ABSTRACT
The solvent-free preparation of unsymmetrical N-aryl-5,6-unsubstituted-1,4-dihydropyridines (DHPs) by ball-milling was investigated. Three different mechanochemical domino reactions (one-pot/one step or stepwise) were studied, the process parameters underlying any mechanochemical process were modulated and the differences with solution-based mechanistic pathways were disclosed. The selection of the most suitable method was driven by the physical state of both the reactants and the intermediates, while the physical state of the final 1,4-DHP directed the choice of the catalyst (Lewis vs Brønsted) and the type of work-up to recover the final products (by column chromatography vs precipitation in water). The results herein described are unprecedented in the arena of synthetic methods to access diversely substituted 1,4-DHPs, an N-heterocyclic scaffold relevant to both synthetic and medicinal purposes.
GRAPHICAL ABSTRACT
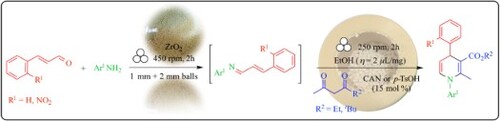
Introduction
Nowadays, the combination of enabling technologies with synthetic strategies and processes ‘benign by design’ and characterized by minimal waste generation represents a powerful approach to address the quest for a sustainable organic chemistry. In this context, synthetic strategies combining tandem, domino or cascade (Citation1, Citation2), with multicomponent reactions (Citation3) (MCR) have attracted increasing attention, being usually characterised by step and intrinsically high atom, step and pot economy while generating structural complexity and molecular diversity with operational simplicity and waste minimization. The efficiency and the low ecological footprint of these powerful synthetic tools can be additionally improved by conducting the reactions using mechanochemistry, an environmentally friendly technology closely intertwined with the 12 principles of Green Chemistry (Citation4,Citation5). The low environmental impact displayed by mechanochemical processes has been recently demonstrated for the preparation of Active Pharmaceutical Ingredients (APIs) by life cycle assessment studies in the continuous flow mechanochemical manufacturing of nitrofurantoin (Citation6), and its quantitative assessment against the 12 principles of green chemistry by using the DOZN 2.0 tool (Citation7). Therefore, the possibility to access complex biologically relevant structures and value-added compounds from simple precursors by mechanochemical domino or multicomponent reactions is very appealing (Citation8). Mechanochemically-activated synthetic approaches to heterocyclic systems were previously reported for both: (i) domino or cascade reactions to prepare dihydropyrroles and pyrroles (via oxidative cyclocondensation reactions) (Citation9), pyrazolones and isoxazolones (by asymmetric organocatalytic Mannich reaction-fluorination) (Citation10), indolylquinones (by Michael addition-oxidation sequence) (Citation11), and (ii) multicomponent reactions to access pyrroles (by Hantzsch pyrrole synthesis) (Citation12, 13), thiophenes (by Gewald reaction) (Citation14), coumarine thiazolidinones (Citation15), 4H-chromene derivatives (Citation16), dihydropyrimidones (Citation17) and 2,3-dihydro-1,2,6-thiadiazine 1-oxides (Citation18) (by Biginelli-type reaction), benzoxazines (by condensation/Mannich base ring-closure reactions) (Citation19), α-amino phosphonate derivatives (by Kabachnik-Fields reaction) (Citation20), or peptidic-like adducts (by Ugi 4-CR) and α-acyloxy amides (by Passerini-3CR) (Citation21).
In our ongoing work in the field of medicinal mechanochemistry (Citation22–25), applied to the sustainable preparation of pharmacologically relevant scaffolds (Citation26, 27), Active Pharmaceutical Ingredients (APIs) (Citation28–31) and World Health Organisation (WHO) essential medicines (Citation28, Citation32–35), we pointed our attention pointed towards the development of mechanochemical methods to access 1,4-dihydropyridines (1,4-DHPs), a N-heterocyclic scaffold relevant to both synthetic and medicinal purposes. The great versatility of the plethora of solution-based synthetic methods already available (Citation36–39), and the careful choice of the appropriate precursors, make 1,4-DHPs privileged scaffolds with a biological activity that can be tuned, in potency and selectivity, to the desired extent to target specific pharmacological effects in diverse therapeutic areas (Citation40–43). Several 1,4-DHPs are therapeutic agents used in medical practice worldwide as calcium-channel-blockers (e.g. nifedipine) for treating cardiovascular diseases (Citation44).
Hantzsch’s method, a 4-CR involving an aromatic aldehyde, a β-ketoester and ammonia, is the most common synthetic strategy to access N-unsubstituted symmetrical 1,4-DHPs (Scheme 1(a)) (Citation45). However, this method is unsuitable for preparing some relevant derivatives such as unsymmetrical N-aryl-1,4-dihydropyridines and N-aryl-5,6-unsubstituted-1,4-DHPs, which are still scarcely investigated for their biological activity, due to the lack of efficient synthetic methods. Moreover, due to the presence of a C5-C6-unsubstituted positions, they show enamine-like reactivity enabling the preparation of complex heterocyclic frameworks. To overcome these limitations, one of us (Citation46). previously reported a straightforward solution-based domino 3-CR of 1,4-DHPs from aromatic amines, α,b-unsaturated aldehydes and ethyl acetoacetate catalysed by cerium(IV) ammonium nitrate (CAN) at room temperature during one hour (Scheme 1(b)) (Citation46). The synthetic strategy relies on the use of α,β-unsaturated aldehydes, very often used as inexpensive bifunctional reagents employed as C-building blocks in the preparation of pyridines, 1,2-DHPs and 1,4-DHPs, due to the presence of two electrophilic centers (Citation37).
Scheme 1. MCRs for the preparation of diverse 1,4-DHP scaffolds: (a) and (b) in solution (Citation45, 46) or (c) solvent-free by manual grinding (Citation47) (EWG = electron-withdrawing group).
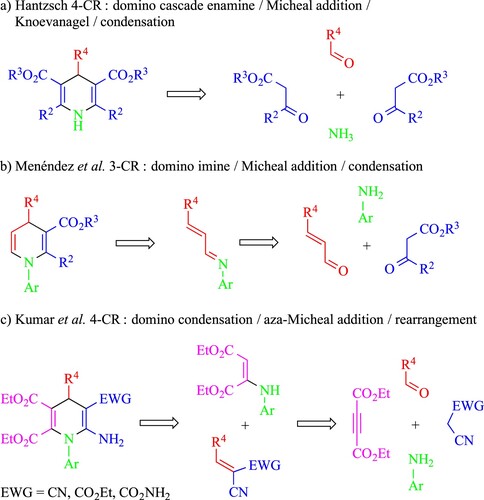
To the best of our knowledge, there are very few reports dealing with the use of ball-milling processes to prepare 1,4-DHPs, including some Hantzsch reaction of aromatic aldehydes, alkyl acetoacetates and alkyl 3-aminocrotonates promoted by Lipozyme® RM IM (triacylglycerol acylydrolase) (Citation48), and one report on the synthesis of unsymmetrical N-aryl-1,4-dihydropyridines by manual grinding (Scheme 1(c)) (Citation47). In the latter reaction, the domino MCR involved the stoichiometric coupling of an aromatic aldehyde with an active methylene compound (malononitrile, ethyl cyanoacetate or cyanoacetamide) and an aza-Michael reaction of an aniline on diethyl acetylenedicarboxylate (DEAD). The rearrangement of the intermediates generated the corresponding N-aryl-1,4-dihydropyridines (Scheme 1(c)) (Citation47, Citation49).
