ABSTRACT
In response to environmental concerns and restrictions on isocyanate-based materials, researchers and the coatings industry are focused on developing eco-friendly isocyanate-free polyurethanes. This article introduces a novel class of environmentally-friendly, initiator/catalyst-free, UV-curable, self-healing non-isocyanate polyurethanes (NIPUs) synthesized from bio-based carbonated soybean oil (CSO) and non-toxic coumarin. The synthesis of these polymers is based on using a photo-reactive coumarin that undergoes a reversible [2 + 2] cycloaddition upon exposure to the wavelength of UV light. UV-curable three coumarin-terminated isocyanate-free polyurethane prepolymers were synthesized using CSO and three different amines and epoxy coumarin. Subsequently, a set of cross-linked NIPU polymers were obtained with exposure of 365 nm UV irradiation. The photo-reversible nature of these polymers was investigated in response to various wavelengths of UV radiation. Additionally, their self-healing ability and the thermal and mechanical properties of NIPU coatings were studied using optical microscopy, thermogravimetric analysis, differential scanning calorimetry, and a universal testing machine. The outcomes demonstrate that this polyurethane has the potential to provide a sustainable alternative to isocyanate-based materials. Two examples of stimulated healing are given, that of healing a scratch and the other being the healing of a sample that has been mechanically stressed to failure in a tensile mode.
GRAPHICAL ABSTRACT
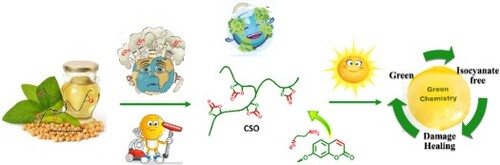
Introduction
The need for environmentally friendly, functional polymeric materials continues to motivate researchers to use renewable resources to create intelligent and advanced polymeric materials, as these renewable resources can reduce dependence on fossil substituents and decrease greenhouse gas emissions (Citation1,Citation2). With worldwide consumption of 7% (Citation3), polyurethanes (PUs) are among the essential polymers used in our daily life, with a broad range of applications, such as coatings, adhesives, foams, sealants, clothes, packaging, drug delivery and elastomers (Citation4–11). However, traditional synthesis of PUs was carried out via polycondensation between polyols and diisocyanates (Citation12), where isocyanates were derived from highly toxic phosgene (Citation13). In addition, the waste material that remains in the used products can potentially harm human health and the environment (Citation14,Citation15). Therefore, in the last two decades researchers have developed alternative ‘green’ non-isocyanate polyurethanes (NIPUs) pathways to address these issues. The more efficient and sustainable method for the synthesis of NIPUs is ring-opening polymerization of multifunctional cyclic carbonates with difunctional amines (Citation16). Indeed, cyclic carbonates are promising and safe intermediates employed as building blocks for commercial monomers, polymers, curing agents, plasticizers, surfactants, cross-linking agents, and environmentally friendly solvents with high boiling temperatures (Citation17). In addition, the synthesis of cyclic carbonates involves the insertion of CO2 into epoxides, which has been considered one of the most promising strategies for transforming CO2 into chemical raw materials with added value (Citation18,Citation19). Moreover, the NIPUs synthesized via the cyclic carbonate route have better thermal stability and water and chemical resistance than the traditional PUs (Citation20).
Adhering to the principles of green chemistry, the use of bio-based starting materials can further improve the sustainability aspects of NIPUs and reduce their dependence on fossil fuels (Citation21–24). Renewable raw materials, such as plant oils, furfural, limonene and lignin-derived materials are the known starting materials for the synthesis of cyclic carbonates, followed by the NIPUs (Citation24–29). Among these, epoxidized soybean oil with an adequate number of epoxy groups, commercial availability, biocompatibility, renewability, easy processability and easy synthesis from non-toxic soybean oil make it a promising raw material for the synthesis of bio-based cyclic carbonates and NIPUs (Citation30–34).
Self-healing polymers are promising for a range of applications, especially when material longevity and durability are essential. Furthermore, self-healing polymers can repair themselves when damaged, thus improving the material's lifespan and reducing waste generation. In recent years, significant progress has been made in developing self-healing polymers based on various physical and chemical mechanisms (Citation35). Introducing dynamic covalent bonds into polymer chains is an effective strategy for creating healable polymeric networks, as these bonds are reversible autonomously or in the presence of external stimuli (Citation36–38). Due to the advantages associated with the self-healing polymeric materials, researchers are keen to develop self-healing NIPUs. However, few attempts have been reported with regards to the synthesis of self-healable NIPUs from carbonated soybean oil (CSO). Xiuxiu and his co-workers reported the self-healing NIPUs from CSO and renewable 1,8-menthane diamine using the advantage of dynamic carbamate bonds (Citation30). Jincheng et al. utilized disulfide and hydrogen bonds to fabricate self-healable NIPUs from CSO (Citation39). Recently Xinxin and colleagues synthesized a self-healing NIPU from CSO using disulfide exchange and transcarbamylation chemistries (Citation40). However, these NIPU systems from CSO require elevated temperatures for their formation and healing. In addition, the systems possess poor mechanical properties and most of these films are opaque (Citation30,Citation40).
