuel, H.A.; Gazit, E.; Hamley, I.W.; Davis, T.P.; Fändrich, M. et al. Half a Century of Amyloids: Past, Present and Future. Chem. Soc. Rev. 2020, 49, 5473–5509.
- Knowles, T.P.; Vendruscolo, M.; Dobson, C.M. The Amyloid State and Its Association with Protein Misfolding Diseases. Nat. Rev. Mol. Cell Bio. 2014, 15, 384–396.
- Chiti, F.; Dobson, C.M. Protein Misfolding, Functional Amyloid, and Human Disease. Annu. Rev. Biochem. 2006, 75, 333–366.
- Rizzo, A.; Solin, N.; Lindgren, L.J.; Andersson, M.R.; Inganas, O. White Light with Phosphorescent Protein Fibrils in OLEDs. Nano Lett. 2010, 10, 2225–2230.
- Yuan, Y.; Solin, N. Mechanochemical Preparation and Self-Assembly of Protein: Dye Hybrids for White Luminescence. ACS Appl. Poly. Mater. 2021, 3, 4825–4836.
- Hu, J.; Wang, L.; Zhang, X.; Yu, W.; Gao, H.W.; Solin, N.; Hu, Z.; Uvdal, K. Selective Colorimetric Detection of Copper (II) by a Protein-Based Nanoprobe. Spectrochim. Acta A. 2021, 252, 119462.
- Han, Y.; Cao, Y.; Bolisetty, S.; Tian, T.; Handschin, S.; Lu, C.; Mezzenga, R. Amyloid Fibril-Templated High-Performance Conductive Aerogels with Sensing Properties. Small 2020, 16, 2004932.
- Yuan Y.; Solin N. Protein-Based Flexible Conductive Aerogels for Piezoresistive Pressure Sensors. ACS Appl. Bio Mater. 2022, 5, 3360–3370. doi:10.1021/acsabm.2c00348
- Yuan, Y.; Wang, L.; Porcheddu, A.; Colacino, E.; Solin, N. Mechanochemical Preparation of Protein: Hydantoin Hybrids and Their Release Properties. Chem. Sus. Chem. 2022, 15, e202102097.
- Mains, J.; Lamprou, D.A.; McIntosh, L.; Oswald, I.D.; Urquhart, A.J. Beta-Adrenoceptor Antagonists Affect Amyloid Nanostructure; Amyloid Hydrogels as Drug Delivery Vehicles. Chem. Commun. 2013, 49, 5082–5084.
- Duraj-Thatte, A.M.; Manjula-Basavanna, A.; Courchesne, N.M.D.; Cannici, G.I.; Sánchez-Ferrer, A.; Frank, B.P.; van’t Hag, L.; Cotts, S.K.; Fairbrother, D.H.; Mezzenga, R.; Joshi, N.S. Water-Processable, Biodegradable and Coatable Aquaplastic from Engineered Biofilms. Nat. Chem. Biol. 2021, 17, 732–738.
- Nyström, G.; Fernández-Ronco, M.P.; Bolisetty, S.; Mazzotti, M.; Mezzenga, R. Amyloid Templated Gold Aerogels. Adv. Mater. 2016, 28, 472–478.
- Altamura, L.; Horvath, C.; Rengaraj, S.; Rongier, A.; Elouarzaki, K.; Gondran, C.; Maçon, A.L.; Vendrely, C.; Bouchiat, V.; Fontecave, M. et al. A Synthetic Redox Biofilm Made from Metalloprotein–Prion Domain Chimera Nanowires. Nat. Chem. 2017, 9, 157–163.
- Guttenplan, A.P.; Young, L.J.; Matak-Vinkovic, D.; Kaminski, C.F.; Knowles, T.P.; Itzhaki, L.S. Nanoscale Click-Reactive Scaffolds from Peptide Self-Assembly. J. Nanobiotechnol. 2017, 15, 1–8.
- Friščić, T.; Mottillo, C.; Titi, H.M. Mechanochemistry for Synthesis. Angew. Chem. Int. Ed. 2020, 132, 1030–1041.
- Rizzo, A.; Inganäs, O.; Solin, N. Preparation of Phosphorescent Amyloid-Like Protein Fibrils. Chem-Eur. J. 2010, 16, 4190–4195.
- Bäcklund, F.G.; Wigenius, J.; Westerlund, F.; Inganäs, O.; Solin, N. Amyloid Fibrils as Dispersing Agents for Oligothiophenes: Control of Photophysical Properties Through Nanoscale Templating and Flow Induced Fibril Alignment. J. Mater. Chem. C. 2014, 2, 7811–7822.
- Bäcklund, F.G.; Pallbo, J.; Solin, N. Controlling Amyloid Fibril Formation by Partial Stirring. Biopolymers 2016, 105, 249–259.
- Bäcklund, F.G.; Solin, N. Development and Application of Methodology for Rapid Screening of Potential Amyloid Probes. ACS Comb. Sci. 2014, 16, 721–729.
- Wang, L.; Solin, N. Preparation of Functionalized Protein Materials Assisted by Mechanochemistry. J. Mater. Sci. 2018, 53, 13719–13732.
- Bäcklund, F.G.; Solin, N. Tuning the Aqueous Self-Assembly Process of Insulin by a Hydrophobic Additive. RSC Adv. 2015, 5, 92254–92262.
- Wang, L.; Bäcklund, F.G.; Yuan, Y.; Nagamani, S.; Hanczyc, P.; Sznitko, L.; Solin, N. Air–Water Interface Assembly of Protein Nanofibrils Promoted by Hydrophobic Additives. ACS Sustainable Chem. Eng. 2021, 9, 9289–9299.
- Fischer, H.; Polikarpov, I.; Craievich, A.F. Average Protein Density Is a Molecular-Weight-Dependent Function. Protein Sci. 2004, 13, 2825–2828.
- Kruk, M.; Jaroniec, M.; Sayari, A. Application of Large Pore MCM-41 Molecular Sieves to Improve Pore Size Analysis Using Nitrogen Adsorption Measurements. Langmuir 1997, 13, 6267–6273.
- Colquhoun, C.; Draper, E.R.; Schweins, R.; Marcello, M.; Vadukul, D.; Serpell, L.C.; Adams, D.J. Controlling the Network Type in Self-Assembled Dipeptide Hydrogels. Soft. Matter. 2017, 13, 1914–1919.
- Ye, X.; Capezza, A.J.; Xiao, X.; Lendel, C.; Hedenqvist, M.S.; Kessler, V.G.; Olsson, R.T. Protein Nanofibrils and Their Hydrogel Formation with Metal Ions. ACS Nano. 2021, 15, 5341–5354.