By virtue of the importance of 1,4-DHP scaffold in synthetic and medicinal chemistry the use of mechanochemical methods to access them becomes particularly appealing. Based on our previous findings in solution (Scheme 1(b)) (Citation46), we report herein the solvent-free preparation of N-aryl-5,6-unsubstituted-1,4-DHPs by ball-milling. The purpose of this endeavor is to: (i) improve the ecological footprint of the solution-based methods, (ii) avoid the use of any critical raw materials (Citation50) (e.g. the rare light earth elements such as cerium) or heterogeneous catalysts (Citation38), and (iii) investigate the reactivity and selectivity of the reactive system by three different mechanochemical methods, one-pot/one step or stepwise, to highlight the differences with solution-based mechanistic pathways (Citation51). Process parameters were investigated in neat or liquid-assisted grinding (Citation52) (LAG) conditions, including the influence of the milling speed, milling stress and milling media, the size and number of the milling balls, with or without additives.
Results and discussion
Optimization of Reaction Conditions. Based on the CAN-promoted synthetic strategy previously reported by us in solution (Scheme 1(b)) (Citation46), aniline, cinnamaldehyde and ethyl acetoacetate were selected as benchmark substrates to both optimize the reaction conditions to prepare 1,4-DHP 3 and to investigate the influence of process parameters in the ball mill. The study was conducted according to three different synthetic pathways: (i) the one-pot/one-step domino 3-CR (Method A, Scheme 2, based on the previously reported method in solution (Citation46)), (ii) the one-pot /two-step 3-CR involving the formation of an imine 1 (step 1), followed by the 1,4-Michael conjugate addition/ cyclocondensation sequence (step 2) (Method B1, Scheme 2), and (iii) the one-pot /two-step 3-CR involving the formation of a β-enamino ester 2 (step 1), telescoped with the cinnamaldehyde through a 1,4-Michael addition/cyclization and dehydration sequence (step 2) (Method B2, Scheme 2). In all cases, water was generated as the only by-product of the reaction.
After some unsuccessful experiments with a vibratory ball mill (see Supporting Information), Method A was investigated in a planetary ball mill. To this end, equimolar amounts of the reactants and the Lewis catalyst CAN (Citation46, 53) (5 mol %) were ball milled for 4 h at 450 rpm, in a ZrO2 jar containing 25 balls of 5 mm diameter of the same material. Even if the analysis of the crude by GC-MS was promising, showing that 1,4-DHP 3 was the major product (60%), a residual amount of aniline (13%) was still present, together with a by-product (27%) that was tentatively identified, based on its GC-MS fragmentation, as the corresponding compound generated by the competitive 1,2-addition reaction of ethyl acetoacetate to E-cinnamaldehyde, not previously reported in solution nor detected under vibrating ball milling. Furthermore, liquid-assisted grinding (Citation52) (LAG) conditions with EtOH (55.1 μL, η = 0.1 μL/mg) were detrimental, leading to an additional unidentified by-product, as indicated by the GC-MS analysis of the crude mixture. In all cases, GC-MS analyses of the crude showed that the rapid (and undesired) oxidative aromatization of 1,4-DHPs to the corresponding pyridines, previously reported with excellent yields in the presence of CAN (1.5 equiv) by manual grinding over 15 min (Citation54, 55), did not occur at all. Replacement of the Lewis acidic catalyst CAN by p-toluene sulfonic acid (Citation56, 57) (p-TsOH, 5 mol %) led to identical results, while no reaction occurred when using the organocatalyst L-proline (Citation58) (10 mol %). In this case, the 1,4-DHP 3 was not formed at all and the 1,2-addition reaction was predominant. Whatever the reaction and process conditions, the formation of the 1,2-addition by-product could not be avoided, strongly suggesting that the one-pot/one-step 3-CR procedure commonly successful in solution was unsuitable for ball-milling. To overcome the above-mentioned limitations and to circumvent the formation of the 1,2-addition by-product, the reaction was also studied in stepwise manners (Methods B1 and B2, Scheme 2).
To optimise the reaction conditions and the mechanochemical parameters, the synthesis of imine 1 from E-cinnamaldehyde and aniline was investigated (step 1, Method B1, Scheme 2) and a selection of data is reported in . In addition, process parameters such as type of mechanical stress (in a planetary or vibrating ball-mill), milling speed, milling time, type of grinding media (stainless steel or zirconium oxide), number and size of the milling balls, in neat conditions or using liquid-assisted grinding (LAG) procedures, with or without additive, were investigated.
Table 1. Selected data for the screening of the reaction and milling conditions for the preparation of imines 1 (R = H) and 4 (R = NO2) (Method B1, step 1, Scheme 2).Table Footnotea
In our ongoing work on MCR activated by ball-milling (Citation19, 20), we previously reported the successful mechanochemical synthesis of E-imines using both stainless steel (SS) and zirconium oxide as grinding media (Citation20). Therefore, two parallel experiments were performed in 12 mL SS and zirconium oxide jars containing 25 balls (5 mm diameter, total weight mtot = 12.7 g for SS and mtot = 10.3 g for ZrO2) (, entries 1 and 2), milling a stoichiometric amount of the reactants at 450 rpm (in a planetary ball mill) during two hours. Analyses of the crude mixtures showed that better conversions were obtained with ZrO2 as grinding media, with only 10% of residual aldehyde (vs 26% for SS) still present in the crude, together with the corresponding E-imine 1. This result confirmed our previous findings on the role of the jar material (Citation20), responsible for specific surface-mediated phenomena influencing the outcome of the reaction.
No substantial improvements were observed when milling the reactants in the presence of MgSO4, as solid support and dehydrating agent (entry 4) or reducing the milling stress frequency (Citation59) (using 3 ball of 10 mm diameter, total weight mtot = 8.8 g, entry 5), leading to a similar amount of residual aldehyde (12-13%). The reaction was repeated by extending the milling time to 3 h (entry 2) but the conversion was decreased, indeed, the amount of water formed during ball-mill could be responsible for aldehyde hydration, which hamper the formation of the E-imine 1. When changing the type of mechanical stress and the milling stress frequency (Citation59) (using 3 balls of 10 mm diameter at 50 Hz, total weight mtot = 8.8 g, entry 6), conversion was also lower, and the amount of residual aldehyde increased (21% and 28% respectively).