Light-stimulated polymerization is a green alternative to thermal methods because it can produce polymers at room temperature and in solid states, eliminating toxic solvent utilization (Citation41). In recent years, light stimulated [2 + 2] dimerization reactions have gained much attention as these reactions can be carried out without a catalyst or initiator, and no by-products are formed (Citation38,Citation42). Moreover, these reactions are reversible and can be achieved by simply varying the wavelength of the light source (Citation43–45). For example, the most common moieties of coumarin, thymine and cinnamic acid readily undergo photo-reversible [2 + 2] cycloaddition reactions (Citation38). Among these, coumarin, which is plant-based and non-toxic with better reversible efficiency and readily available in many functionalized forms, gained must attention to exploring its reversible chemistries in the areas of self-healing coatings, photo-resists, reversible adhesives, smart membranes, hydrogels and polymer particles (Citation43,Citation46–54). For example, researchers utilized coumarins [2 + 2] reversible chemistries to fabricate the isocyanate-based self-healing coatings (Citation55–57). However, to the best of our knowledge, no reports are available that utilizes [2 + 2] reversible cycloadditions to synthesize UV-curable self-healing NIPUs.
In this study, we report new sustainable, catalyst-free, UV-curable and self-healing NIPUs from renewable CSO and non-toxic photo-active coumarin. The photo-active coumarin terminated NIPU prepolymer systems were synthesized using three different chain-length amines to fabricate cross-linked NIPU networks. The modification of CSO was carried out in eco-friendly and economical ways without using solvents and catalysts. As a result, the coumarin-terminated NIPU prepolymers readily form cross-linked rigid polymer networks via [2 + 2] cycloadditions of coumarins under 365 nm UV light. Further, irradiation of NIPUs with 254 nm UV radiation breaks the coumarin dimers and transforms the cross-linked materials into a flowable state to heal the damage. We examined the effect of the amine chain length on the curing kinetics and thermal, mechanical and self-healing properties of the obtained NIPUs. This study leads to the development of a new generation of photo-curable green NIPUs with enhanced and tuneable thermal and mechanical properties.
Materials and methods
Materials
Epoxidized soybean oil with an epoxy equivalent weight of 237 g (4.01 epoxy groups per triglyceride and had a molar mass of approximately 950 g/mol) was purchased from Makwell plasticizers, Mumbai, India. Tetrabutylammonium bromide, 7-hydroxycoumarin, ethylenediamine, hexamethylenediamine and 4,7,10-trioxa-1,13-tridecanediamine were purchased from Sigma-Aldrich, USA. Chloroform was obtained from Ajax Finechem, Australia.
Experimental procedures
Synthesis of carbonated soybean oil (CSO)
The carbonization of epoxy soybean oil (ESO) was carried out in a high-pressure reactor. To the reaction vessel, about 250 g of ESO and a catalytic amount of tetrabutylammonium bromide (TBAB, 8.8wt.%) with respect to the weight of ESO is added and the reaction mixture is continuously stirred and heated to 120°C. After the temperature is reached, CO2 was passed into the reactor, pressurized to 10 bars, and maintained for 24 hrs at 120°C. After the completion of the reaction, the pressure was released, and the viscous oil was collected through the exit valve located at the bottom of the reactor. The crude product was dissolved in ethyl acetate and washed thoroughly with distilled water in a separating funnel. The organic layer was dried over anhydrous Na2SO4, and the solvent was removed using a rotary evaporator to yield a highly viscous clear and brownish CSO. Yield 97% 1H NMR (400 MHz, CDCl3) δ 0.88 (m), 1.22 (m, br), 2.3 (s, br), 4.22 (m, br), 4.48 (m, br), 4.75 (m, br), 5.24 (s, br) ppm. 13C NMR (400 MHz, CDCl3) δ 13.9, 21.0, 22.3, 22.7, 24.1, 25.6, 26.6, 27.8, 29.7, 31.2, 31.9, 33.4, 34.0, 57.2, 60.4, 62.1, 68.9, 75.4, 79.4, 82.0, 153.8, 171.1, 172.7, 173.1 ppm. IR (ATR) 2925, 2855, 1794, 1738, 1462, 1372, 1167, 1048, 774 cm−1.
Synthesis of CSO-2-Coumarin
CSO 2 g (1.78 mmol) and 426 mg (7.1 mmol) of ethylenediamine were placed in a round bottom flask, and the reaction mixture was stirred for 1hr at 80°C. After 1 hr, 1.55 gm (7.1 mmol) of epoxy coumarin was added to the reaction mixture and the temperature increased to 135°C, and the reaction mixture was stirred for one more hour. The reaction mixture was allowed to cool to room temperature, dissolved in chloroform, and filtered, and the chloroform was removed under reduced pressure. The synthesized CSO-2-Coum was a yellow colored brittle solid. Yield 96%. 1H NMR (400 MHz, DMSO-d6) δ 0.85 (s, br), 1.22 (m, br), 2.26 (s, br), 2.6 (s, br), 3.02 (s, br), 3.95 (m, br), 4.52 (s, br), 5.17 (s, br), 6.27 (m, br), 6.96 (m, br), 7.59 (m, br), 7.989 (m, br) ppm. 13C NMR (400 MHz, DMSO-d6) δ 14.3, 22.5, 24.8, 28.8, 29.1, 29.4, 29,4, 31.72, 33.8, 62.2, 67.8, 68.4, 71.8, 101.6, 112.7, 129.9, 144.8, 155.7, 160.9, 162.4, 173.0 ppm. IR (ATR) 3347, 3083, 2924, 2854, 1720, 1611, 1554, 1509, 1459, 1230, 1122, 1027, 834, 774 cm−1.
Synthesis of CSO-6-Coumarin
CSO 2 g (1.78 mmol) and 824 mg (7.1 mmol) of hexamethylenediamine were placed in a round bottom flask, and the reaction mixture was stirred for 1hr at 80°C. After 1 hr, 1.55 gm (7.1 mmol) of epoxy coumarin was added to the reaction mixture and increased the temperature was increased to 135°C and the reaction mixture was stirred for one more hour. After cooling to room temperature, the reaction mixture dissolved in chloroform. It was then filtered, and the chloroform removed under reduced pressure. The synthesized CSO-2-Coum was a yellow colored brittle solid. Yield 96%. 1H NMR (400 MHz, DMSO-d6) δ 0.85 (s, br), 1.21 (m, br), 2.26 (s, br), 2.93 (s, br), 3.89 (m, br), 4.51 (s, br), 5.18 (s, br), 6.24 (m, br), 6.87 (m, br), 7.55 (m, br), 7.93 (m, br) ppm. 13C NMR (400 MHz, CDCl3) δ 14.39, 22.6, 29.2, 29.5, 29.9, 31.8, 49.9, 63.2, 68.4, 69.8, 71.9, 101.9, 112.8, 130.0, 144.8, 155.83, 162.4, 172.5 ppm. IR (ATR) 3345, 3081, 2924, 2854, 1721, 1708, 1611, 1543, 1509, 1459, 1230, 1122, 1028, 834, 774 cm−1.
Synthesis of CSO-13-Coumarin
CSO 2 g (1.78 mmol) and 1.56 gm (7.1 mmol) of 4,7,10-trioxa-1,13-tridecanediamine were placed in a round bottom flask, and the reaction mixture was stirred for 1hr at 80°C. After 1 hr, 1.55 gm (7.1 mmol) of epoxy coumarin was added to the reaction mixture and the temperature was increased to 135°C and stirred for one more hour, with the mixture being allowed to cool to room temperature, dissolved in chloroform, and filtered, and the chloroform was removed under reduced pressure. The synthesized CSO-2-Coum was a yellow colored sticky material. Yield 97%. 1H NMR (400 MHz, DMSO-d6) δ 0.83 (s, br), 1.20 (m, br), 2.24 (s, br), 2.57 (m, br), 3.0 (s, br), 3.44 (m, br), 3.93 (m, br), 4.48 (s, br), 5.15 (s, br), 6.24 (m, br), 6.94 (m, br), 7.58 (m, br), 7.95 (m, br) ppm. 13C NMR (400 MHz, CDCl3) δ 14.3, 22.5, 24.8, 27.3, 30.0, 31.7, 33.8, 36.3, 47.0, 52.5, 70.2, 71.9, 101.6, 112.7, 113.3, 130.0, 144.9, 155.7, 161.0, 162.4, 173.0 ppm. IR (ATR) 3344, 3081, 2924, 2856, 1725, 1709, 1612, 1555, 1509, 1459, 1230, 1122, 1029, 835, 773 cm−1.
Characterization
FTIR spectroscopic analysis was performed using an Agilent Cary 630 spectrometer with a resolution of 4 cm−1 in the range of 4000–600 cm−1, aggregating 60 scans. 1H NMR was recorded in the DMSO-d6 solvent on a 400 MHz Bruker DRX 400 NMR spectrometer. UV-Viss spectroscopic analysis was carried out on the UV-1800 Shimadzu spectrometer. Photo-polymerization and depolymerization reactions were carried out in UVP UV crosslinker CL-1000L 365 nm and CL-1000 254 nm, respectively. Thermal degradation of the synthesized polymer networks was analyzed on a Mettler Toledo TGA/DSC1 STAR instrument with a heating rate of 10°C/min under the nitrogen. The glass transition temperatures (Tg) of the cross-linked polymer networks were determined using a Perkin Elmer DSC8000 under a nitrogen atmosphere with a gas flow rate of 25 mL/min and a heating rate of 10°C/min. Mechanical properties of the polymers were determined on an Instron 5965 equipped with a 10 KN load cell at a speed of 5 mm min−1 at room temperature. Optical images of scratched and healed polymer surfaces were obtained from Olympus GX51 optical microscope.
Results and discussions
Synthesis of carbonated soybean oil
The carbonization of ESO was carried out as displayed in (Scheme 1). The synthesized CSO was characterized by using FTIR and 1H NMR. As shown in ,the characteristic absorption peak of the epoxy group at 821 cm−1 disappeared, and the appearance of new absorption peaks at 1794cm−1 correspond to carbonyl (C = O) stretching of the cyclic carbonate, which confirms the successful conversion of epoxy groups into cyclic carbonates (Citation30,Citation31). The conversion of ESO to CSO is further supported by 1H NMR analysis. As shown in , the peaks at 2.8 - 3.2 ppm belonging to the epoxy groups have disappeared, and new peaks belonging to the cyclic carbonate appeared at 4.4 - 5.0 ppm.