It was also speculated that the outcome of the reaction was driven by the rheology of the mixture: at the beginning of the synthesis, both reactants are liquids, so that no mechanical effects can be evoked in this stage. However, as soon as the E-imine 1 is formed, the mixture turned to a solid, desirable in view of the subsequent mechanochemical step (Scheme 2, Method B1, step 2). The visual inspection of the crude mixture containing the newly formed solid E-imine 1 showed a non-homogenous aspect, with zones characterized by a finely dispersed pale-yellow powder and others appearing as wet clumps. Postulating that better conversions could be achieved by avoiding the formation of the clumps, a set of experiments was planned to increase the milling stress frequency. The modulation of this process parameter was achieved by reducing the size of the balls, while keeping their weighted amount close to that one used in the most promising experiment (entry 2). Therefore, the 25 balls in ZrO2 (5 mm, mtot = 10.3 g) were replaced by 1 mm or 2 mm ZrO2 balls (mtot = 10.3 g, , entries 7 and 10). The results were comparable to those already obtained so far, and were not improved by adding MgSO4 (entry 9) or by doubling the weighted amount of 2 mm balls (entry 8), (i.e. 9-13% of residual aldehyde still detectable by GC-MS in the crude mixture).
A possible strategy to reduce the clumps, to keep both increased stress frequency and the individual impacts more energetic, was to mix balls of different sizes (, entries 11–15). Their relative weighted amount was also investigated: in two cases (entries 12 and 15), better results were obtained (e.g. the residual amount of cinnamaldehyde was 6–7%), while the addition of EtOH as LAG solvent was detrimental, also when a solid reactant such as o-nitrocinnamaldehyde (entry 13) was used, or by increasing the amount of 1 mm ZrO2 balls (entry 14). Despite all the attempts, it was impossible to achieve complete conversion of the starting materials, even when an excess of aniline (1.5 equiv) was added.
Having disclosed the suitable process conditions to prepare E-imine 1 (, entry 12) in the ball mill, the optimization of the cascade reaction, i.e. 1,4-conjugate addition / cyclisation / dehydration occurring in the second step (Scheme 2, Method B1, step 2) was directly carried out on the crude obtained from step 1.
Therefore, aiming to disclose the most suitable catalyst between CAN or p-TsOH, two experiments were run in parallel. To the jar already containing E-imine 1 and the mixture of zirconium oxide balls having 1 mm (mtot = 5.5 g) and 2 mm (mtot = 10.3 g) diameter, a stoichiometric amount of ethyl acetoacetate was added, together with the catalyst (15 mol%) and EtOH (1.27 mL, η = 2 μL/mg) (Citation60). The mixture was ball-milled at 450 rpm for two hours, then the crudes were analysed by GC-MS. It was found that, for both experiments, the E-imine was fully converted, but the 1,4-DHP 3 was formed together with the by-product 5, arising from the competing 1,2-nucleophilic addition to the aldehyde function. This result was also confirmed by the 1H NMR analyses of the crude mixture. Aiming to avoid the formation of by-product 5, the new set of experiments was conducted in the same milling conditions as before (for both steps 1 and 2), except for the milling speed, now reduced to 250 rpm (Scheme 3).
Scheme 3. Optimised reaction conditions for the one-pot/two-step synthesis of 1,4-DHPs via Method B1 in a ball mill. Legend: yields vs method for product recovery for compounds 6 and 7: black: by precipitation in water, purple: by liquid-liquid extraction.
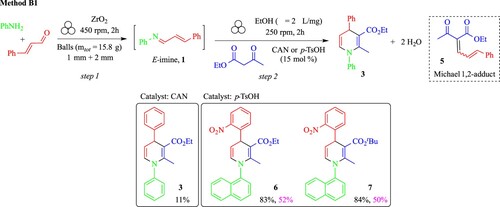
For both catalysts, the GC-MS analyses showed similar reactivity and selectivity profiles: 1,4-DHP 3 was the major product of the reaction together with traces (1%) of by-product 5. 1H NMR analyses of both crudes in CDCl3 revealed that the 1,4-DHP 3 obtained by the CAN-catalysed reaction underwent to a fast degradation during the analyses, as also confirmed later by an aging experiment during 12 h in the NMR tube, complemented by GC-MS analyses run in parallel. This is not surprising, considering the strong oxidizing character of CAN catalyst, particularly effective for the oxidative aromatisation of 1,4-DHP to pyridines (Citation54). However, this side reaction did not occur during ball-milling, as demonstrated by GC-MS analyses of the crude mixture immediately after the jar was opened. No characteristic signals of the corresponding pyridine by-product could be detected immediately after milling, the degradation occurring in solution upon exposure of the sample to the air.
Any attempt to recover the final product 3 by precipitation from water or any other (green) solvents was unsuccessful for both crude mixtures issued by CAN or p-TsOH catalysed reaction. This was due to the oily physical state of the final product, that could be recovered later on only by a chromatographic purification, impacting negatively the sustainability of the entire process, and being the cause of low yield. Moreover, if the CAN-catalysed reaction lead 1,4-DHP 3 in a disappointing 11% yield after column, the chromatographic purification of the crude mixture issued from p-TsOH catalysed reaction was not successful, due to the concomitant formation of an unidentified by-product that was co-eluted with the desired 1,4-DHP 3.
To verify the hypotheses above and to potentially reconsider p-TsOH as a valuable alternative catalyst to CAN, solid 1,4-DHPs needed to be prepared, in order to allow an easier recovery by a straightforward work-up based on precipitation in water, followed by in vacuo filtration.
Therefore, solid reactants such as 1-naphthylamine and o-nitrocinnamaldehyde were selected as starting reagents for step 1. Two reactions were run in parallel for two hours at 450 rpm in the same milling conditions disclosed so far (Scheme 3), leading to the corresponding E-imine 4 (). In the second step, p-TsOH (15 mol%) was added, and different alkyl acetoacetates (Alkyl = Et, t-Bu) in each experiment. The mixtures were ball-milled at 250 rpm for two hours according to Method B1 (Scheme 2), and the solid final product was recovered by precipitation from water followed by filtration. In both cases, the corresponding 1,4-DHPs 6 and 7 were obtained in very satisfying and comparable yields (83% and 84% respectively), without any other degradation by-products, as demonstrated by 1H NMR analyses, even upon aging. Compounds 6 and 7 were isolated as a mixture of two species as shown by 1H NMR analyses, in a 1:0.6 ratio for compound 6 (with a relative proportion of 62: 38) and 1:0.8 ratio for compound 7 (with a relative proportion of 56: 44), due to the presence of axial chirality. 1H NMR experiments in DMSO-d6 were conducted by heating up compounds 6 and 7 progressively and stepwise with 10°C incremental heating from room temperature to 100°C, but no modification of the ratios could be observed. This behavior can be ascribed to restricted rotation around the C4-Ar bond (Citation46) or the N-Ar bond (Citation61), and it will be subjected to further studies.
To confirm that the recovery of the product by a standard ‘organic’ solvent-based work-up negatively impacted the final yields, the experiments were repeated and the products recovered by a work-up involving a liquid–liquid extraction followed by a purification by column chromatography. As a result, the yields dropped drastically, to 52% and 50% respectively for compounds 6 and 7, confirming the initial assumption.