Synthesis and characterization of CSO-2-coum, CSO-6-Coum and CSO-13-Coum
In this work, three types of coumarin-terminated NIPU prepolymer systems were synthesized from carbonated soybean oil and three different amines were used such as ethylene diamine (ED), hexamethylene diamine (HMDA) and tridecane diamine (TDA), named as CSO-2-Coum, CSO-6-Coum and CSO-13-Coum. The reactions were carried out in a continuous two-step pathway without solvent and catalyst (Scheme 2). Initially, the CSO reacted with diamines to yield amine-terminated intermediates via ammonolysis of cyclic carbonate groups of the CSO. Amine-terminated intermediates were further reacted with epoxy coumarin to yield coumarin-terminated CSO-2-Coum, CSO-6-Coum and CSO-13-Coum. The epoxy coumarin was synthesized following our previous report from naturally-occurring umbelliferon (7-hydroxycoumarin) (Citation46).
Scheme 2. Synthetic route for coumarin terminated NIPU prepolymers and photo-reversible dimerization of prepolymers.
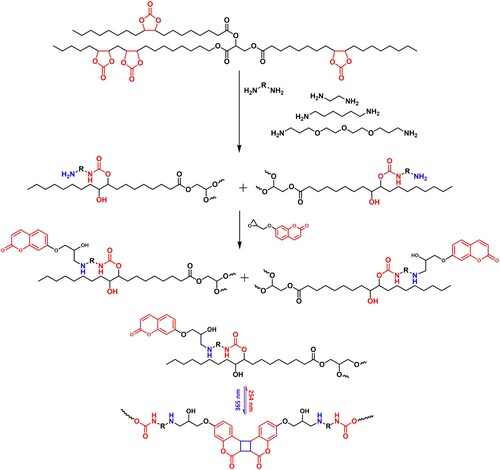
The amine-terminated intermediates from CSO were characterized by FTIR spectroscopy. shows the FTIR spectrums of the CSO and amine-terminated intermediates, all samples demonstrating a similar appearance. The characteristic absorption of the cyclic carbonate carbonyl group at 1794cm−1 disappeared in all three systems, which conforms to the ammonolysis of the cyclic carbonate. The appearance of new broad peaks at 3200 - 3400 cm−1 correspond to the O-H and N-H (free amine) stretching, and the peak 1694 and 1526 cm−1 correspond to the C = O stretching and N-H bending vibrations of the urethane linkage (Citation30,Citation39). The FTIR results confirm the successful formation of the amine-terminated intermediates.
Further, amine-terminated intermediates were reacted with epoxy coumarin to yield CSO-2-Coum, CSO-6-Coum and CSO-13-Coum. As shown in , the absorption in the 3200 - 3400 cm−1 region for all three becomes smoother, indicating the reaction between epoxy coumarin and free amines of amine-terminated intermediates. The appearance of new characteristic peaks of coumarin pyrone ring C = C stretching and C = C ̶ H bending vibrations at 1611 and 834 cm−1 confirmed the grafting of coumarin onto the amine-terminated intermediates. In addition, the low intensity peaks at 1654 cm−1 appeared in all three, materials corresponding to the amide from aminolysis of a triglyceride of CSO and diamines (Citation30,Citation33,Citation39). Further, 1H NMR spectroscopy was also used to characterize the chemical structures of CSO-2-Coum, CSO-6-Coum and CSO-13-Coum, as shown in , the appearance of new peaks corresponding to coumarin in the aromatic region and disappearance of peaks corresponding to cyclic carbonate, further supported the successful synthesis of the coumarin terminated NIPU prepolymers.
Photo-reversibility of NIPU's
Using the synthesized three prepolymers, four cross-linked NIPU polymers were produced: three polymers were synthesized separately from CSO-2-Coum, CSO-6-Coum and CSO-13-Coum and the fourth polymer was synthesized from the 50:50 wt % mixture of CSO-2-coum and CSO-13-Coum, which was further named as CSO-2 + 13-Coum. The polymerization was carried out at 365 nm, and depolymerization was done under 254 nm UV light irradiation. The formation of photo-crosslinked NIPU polymers and their photo-reversibility were examined using UV-Vis and FTIR spectroscopy. CSO-2-Coum, CSO-6-Coum, CSO-13-Coum and CSO-2 + 13-Coum were dissolved in chloroform and spin-coated over quartz slides, the samples were exposed to 365 nm UV light, and the progression and degree of the polymerization reaction determined by comparing and monitoring the signals from coumarin double bonds at 323 nm. , and Figure S1, S2 and S3 in the supporting information show the UV-Vis spectra of the samples at different irradiation times of polymerization, depolymerization and repolymerization. As shown in a, the absorbance at 323 nm corresponding to the coumarin double bond decreases significantly after each dose of irradiation for all the systems, indicating the dimerization of the coumarin units of the prepolymers. The percentage of reacted coumarin units was calculated to be 86%, 87%, 87% and 86% for CSO-2-Coum, CSO-6-Coum, CSO-13-Coum and CSO-2 + 13-Coum, respectively. To investigate the photo-reversible character of the formed NIPUs, the samples were subsequently irradiated with 254 nm UV radiation. With increasing irradiation time, the absorbance at 323 nm was increased (b), which indicated cleavage of the cyclobutane ring and reformation of coumarin double bonds. The absorbance increased with increasing irradiation dose and further irradiation showed no change in the absorbance due to the equilibrium between the forward and reverse reactions under 254 nm UV irradiation (Citation58). The calculation showed that 68%, 71%, 72% and 73% of the dimers were cleaved for CSO-2-Coum, CSO-6-Coum, CSO-13-Coum and CSO-2 + 13-Coum. Due to structural similarity, all of the systems showed similar polymerization and depolymerization characteristics. However, the NIPUs with flexible amines showed better decrosslinking than the short-chain amine, which is attributed to the greater mobility of the coumarin units. Samples were again irradiated with 365 nm of UV light to repolymerize them, as shown in c, the absorbance at 323 nm again decreased with increasing irradiation dose, and 96%, 94%, 98% and 99% of coumarins were repolymerized for CSO-2-Coum, CSO-6-Coum, CSO-13-Coum and CSO-2 + 13-Coum, respectively. d, and Figure S1d, S2d and S3d in the supporting information show FTIR analysis of pure prepolymers and polymerized, depolymerized and repolymerized of four NIPU systems. After the polymerization under the 365 nm UV light in all four systems, the carbonyl stretching frequencies shifted to higher wavenumbers (1708cm−1– 1740cm−1) due to the cleavage of C = C bonds of the pyrone ring of coumarin during the formation of coumarin dimers, along with the frequencies at 1611, and 1553 cm−1 corresponds the C = C shifted to 1621 and 1587 cm−1. Additionally, a reduction in peak intensities at 834 cm−1 corresponded to the C–H bending of C = C. The subsequent irradiation with 254 nm UV light cleaved the coumarin dimers and restored the C = C bonds of the pyrone ring of coumarin as a result, the carbonyl and C = C stretching frequencies were shifted to the original, and the intensities of C–H bending of C = C at 834 cm−1 restored. Again, irradiation with the 365 nm UV light caused the coumarin to undergo dimerization, and the carbonyl (C = O) and C = C stretching frequencies were shifted to the higher wave numbers and the intensities of C–H bending of C = C at 834 cm−1 decreased. The FTIR analysis further supports the successful reversible polymerization of the NIPUs with respect to the UV irradiation wavelengths.
Thermal properties of NIPUs
The thermal stability of the synthesized NIPUs was investigated using thermogravimetric analysis (TGA). Figure S4 in the supporting information shows the thermograms of the NIPUs. The NIPUs showed similar decomposition patterns with thermal stability (T10%) ranging from 250 - 280°C. The NIPUs showed better thermal stabilities compared to traditional UV-curable vegetable oil-based PUs derived from isocyanates and acrylates, which degrade at 200–270°C (T10%) (Citation59).
The glass transition temperature (Tg) of the NIPUs was measured using differential scanning calorimetry (DSC), and results are shown in Figure S5-S8 and . As expected, the NIPU CSO-13-Coum with more flexible diamines showed the lowest Tg of the four due to greater mobility, whereas CSO-2-Coum, with a shorter diamine, showed the highest Tg. The NIPUs CSO-6-Coum and CSO-2 + 13- Coum Tg values were between these values.
Table 1. Glass transition temperatures (Tg) of the NIPUs in the polymer state and after depolymerization.
The light-stimulated healing of the polymeric systems depends on the depolymerized Tg of the system (Citation44,Citation46,Citation60). Therefore, we calculated the depolymerized Tg of all systems after the irradiation with 254 nm UV light. The results are shown in Figure S9-S12 in the supporting information and . The results indicated a significant change in Tg for all four systems after the irradiation with 254 nm UV light, evidence of the successful depolymerization of NIPUs.
Light-stimulated scratch healing of the NIPUs
To study the light-stimulated healing of the NIPUs, the polymers were prepared on a glass slide under 365 nm of UV light, scratched with a sharp blade, the cracks having a width of 30 - 40 µm on the polymer surfaces (). The damaged polymer surfaces were then irradiated with 254 nm of UV light to initiate the healing process and observed using an optical microscope. The light-stimulated healing mechanism of the cross-linked photo-reversible polymer networks is based on the formation of flowable oligomeric/monomeric units during the depolymerization process of coumarin dimers, which can fill the scratched regions, reducing surface area (and thus decreasing surface energy), the mobility of the smaller units depending on their Tg. shows the schematic representation of the light-stimulated healing mechanism.
The damages on the surfaces of CSO-6-Coum, CSO-13-Coum and CSO-2 + 13- Coum NIPUs were healed with the 0.69, 2.1 J cm−2 dose of 254 nm irradiation, respectively (d, f and h). As the depolymerized polymers have a Tg below the room temperature (), the depolymerized materials are readily flow into the damaged areas, and scratches were healed. However, the CSO-2-Coum polymer surface was not healed completely (b). This is most likely because the rigid structural design and high Tg (45.4°C) after the depolymerization make it difficult for depolymerized materials to move freely into the scratched areas and fill them in order to cause healing (Citation46).
Figure 5. Light- Stimulated healing of polymerized CSO-2-Coum, CSO-6-Coum, CSO-13-Coum and CSO-2 + 13-Coum NIPUs under 254 nm irradiation at room temperature, a), c), e), g) scratches and b), d), f), h) healed surfaces.