Being the reactivity and the selectivity of both catalysts the same in the milling conditions disclosed for Method B1, the choice of the most appropriate catalyst strictly depended on the physical state of the final 1,4-DHP, driving the choice of the work-up to be used for its recovery. Generally speaking, CAN is the suitable catalyst if the final target is an oil requiring a purification by column chromatography. At the same time, for solid 1,4-DHPs that can be recovered by precipitation in water, p-TsOH is a valuable alternative, not only because as Brønsted acid enhances the electrophilicity of the imine, favoring the 1,4-conjugate addition of the β-ketoester (Citation62), but also to avoid the risks associated to the CAN-promoted aerobic over-oxidation to pyridines.
To study the mechanism of the reaction in the ball mill, the preparation of 1,4-DHP 3 was also explored by Method B2 (Scheme 1), involving the formation of a β-enaminoester intermediate, combining the nucleophilicity of the enamine and the electrophilicity of enones. For Method B2, the same process conditions disclosed in Method B1 (Scheme 3) were used. Aniline and ethyl acetoacetate were milled together in the first step for two hours at 450 rpm, leading to the corresponding β-enaminocarbonyl intermediate. The 1,4-Michael addition to cinnamaldehyde/cyclisation/dehydration occurred in the second step in the presence of Lewis catalysts CAN (15 mol%), milling the mixture for two additional hours. Even if the reactivity, selectivity and yield of the system were comparable to those obtained starting from the E-imine derivative (Method B1), the rheology corresponding to the formation of the β-enaminocarbonyl derivative corresponded to an oily texture, ruling out the possibility to evoke any mechanochemical activation for both the first and the second step of the reaction. Thus, it can be concluded that the preparation of 1,4-DHPs was equally possible by both stepwise addition pathways (Methods B1 and B2) or by the simultaneous addition of the three reaction components. However, Method B1 remains the most promising, mainly due to: (i) its better selectivity in comparison with Method A, and (ii) its better rheological profile, leading to a solid E-imine intermediate (step 1).
The reaction scope towards the preparation of unsymmetrical N-aryl-5,6-unsubstituted-1,4-diaryl DHPs was investigated using the optimized ball-milling Method B1, with structural variations introduced simultaneously at the N-1 (Ar1 substituent), at C-4 (Ar2 substituent) and at the ester functional group (R2 substituent) (Scheme 4). Beyond the physical state of the final target (solid or liquid), key to choose the catalyst to be used, the reactivity of the system seemed to be driven by the nature of substitution: (i) on the enals (e.g. for compounds 3 vs 10, 8 vs 13, and 9 vs 14), with slightly better results obtained when o-nitrocinnamaldehyde was used, (ii) on the aniline component, with the better results obtained with electron-donating groups (EDG) (e.g. EDG = OMe, Me vs Ph, Cl, for compounds 11 and 16 vs 10, 11 vs 13 and 12 vs 14), enhancing the nucleophilicity of the amino group, while (iii) the reactivity was independent on the β-ketoester alkyl substitution (e.g. with alkyl = Et vs tBu for compounds 8 vs 9, 11 vs 12, 13 vs 14 and 16 vs 17).
Scheme 4. Reaction scope using Method B1. Legend: comparative yields are given in black: for ball-milling Method B1, in blue: for solution-based synthesis via one-pot/one-step domino reaction using CAN as catalyst,(Citation46) in purple: for other solution-based methods involving catalysts other than CAN and p-TsOH
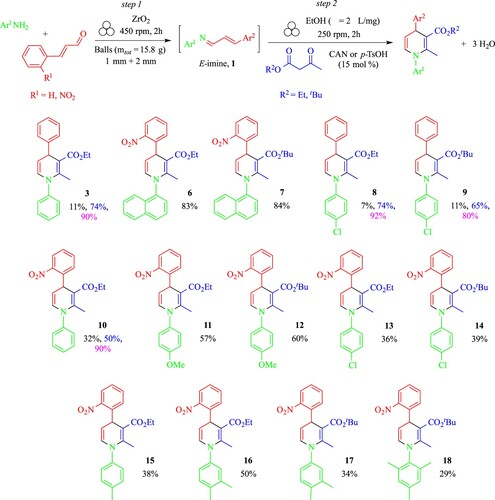
Unfortunately, the yields of 1,4-DHPs prepared by Method B1 were only moderate in many cases, especially in comparison with CAN-promoted synthesis in solution (Citation46), and in general, with respect to other solution-based methods with different catalysts (e.g. I2, (Citation63) Mg(ClO4)2 (Citation64) or HClO4.SiO2, silica-supported sulfonic acid,56 and metal oxides such as nano CuO (Citation65), nano Fe3O4 (Citation66) or SO42-/CexZr1-xO2 (Citation67)) (Scheme 4), despite that the crudes of the reactions were generally clean, as assessed by GC/MS and 1H NMR analyses. The isolation of compounds 8–18 presented the same difficulties already encountered for the purification of 1,4-DHP 3, due to the lability of these compounds, which quickly oxidized on SiO2 in the presence of an oxygen atmosphere (Citation64). The limited number of synthetic methods to prepare N-aryl-5,6-unsubstituted-1,4-DHPs and their unstable character in some conditions have limited the number of studies finalized to investigate their biological activity. However, 1,4-DHP 3 (R2 = Et) displayed antidyslipidemic activity, lowering cholesterol or triglyceride levels in plasma, while compound 14 (R2 = tBu) acted as a potent antioxidant, the activity in vitro being modulated by the nature of the R2 substituent at the ester functional group (Scheme 4) (Citation63).
The first quantitatively assessment of the greenness of mechanochemical processes against the 12 Principles of Green Chemistry was applied to the preparation of the World Health Organisation (WHO) Essential Medicine nitrofurantoin (Citation7), using the free, web-based scoring matrix DOZNTM 2.0 tool (Citation68, Citation69). It provides a unified set of metrics, organizing the 12 Principles of Green Chemistry into three major groups: improved resource use (G1, Group 1: principles # 1, 2, 7, 8, 9 and 11), increased energy efficiency (G2, Group 2: principle # 6), and reduced human and environmental hazards (G3, Group 3: principles # 3, 4, 5, 10 and 12), providing a score for the individual 12 principles, and aggregating the greenness scores (aggregated score) of processes on a scale of 0–100, with 0 being the most desirable one (Citation68).
Therefore, DOZN 2.0 greenness scores were generated for the synthesis of compound 1,4-DHP 3 by ball milling process (Method B1) in comparison with the solvent-based procedure (Citation46) ().
Table 2. Comparative scores for the preparation of 1,4-DHP 3 by ball milling vs. solvent-based procedure (Citation70).
Both processes display a DOZN 2.0 aggregated score of 1, however, the scores are lower for each group of principles in the ball mill (Method B1) indicating a greener synthesis compared to solution-based process. The scores were calculated by neglecting the quantities and nature of raw materials and solvents used for the work-up.