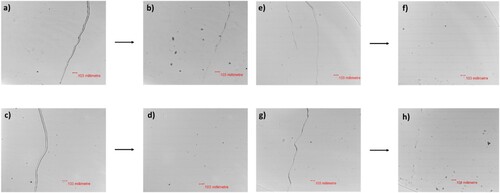
Table 2. Thermal and mechanical properties of NIPUs.
Mechanical properties of the NIPUs
To evaluate the mechanical properties of the NIPUs, the polymer films with a thickness of 25 −30 µm were prepared via solution casting followed by polymerizations under 365 nm UV radiation. Figure S13 in the supporting information shows the prepared NIPU film. The CSO-2-Coum results in a brittle film due to the shorter diamine and high cross-linking density. The mechanical properties of the NIPUs are summarized in . As shown in , by increasing the amine chain length from CSO-6-Coum to CSO-13-Coum, the tensile strength, Young’s modulus decreased, and elongation at break increased. The 50 + 50 wt% mixture of CSO-2-Coum and CSO-13-Coum mechanical properties lying between CSO-6-Coum and CSO-13-Coum. Moreover, the synthesized NIPUs showed better mechanical properties (10 - 36 MPa) than previously reported NIPUs based on CSO, which had values between 2 - 13 MPa (Citation30–32,Citation39,Citation40). This study also showed that the NIPUs can have their mechanical properties tailored to specific applications by adjusting the ratios of their prepolymer components.
Figure 6. a) Light-stimulated healing process of the NIPUs and Stress-Strain curves of b) pristine NIPUs and c) healed NIPUs.
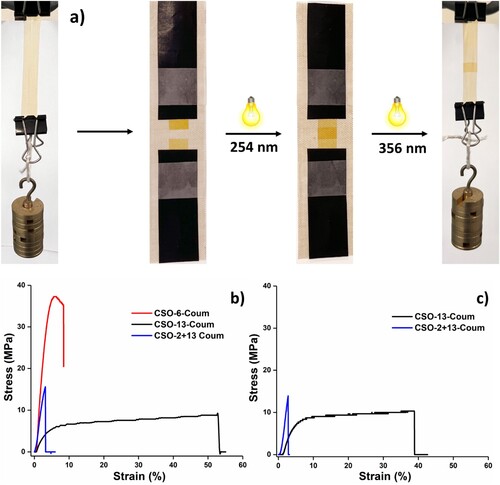
Further, we also investigated the mechanical properties of the healed samples. First, the samples were cut into two pieces using a scissor, and the cut samples were irradiated on an area of 50 mm2 with 254 nm UV light to initiate depolymerization (). Afterwards, the samples were held together by overlapping with an area of 50 mm2 and irradiated with 365 nm of UV radiation to polymerize () the samples. The healed samples of CSO-13-Coum and CSO-2 + 13 Coum also showed similar mechanical properties as pristine before they failed (). Moreover, the breakage did not occur at the healed position (Figure S14), which confirms the efficient and effective healing of NIPUs. However, the sample CSO-6-Coum was not healed, the two pieces separated without any force due to its glassy, rigid surface and high depolymerization Tg (27.8°C), which makes the depolymerized surfaces unable to adhere to each other as for the other softer samples, and thus unable to form a strong adhesive bond.
Conclusions
This work demonstrated sustainable catalyst-free UV-curable and self-healing NIPUs from plant-based CSO and non-toxic coumarins, which can assist in addressing environmental concerns and restrictions on isocyanate-based materials in the coatings industry. We successfully synthesized three coumarin-terminated NIPU prepolymers using different amines from CSO to create four photo-reversible cross-linked NIPU polymers using the photo-reversible dimerization from coumarins. All four polymer systems showed efficient photo-reversibility with alternating irradiation of 365 and 254 nm UV irradiation. The results show that the thermal, mechanical and self-healing properties of the NIPUs largely depend on the diamines used. The polymer derived from CSO-2-Coum, which possesses a short chain diamine (ED), resulted in a brittle coating with a Tg of 77.3°C, whereas the polymers CSO-6-Coum, CSO-13-Coum and CSO-2 + 13-Coum had a Tg of 13.2°C to 56.1°C. Moreover, the NIPUs showed better mechanical properties with a tensile strength of 10 ± 3 MPa to 36 ± 2 MPa compared to the previous studies. In addition, the thermal and mechanical properties of the NIPUs can be tuned by varying the prepolymer components. The self-healing abilities of the polymers were investigated by observing the scratches made on the coating surfaces under 254 nm UV irradiations. The polymers CSO-6-Coum, CSO-13-Coum and CSO-2 + 13-Coum efficiently healed at room temperature. In addition, we also investigated the mechanical properties of the healed polymers, and the results showed that healed polymers recovered to possess similar mechanical properties to pristine NIPUs.
Supplemental Material
Download MS Word (17.9 MB)Acknowledgments
The author thanks Monash University's Chemicals and Polymers Graduate Research Industry Partnership (GRIP) for funding KB's PhD with a scholarship. AP, JW, and KS also acknowledge the support of the Australian Research Council Industrial Transformation Training Centre (Green Chemistry in Manufacturing, Project number IC90100034), funded by the Australian Government. KS acknowledges support from JSPS KAKENHI Grant-in-Aid for Scientific Research(B) JP22H02121, Grant-in-Aid for Transformative Research Areas (B) JP22H05046, and Grant-in-Aid for Exploratory Research 22K19868.
Disclosure statement
No potential conflict of interest was reported by the author(s).
Additional information
Funding
References
- Bozell, J.J. Connecting Biomass and Petroleum Processing with a Chemical Bridge. Science 2010, 329 (5991), 522–523.
- Ding, C.; Shuttleworth, P.S.; Makin, S.; Clark, J.H.; Matharu, A.S. New Insights Into the Curing of Epoxidized Linseed oil with Dicarboxylic Acids. Green Chem. 2015, 17 (7), 4000–4008.
- Easley, A.; Mangano, A.; Fors, B.P. Safer Polyurethane Foams with Cyclic Carbonates. Angew. Chem., Int. Ed. 2023, 62 (20), 202218062.
- Boga, K.; Gaddam, S.K.; Chepuri, R.R.; Palanisamy, A. Development of Biobased Polyurethane-Imides from Maleinized Cottonseed oil and Castor oil. Polym. Adv. Technol. 2019, 30 (11), 2742–2749.
- Tenorio-Alfonso, A.; Sánchez, M.C.; Franco, J.M. A Review of the Sustainable Approaches in the Production of bio-Based Polyurethanes and Their Applications in the Adhesive Field. J. Polym. Environ. 2020, 28, 749–774.
- Skleničková, K.; Abbrent, S.; Halecký, M.; Kočí, V.; Beneš, H. Biodegradability and Ecotoxicity of Polyurethane Foams: A Review. Crit. Rev. Environ. Sci. Technol. 2022, 52 (2), 157–202.
- Lu, L.; Zhao, D.; Fan, J.; Li, G. A Brief Review of Sealants for Cement Concrete Pavement Joints and Cracks. Road Mater. Pavement Des. 2022, 23 (7), 1467–1491.
- Viscusi, G.; Lamberti, E.; D'Amico, F.; Tammaro, L.; Vigliotta, G.; Gorrasi, G. Design and Characterization of Polyurethane Based Electrospun Systems Modified with Transition Metals Oxides for Protective Clothing Applications. Appl. Surf. Sci. 2023, 617, 156563.
- Indumathi, M.; Rajarajeswari, G. Mahua oil-Based Polyurethane/Chitosan/Nano ZnO Composite Films for Biodegradable Food Packaging Applications. Int. J. Biol. Macromol. 2019, 124, 163–174.
- Gencturk, A.; Kahraman, E.; Güngör, S.; Özhan, G.; Özsoy, Y.; Sarac, A. Polyurethane/Hydroxypropyl Cellulose Electrospun Nanofiber Mats as Potential Transdermal Drug Delivery System: Characterization Studies and in Vitro Assays. Artif. Cells. Nanomed. Biotechnol. 2017, 45 (3), 655–664.
- Petrović, Z.S.; Ferguson, J. Polyurethane Elastomers. Prog. Polym. Sci. 1991, 16 (5), 695–836.
- Arukula, R.; Thota, A.; Boga, K.; Narayan, R.; Rao, C.R. Investigations on Anticorrosive, Thermal, and Mechanical Properties of Conducting Polyurethanes with Tetraaniline Pendent Groups. Polym. Adv. Technol. 2018, 29 (6), 1620–1631.
- Cotarca, L.; Eckert, H. Phosgenations: A Handbook; John Wiley & Sons: Weinheim, 2006.
- Arnold, S.M.; Collins, M.A.; Graham, C.; Jolly, A.T.; Parod, R.J.; Poole, A.; Schupp, T.; Shiotsuka, R.N.; Woolhiser, M.R. Risk Assessment for Consumer Exposure to Toluene Diisocyanate (TDI) Derived from Polyurethane Flexible Foam. Regul. Toxicol. Pharmacol. 2012, 64 (3), 504–515.
- Wang, Y.; Liu, X.; Wang, Y.; Zhao, J. Epoxy-free Synthesis of Aromatic Dicyclocarbonates and the Related Strong Epoxy Hybrid non-Isocyanate Polyurethanes. Mater. Today Commun. 2023, 34, 105263.
- Chen, H.; Chauhan, P.; Yan, N. “Barking” up the Right Tree: Biorefinery from Waste Stream to Cyclic Carbonate with Immobilization of CO2 for non-Isocyanate Polyurethanes. Green Chem. 2020, 22 (20), 6874–6888.
- Clements, J.H. Reactive Applications of Cyclic Alkylene Carbonates. Ind. Eng. Chem. Res. 2003, 42 (4), 663–674.
- Chen, F.; Liu, N.; Dai, B. Iron(II) Bis-CNN Pincer Complex-Catalyzed Cyclic Carbonate Synthesis at Room Temperature. ACS. Sustain. Chem. Eng. 2017, 5 (10), 9065–9075.
- Bayer, U.; Werner, D.; Maichle-Mössmer, C.; Anwander, R. Effective and Reversible Carbon Dioxide Insertion Into Cerium Pyrazolates. Angew. Chem., Int. Ed. 2020, 59 (14), 5830–5836.