Although a purification by chromatographic column is necessary for both solution-based and by ball-mill methods, fewer numbers of raw materials (and solvents) were used in the work-up for method B1 (Citation3) by ball milling than for the solution-based process (Citation6), also requiring several washings by liquid–liquid extraction (Citation71). This makes the process by ball milling greener and economic (# 2, # 4, and # 7) and also less hazardous and inherently safer (# 3 and # 12).
Both processes use of the same reactants to make the same product (1,4-DHP 3), the differences in scores arises from the amount of EtOH used in the synthesis. For Group 1 (improved resource use), Method B1 received low scores because the raw material EtOH is used as grinding additive and not as solvent. This adds to the overall resource use without adding to the final product yield, generating a higher score for solvent-based process in Group 1 and for Group 3 (reduced human and environmental hazards), that quantifies the amount of waste produced, the severity of the waste, and the hazard level of the product being produced. In the case of Group 2 (energy efficiency) both processes received a zero score because there is no deviation from ambient conditions with regard to temperature and pressure.
Conclusions
The preparation of N-aryl-5,6-unsubstituted-1,4-DHPs was disclosed in the ball mill and the reactivity of the system was investigated according to three different domino reactions (one-pot/one-step or stepwise), and the process parameters underlying any mechanochemical process where modulated. As a result, the selection of the most suitable method was driven by the physical state of both the reactants and the intermediates, while the physical state of the final 1,4-DHP directed the choice of the catalyst (Lewis vs BrØnsted) and the type of work-up to recover the final products (by column chromatography vs precipitation in water). The quantitatively assessment of the greenness of Method B1 by ball milling against the 12 Principles of Green Chemistry was done in comparison with the solution-based procedure, showing the advantages of ball milling approach.
Despite the challenges to be faced in the preparation of N-aryl-5,6-unsubstituted-1,4-DHPs, in solution or by ball-milling, the results herein described are unprecedented in the arena of synthetic methods to access diversely substituted 1,4-DHPs. Hopefully they will pave the way towards the systematic use of mechanochemical procedures to prepare other pharmacologically relevant compounds, containing 1,4-DHP scaffolds.
Supporting information
Experimental procedures, 1H and 13C spectral data and full characterization for all of the synthesized compounds are available in the supporting information file.
Authors’ contribution
C.B.B. experimental data production, analyses and manuscript preparation; E.C. conceptualization, supervision, data curation, manuscript preparation and funding; J.C.M.: supervision, conceptualization, manuscript editing and funding; J.F.G. manuscript editing; D.V.: manuscript editing and funding; A.P. manuscript editing and data curation.
Research ethics
No requirement for completing an ethical assessment prior to conducting this research.
Supplemental Material
Download PDF (3.4 MB)Acknowledgments
This article is based upon work from COST Action CA18112 Mechanochemistry for Sustainable Industry, supported by COST (European Cooperation in Science and Technology). COST (European Cooperation in Science and Technology) is a funding agency for research and innovation networks. Our Actions help connect research initiatives across Europe and enable scientists to grow their ideas by sharing them with their peers. This boosts their research, career and innovation. www.cost.eu. E.C. expresses her gratitude towards BetaInnov (www.beta-innov.com) for providing the planetary milling equipment for conducting the research project. E.C. is grateful to Région Occitanie (France) for the Pre-Maturation 2020 − MECH-API grant (ESR_PRE-MAT − 00262). C.B.B. and J.C.M. gratefully acknowledge funding from MICINN (grant RTI2018-097662-B-I00), to the E.U. (Erasmus+ Program, Complutense University of Madrid, Spain) for a fellowship and to the COST Action CA18112 ‘Mechanochemistry for Sustainable Industry’ (Citation72, 73) for the Short Term Scientific Mission (STSM) mobility grant. D.V. is grateful to SATT AXLR for the Proof of Concept 2020 − MITH/D grant. A. P. is grateful to MIUR Italy, PRIN 2017 project (grant number: 226 2017B7MMJ5_001) ‘MultIFunctional poLymer cOmposites based on groWn matERials’ (MI-FLOWER) and Fondazione di Sardegna (FdS, F72F20000230007). The results reported in this manuscript were obtained during the worldwide outbreak due to COVID-19 pandemic disease.
Disclosure statement
No potential conflict of interest was reported by the author(s).
Data availability
Data are available as part of the electronic supplementary material.
Additional information
Funding
References
- Domino Reactions: Concepts for Efficient Organic Synthesis. L.F. Tietze, Ed., Wiley-VCH, Weinheim (Germany), pp. 621. ISBN:978-3-527-33432-2 2014.
- Volla, C. M. R.; Atodiresei, I.; Rueping, M., Catalytic C-C Bond-Forming Multi-Component Cascade or Domino Reactions: Pushing the Boundaries of Complexity in Asymmetric Organocatalysis. Chem. Rev. 2014, 114, 2390–2431. DOI:10.1021/cr400215u.
- Zhu, J.; Bienaymé, H., Multicomponent Reactions. Weinheim: Wiley-VCH, 2005.
- Ardila-Fierro, K. J.; Hernández, J. G., Sustainability Assessment of Mechanochemistry by Using the Twelve Principles of Green Chemistry. ChemSusChem. 2021, 14, 2145–2162. DOI: 10.1002/cssc.202100478.
- Colacino, E.; Isoni, V.; Crawford, D.; Garcia, F., Upscaling Mechanochemistry: Challenges and Perspectives. Trends Chem. 2021, 3, 335–339. DOI: 10.1016/j.trechm.2021.02.008.
- Galant, O.; Cerfeda, G.; McCalmont, A. S.; James, S. L.; Porcheddu, A.; Delogu, F.; Crawford, D. E.; Colacino, E.; Spatari, S., Mechanochemistry Can Reduce Life Cycle Environmental Impacts of Manufacturing Active Pharmaceutical Ingredients. ACS Sustain. Chem. Eng. 2022, 10, 1430–1439. DOI:10.1021/acssuschemeng.1c06434.
- Sharma, P.; Vetter, C.; Ponnusamy, E.; Colacino, E., Assessing the Greenness of Mechanochemical Processes by DOZN 2.0 Tool. ACS Sustain. Chem. Eng. 2022, 10, 5110–5116. DOI: 10.1021/acssuschemeng.1c07981.
- Leonardi, M.; Villacampa, M.; J.-C., M., Multicomponent Mechanochemical Synthesis. Chem. Sci. 2018, 9, 2042–2064. DOI: 10.1039/C7SC05370C.
- Xu, H.; Liu, H.-W.; Chen, K.; Wang, G.-W., One-Pot Multicomponent Mechanosynthesis of Polysubstituted Trans-2,3-Dihydropyrroles and Pyrroles from Amines, Alkyne Esters, and Chalcones. J. Org. Chem. 2018, 83, 6035–6049. DOI: 10.1021/acs.joc.8b00665.
- Krištofíková, D.; Mečiarová, M.; Rakovský, E.; Šebesta, R., Mechanochemically Activated Asymmetric Organocatalytic Domino Mannich Reaction-Fluorination. ACS Sustain. Chem. Eng. 2020, 8, 14417–14424. DOI: 10.1021/acssuschemeng.0c04260.
- Piquero, M.; Font, C.; Gullón, N.; López-Alvarado, P.; Menéndez, J.-C., One-Pot Mechanochemical Synthesis of Mono- and Bis-Indolylquinones via Solvent-Free Multiple Bond-Forming Processes. ChemSusChem. 2021, 14, 4764–4775. DOI: 10.1002/cssc.202101529.
- Leonardi, M.; Estévez, V.; Villacampa, M.; Menéndez, J. C., Diversity-Oriented Synthesis of Complex Pyrrole-Based Architectures from Very Simple Starting Materials. Adv. Synth. Catal. 2019, 361, 2054–2074. DOI: 10.1002/adsc.201900044.
- Leonardi, M.; Villacampa, M.; Menéndez, J.-C., Mild and General Synthesis of Pyrrolo[2,1-a]Isoquinolines and Related Polyheterocyclic Frameworks from Pyrrole Precursors Derived from a Mechanochemical Multicomponent Reaction. J. Org. Chem. 2017, 82, 2570–2578. DOI: 10.1021/acs.joc.6b02995.
- Shearouse, W. C.; Shumba, M. Z.; Mack, J., A Solvent-Free, One-Step, One-Pot Gewald Reaction for Alkyl-Aryl Ketones via Mechanochemistry. Appl. Sci. 2014, 4, 171–179. DOI: 10.3390/app4020171.
- Jadhav, S. A.; Shioorkar, M. G.; Chavan, O. S.; Chavan, R. V.; Pardeshi, R. K., An eco-Friendly Solvent-Free one-pot Multi-Component Synthesis of Coumarin Thiazolidinone Derivatives. Pharma Chem. 2015, 7, 329–334.
- Dekamin, M. G.; Eslami, M., Highly Efficient Organocatalytic Synthesis of Diverse and Densely Functionalized 2-Amino-3-Cyano-4H-Pyrans Under Mechanochemical Ball Milling. Green Chem. 2014, 16, 4914–4921. DOI: 10.1039/c4gc00411f.
- Sahoo, P. K.; Bose, A.; Mal, P., Solvent-Free Ball-Milling Biginelli Reaction by Subcomponent Synthesis. Eur. J. Org. Chem. 2015, 2015, 6994–6998. DOI: 10.1002/ejoc.201501039.
- Krauskopf, F.; Truong, K.-N.; Rissanen, K.; Bolm, C., 2,3-Dihydro-1,2,6-Thiadiazine 1-Oxides by Biginelli-Type Reactions with Sulfonimidamides Under Mechanochemical Conditions. Org. Lett. 2021, 23, 2699–2703. DOI: 10.1021/acs.orglett.1c00596
- Martina, K.; Rotolo, L.; Porcheddu, A.; Delogu, F.; Bysouth, S. R.; Cravotto, G.; Colacino, E., High Throughput Mechanochemistry: Application to Parallel Synthesis of Benzoxazines. Chem. Comm. 2018, 54, 551–554. DOI: 10.1039/C7CC07758K
- Fiore, C.; Sović, I.; Lukin, S.; Halasz, I.; Martina, K.; Delogu, F.; Ricci, P. C.; Porcheddu, A.; Schemchuk, O.; Braga, D.; Pirat, J.-L.; Virieux, D.; Colacino, E., The Kabachnik-Fields Reaction by Mechanochemistry: New Horizons from old Methods. ACS Sustain. Chem. Eng. 2020, 8, 18889–18902. DOI: 10.1021/acssuschemeng.0c05744.
- Polindara-García, L. A.; Juaristi, E., Synthesis of Ugi 4-CR and Passerini 3-CR Adducts Under Mechanochemical Activation. Eur. J. Org. Chem. 2016, 2016, 1095–1102. DOI: 10.1002/ejoc.201501371.
- Colacino, E.; Porcheddu, A.; Charnay, C.; Delogu, F., From Enabling Technologies to Medicinal Mechanochemistry: An eco-Friendly Access to Hydantoin-Based Active Pharmaceutical Ingredients. React. Chem. Eng. 2019, 4, 1179–1188. DOI: 10.1039/c9re00069k.
- Tan, D.; Loots, L.; Friščić, T., Towards Medicinal Mechanochemistry: Evolution of Milling from Pharmaceutical Solid Form Screening to the Synthesis of Active Pharmaceutical Ingredients (APIs). Chem. Commun. 2016, 52, 7760–7781. DOI: 10.1039/C6CC02015A.
- Ying, P.; Yu, J.; Su, W., Liquid-Assisted Grinding Mechanochemistry in the Synthesis of Pharmaceuticals. Adv. Synth. Catal. 2021, 363, 1246–1271. DOI: 10.1002/adsc.202001245.
- Pérez-Venegas, M.; Juaristi, E., Mechanochemical and Mechanoenzymatic Synthesis of Pharmacologically Active Compounds: A Green Perspective. ACS Sustain. Chem. Eng. 2020, 8, 8881–8893. DOI: 10.1021/acssuschemeng.0c01645.
- Gonnet, L.; Tintillier, T.; Venturini, N.; Konnert, L.; Hernandez, J.-F.; Lamaty, F.; Laconde, G.; Martinez, J.; Colacino, E., N-acyl Benzotriazole Derivatives for the Synthesis of di- and Tripeptides and Peptide Biotinylation by Mechanochemistry. ACS Sustain. Chem. Eng. 2017, 5, 2936–2941. DOI: 10.1021/acssuschemeng.6b02439
- Yuan, Y.; Wang, L.; Porcheddu, A.; Colacino, E.; Solin, N., Mechanochemical Preparation of Protein: Hydantoin Hybrids and Their Release Properties. ChemSusChem. 2022, 15, in press. DOI: 10.1002/cssc.202102097.
- Konnert, L.; Reneaud, B.; Marcia de Figueiredo, R.; Campagne, J.-M.; Lamaty, F.; Martinez, J.; Colacino, E., Mechanochemical Preparation of Hydantoins from Amino Esters: Application to the Synthesis of the Antiepileptic Drug Phenytoin. J. Org. Chem. 2014, 79, 10132–10142. DOI: 10.1021/jo5017629.
- Konnert, L.; Dimassi, M.; Gonnet, L.; Lamaty, F.; Martinez, J.; Colacino, E., Poly(Ethylene) Glycols and Mechanochemistry for the Preparation of Bioactive 3,5-Disubstituted Hydantoins. RSC Adv. 2016, 6, 36978–36986. DOI: 10.1039/c6ra03222b.
- Porcheddu, A.; Delogu, F.; De Luca, L.; Colacino, E., From Lossen Transposition to Solventless Medicinal Mechanochemistry”. ACS Sustain. Chem. Eng. 2019, 7, 12044–12051. DOI: 10.1021/acssuschemeng.9b00709.
- Martins, I. C. B.; Carta, M.; Haferkamp, S.; Feller, T.; Delogu, F.; Colacino, E.; Emmerling, F., Mechanochemical N-Chlorination Reaction of Hydantoin: In Situ Real-Time Kinetic Study by Powder X-ray Diffraction and Raman Spectroscopy. ACS Sustain. Chem. Eng. 2021, 9, 12591–12601. DOI: 10.1021/acssuschemeng.1c03812.
- Colacino, E.; Porcheddu, A.; Halasz, I.; Charnay, C.; Delogu, F.; Guerra, R.; Fullenwarth, J., Mechanochemistry for “no Solvent, no Base” Preparation of Hydantoin-Based Active Pharmaceutical Ingredients: Nitrofurantoin and Dantrolene. Green Chem. 2018, 20, 2973–2977. DOI: 10.1039/C8GC01345D.
- Crawford, D. E.; Porcheddu, A.; McCalmont, A. S.; Delogu, F.; James, S. L.; Colacino, E., Twin-Screw Extrusion for Hydrazone Synthesis, Generating Active Pharmaceutical Ingredients (APIs). ACS Sustain. Chem. Eng. 2020, 8, 12230–12238. DOI: 10.1021/acssuschemeng.0c03816.
- Sović, I.; Lukin, S.; Meštrović, E.; Halasz, I.; Porcheddu, A.; Delogu, F.; Ricci, P. C.; Caron, F.; Perilli, T.; Dogan, A.; Colacino, E., Mechanochemical Preparation of Active Pharmaceutical Ingredients Monitored by in Situ Raman Spectroscopy. ACS Omega 2020, 5, 28663–28672. DOI: 10.1021/acsomega.0c03756.
- Mocci, R.; Colacino, E.; De Luca, L.; Fattuoni, C.; Porcheddu, A.; Delogu, F., The Mechanochemical Beckmann Rearrangement: An Eco-Efficient “Cut-and-Paste” Strategy to Design “The Good Old Amide Bond. ACS Sustain. Chem. Eng. 2021, 9, 2100–2114. DOI: 10.1021/acssuschemeng.0c07254.
- Wan, J.-P.; Liu, Y., Recent Advances in new Multicomponent Synthesis of Structurally Diversified 1,4-Dihydropyridines. RSC Adv. 2012, 2, 9763–9777. DOI: 10.1039/c2ra21406g.
- Vchislo, N. V., α,β-Unsaturated Aldehydes as C-Building Blocks in the Synthesis of Pyridines, 1,4-Dihydropyridines and 1,2-Dihydropyridines. Asian J. Chem. 2019, 8, 1207–1226. DOI: 10.1002/ajoc.201900275.
- Anantha, I. S. S.; Kerru, N.; Maddila, S.; Jonnalagadda, S. B., Recent Progresses in the Multicomponent Synthesis of Dihydropyridines by Applying Sustainable Catalysts Under Green Conditions. Front. Chem. 2021, 9800236. DOI: 10.3389/fchem.2021.800236.
- Sharma, V. K.; Singh, S. K., Synthesis, Utility and Medicinal Importance of 1,2- & 1,4-Dihydropyridines. RSC Adv. 2017, 7, 2682–2732. DOI: 10.1039/c6ra24823c.
- Reddy, G. M.; Shiradkar, M.; Chakravarthy, A. K., Chemical and Pharmacological Significance of 1,4-Dihydropyridines. Curr. Org. Chem. 2007, 11, 847–852. DOI: 10.2174/138527207781024058.
- Talwan, P.; Chaudhary, S.; Kumar, K.; Rawal, R. K., Chemical and Medicinal Versatility of Substituted 1,4-Dihydropyridines. Curr. Bioact. Compd. 2017, 13, 109–120. DOI:10.2174/1573407212666160607090202.
- Malhi, D. S.; Kaur, M.; Soh, H. S., Effect of Substitutions on 1, 4-Dihdropyridines to Achieve Potential Anti-Microbial Drugs: A Review. Chem. Select. 2019, 4, 11321–11336. DOI: 10.1002/slct.201902354
- Carosati, E.; Ioan, P.; Micucci, M.; Broccatelli, F.; Cruciani, G.; Zhorov, B. S.; Chiarini, A.; Budriesi, R., 1,4-Dihydropyridine Scaffold in Medicinal Chemistry, The Story So Far And Perspectives (Part 2): Action in Other Targets and Antitargets. Curr. Med. Chem. 2012, 19, 4306–4323. DOI: 10.2174/092986712802884204.
- Gerber, J. G. a. N., A. S. Gilman and Goodman’s the Pharmacological Basis of Therapeutics. 8th edn.: Gilman, A. G., Rall, T. W., Nies, A. S. and Taylor, P., Eds.; Pergamon Press: New York, 1990.
- Hantzsch, A., Condensationsprodukte aus Aldehydammoniak und Ketonartigen Verbindungen. Ber. Dtsch. Chem. Ges. 1881, 14, 1637–1638. DOI: 10.1002/cber.18810140214.
- Sridharan, V.; Perumal, P. T.; Avendano, C.; Menéndez, J.-C., A new Three-Component Domino Synthesis of 1,4-Dihydropyridines. Tetrahedron 2007, 63, 4407–4413. DOI: 10.1016/j.tet.2007.03.092.
- Kumar, A.; Sharma, S., A Grinding-Induced Catalyst- and Solvent-Free Synthesis of Highly Functionalized 1,4-Dihydropyridines via a Domino Multicomponent Reaction. Green Chem. 2011, 13, 2017–2020. DOI: 10.1039/c1gc15223h.
- Jiang, L.; Ye, L.-D.; Gy, J.-L.; Su, W.-K.; Ye, W.-T., Mechanochemical Enzymatic Synthesis of 1,4-Dihydropyridine Calcium Antagonists and Derivatives. J. Chem. Tech. Biotechnol. 2019, 94, 2555–2560. DOI: 10.1002/jctb.6051.
- For a review on mechanochemical rearrangement, p. s. V., D.; Delogu, F.; Porcheddu, A.; Garcia, F.; Colacino, E. ‘Mechanochemical rearrangements’ J. Org. Chem. 2021, 86, 13885−13894. DOI: 10.1021/acs.joc.1c01323
- For the European Commission's list of critical raw materials (CRMs), please visit. https://ec.europa.eu/growth/sectors/raw-materials/areas-specific-interest/critical-raw-materials_en (accessed May 31, 2022).
- Porcheddu, A.; Cuccu, F.; De Luca, L.; Delogu, F.; Colacino, E.; Solin, N.; Mocci, R., Mechanochemistry: New Tools to Navigate the Uncharted Territory of “Impossible” Reactions”. ChemSusChem. 2022, in press. DOI: 10.1002/cssc.202200362R1.
- Hasa, D.; Jones, W., Screening for new Pharmaceutical Solid Forms Using Mechanochemistry: A Practical Guide. Adv. Drug. Del. Rev. 2017, 117, 147–161. DOI: 10.1016/j.addr.2017.05.001.
- For a review on the use of CAN as Lewis acid catalyst, please see; Sridharan, V.; Menéndez, J. C., Cerium(IV) ammonium nitrate as a catalyst in organic synthesis. Chem. Rev. 2010, 110, 3805–3849. DOI: 10.1021/cr100004p
- Khanna, R.; Dalal, A.; Kadyan, K.; Kumar, R.; Kumar, P.; Kamboj, R. C., CAN Mediated Mechanochemical Synthesis of Substituted Pyridine Derivatives. Lett. Org. Chem. 2018, 15, 673–677. DOI: 10.2174/1570178615666180102153707.
- For an additional example of oxidative aromatisation to pyridines mediated by hypervalent iodine reagents, p. s. K., P., Solid State Oxidative Aromatization of Hantzsch 1,4-Dihydropyridines to Pyridines Using Iodobenzene Diacetate or Hydroxy(tosyloxy)iodobenzene. Chin. J. Chem. 2009, 27, 1487-1491. DOI: 10.1002/cjoc.200990250
- Das, B.; Suneel, K.; Venkateswarlu, K.; Ravikanth, B., Sulfonic Acid Functionalized Silica: An Efficient Heterogeneous Catalyst for a Three-Component Synthesis of 1,4-Dihydropyridines Under Solvent-Free Conditions. Chem. Pharm. Bull. 2008, 56, 366–368. DOI: 10.1248/cpb.56.366.
- Liu, F.-J.; Sun, T.-T.; Yang, Y.-G.; Huang, C.; Chen, X.-B., Divergent Synthesis of Dual 1,4-Dihydropyridines with Different Substituted Patterns from Enaminones and Aldehydes Through Domino Reactions. RSC Adv. 2018, 8, 12635–12640. DOI: 10.1039/C8RA01236A.
- Kumar, A.; Maurya, R. A., Organocatalysed Three-Component Domino Synthesis of 1,4- Dihydropyridines Under Solvent Free Conditions. Tetrahedron 2008, 64, 3477–3482. DOI: 10.1016/j.tet.2008.02.022.
- Stolle, A.; Schmidt, R.; Jacob, K., Scale-up of Organic Reactions in Ball Mills: Process Intensification with Regard to Energy Efficiency and Economy of Scale. Faraday Discuss. 2014, 170, 267–286. DOI: 10.1039/C3FD00144J.
- The reaction was initially explored in neat grinding conditions. Initial tests using EtOH as LAG solvent were encouraging. Therefore, the amount of EtOH to be added was determined after a series of experiments, using incremental amounts of LAG solvent, screening the reaction with η = 0.1, 0.2, 0.5, 1, and 2 μL/mg. The best results were obtained with η = 2 μL/mg.
- Cardenas, M. M.; Nguyen, A. D.; Brown, Z. E.; Heydari, B. S.; Heydari, B. S.; Vaidya, S. D.; Gustafson, J. L., Atropisomerism as Inspiration for new Chemistry. ARKIVOC 2021, part i, 20-47. DOI: 10.24820/ark.5550190.p011.382.
- Jiang, J.; Yu, J.; Sun, X.-X.; Rao, Q.-Q.; Gong, L.-Z., Organocatalytic Asymmetric Three-Component Cyclization of Cinnamaldehydes and Primary Amines with 1,3-Dicarbonyl Compounds: Straightforward Access to Enantiomerically Enriched Dihydropyridines. Angew. Chem. Int. Ed. 2008, 47, 2458–2462. DOI: 10.1002/anie.200705300.
- Kumar, A.; Maurya, R. A.; Sharma, S.; Kumar, M.; Bhatia, G., Synthesis and Biological Evaluation of N-Aryl-1,4-Dihydropyridines as Novel Antidyslipidemic and Antioxidant Agents. Eur. J. Med. Chem. 2010, 45, 501–509. DOI: 10.1016/j.ejmech.2009.10.036.
- Bartoli, G.; Babiuch, K.; Bosco, M.; Carlone, A.; Galzerano, P.; Melchiorre, P.; Sambri, L., Simple and Convenient Route Giu to 1,4-Dihydropyridines Synlett 2007, 18, 2897–2901. DOI: 10.1055/s-2007-990839.
- Lakshmi Kantam, M.; Ramani, T.; Chakrapani, L.; Choudary, B. M., Synthesis of 1,4-Dihydropyridine Derivatives Using Nanocrystalline Copper(II) Oxide Catalyst. Catal. Commun. 2009, 10, 370–372. DOI: 10.1016/j.catcom.2008.09.023.
- Yang, S.-H.; Zhao, F.-Y.; Lü, H.-Y.; Deng, J.; Zhang, Z.-H., An Efficient One-Pot Synthesis of 1,4-Dihydropyridines Catalyzed by Magnetic Nanocrystalline Fe3O4. J. Heter. Chem. 2012, 49, 1126–1129. DOI: 10.1002/jhet.953.
- Kahandal, S. S.; Kale, S. R.; Gawande, M. B.; Jayaram, R. V., A Mild Route for one pot Synthesis of 5,6-Unsubstituted 1,4-Dihydropyridines Catalyzed by Sulphated Mixed Metal Oxides. Catal. Sci. Technol. 2014, 4, 672–680. DOI: 10.1039/C3CY00651D.
- Krueder, A. D.; T. House-Knight; J. Whitford; E. Ponnusamy; P. Miller; N. Jesse; R. Rodenborn; S. Sayag; M. Gebel; I. Aped; I. Sharfstein; E. Manaster; I. Ergaz; A. Harris; Grice, L. N., A Method for Assessing Greener Alternatives Between Chemical Products Following the 12 Principles of Green Chemistry. ACS Sustain. Chem. Eng. 2017, 5, 2927–2935. DOI: 10.1021/acssuschemeng.6b02399. DOZN tool is a universal tool, suitable for scoring any process, no matter the activation technique used, once the detailed experimental/process conditions are known. The DOZN 2.0 tool is accessible free of charge here: https://bioinfo.merckgroup.com/dozn (accessed September 3, 2022).
- Ponnusamy, E. S.; Whitford, J., A new Greener Alternative Scoring Matrix Based on Twelve Principles of Green Chemistry. Asian J. Sci. Technol. 2018, 9, 7312–7318.
- Please refer to ESI for the scores calculated for each of the 12 Principles of Green Chemistry.
- Veillasamy, S.; Paramasivan, T. P.; Avendaño, C.; Menéndez, J. C., A New Three-Component Domino Synthesis of 1,4-Dihydropyridines. Tetrahedron 2007, 63, 4407–4413. DOI: 10.1016/j.tet.2007.03.092.
- For more information on COST Action CA18112 ‘Mechanochemistry for Sustainable Industry’. http://www.mechsustind.eu/ (accessed May 21, 2022).
- Hernández, J. G.; Halasz, I.; Crawford, D. E.; Krupička, M.; Baláž, M.; André, V.; Vella-Zarb, L.; Niidu, A.; García, F.; Maini, L.; Colacino, E., European Research in Focus: Mechanochemistry for Sustainable Industry (MechSustInd). Eur. J. Org. Chem. 2020, 8–9. DOI: 10.1002/ejoc.201901718