- Wulf, C.; Reckers, M.; Perechodjuk, A.; Werner, T. Catalytic Systems for the Synthesis of Biscarbonates and Their Impact on the Sequential Preparation of non-Isocyanate Polyurethanes. ACS. Sustain. Chem. Eng. 2020, 8 (3), 1651–1658.
- Carré, C.; Ecochard, Y.; Caillol, S.; Avérous, L. From the Synthesis of Biobased Cyclic Carbonate to Polyhydroxyurethanes: A Promising Route Towards Renewable non-Isocyanate Polyurethanes. ChemSusChem. 2019, 12 (15), 3410–3430.
- Nohra, B.; Candy, L.; Blanco, J.-F.; Guerin, C.; Raoul, Y.; Mouloungui, Z. From Petrochemical Polyurethanes to Biobased Polyhydroxyurethanes. Macromolecules 2013, 46 (10), 3771–3792.
- Carré, C.; Bonnet, L.; Avérous, L. Original Biobased Nonisocyanate Polyurethanes: Solvent- and Catalyst-Free Synthesis, Thermal Properties and Rheological Behaviour. RSC Adv. 2014, 4 (96), 54018–54025.
- Ménard, R.l.; Caillol, S.; Allais, F. Chemo-Enzymatic Synthesis and Characterization of Renewable Thermoplastic and Thermoset Isocyanate-Free Poly(Hydroxy)Urethanes from Ferulic Acid Derivatives. ACS. Sustain. Chem. Eng. 2017, 5 (2), 1446–1456.
- Mokhtari, C.; Malek, F.; Manseri, A.; Caillol, S.; Negrell, C. Reactive Jojoba and Castor Oils-Based Cyclic Carbonates for Biobased Polyhydroxyurethanes. Eur. Polym. J. 2019, 113, 18–28.
- Zhang, W.; Wang, T.; Zheng, Z.; Quirino, R.L.; Xie, F.; Li, Y.; Zhang, C. Plant oil-Based non-Isocyanate Waterborne Poly(Hydroxyl Urethane)s. Chem. Eng. J. 2023, 452, 138965.
- de la Cruz-Martínez, F.; Martínez de Sarasa, M.; Buchaca, J.; Martínez, J.; Fernández-Baeza, L.F.; Sánchez-Barba, A.; Rodríguez-Diéguez, J.A.; Castro-Osma, A.N. Synthesis of Bio-Derived Cyclic Carbonates from Renewable Resources. ACS. Sustain. Chem. Eng. 2019, 7 (24), 20126–20138.
- Bähr, M.; Bitto, A.; Mülhaupt, R. Cyclic Limonene Dicarbonate as a new Monomer for non-Isocyanate Oligo- and Polyurethanes (NIPU) Based upon Terpenes. Green Chem. 2012, 14 (5), 1447–1454.
- Mhatre, S.V.; Mahajan, J.S.; Epps, T.H.; Korley, L.T. Lignin-derivable Alternatives to Petroleum-Derived non-Isocyanate Polyurethane Thermosets with Enhanced Toughness. Materials Advances 2023, 4 (1), 110–121.
- Liu, X.; Yang, X.; Wang, S.; Wang, S.; Wang, Z.; Liu, S.; Xu, X.; Liu, H.; Song, Z. Fully bio-Based Polyhydroxyurethanes with a Dynamic Network from a Terpene Derivative and Cyclic Carbonate Functional Soybean oil. ACS. Sustain. Chem. Eng. 2021, 9 (11), 4175–4184.
- Lee, A.; Deng, Y. Green Polyurethane from Lignin and Soybean oil Through non-Isocyanate Reactions. Eur. Polym. J. 2015, 63, 67–73.
- Poussard, L.; Mariage, J.; Grignard, B.; Detrembleur, C.; Jérôme, C.; Calberg, C.; Heinrichs, B.; De Winter, J.; Gerbaux, P.; Raquez, J.-M. Non-isocyanate Polyurethanes from Carbonated Soybean oil Using Monomeric or Oligomeric Diamines to Achieve Thermosets or Thermoplastics. Macromolecules 2016, 49 (6), 2162–2171.
- Samanta, S.; Selvakumar, S.; Bahr, J.; Wickramaratne, D.S.; Sibi, M.; Chisholm, B.J. Synthesis and Characterization of Polyurethane Networks Derived from Soybean-oil-Based Cyclic Carbonates and Bioderivable Diamines. ACS. Sustain. Chem. Eng. 2016, 4 (12), 6551–6561.
- Paraskar, P.M.; Prabhudesai, M.S.; Hatkar, V.M.; Kulkarni, R.D. Vegetable oil Based Polyurethane Coatings – A Sustainable Approach: A Review. Prog. Org. Coat. 2021, 156, 106267.
- Binder, W.H. Self-healing Polymers: From Principles to Applications; John Wiley & Sons: Weinheim, 2013.
- Zou, W.; Dong, J.; Luo, Y.; Zhao, Q.; Xie, T. Dynamic Covalent Polymer Networks: From old Chemistry to Modern day Innovations. Adv. Mater. 2017, 29 (14), 1606100.
- Chakma, P.; Konkolewicz, D. Dynamic Covalent Bonds in Polymeric Materials. Angew. Chem., Int. Ed. 2019, 58 (29), 9682–9695.
- Hughes, T.; Simon, G.; Saito, K. Chemistries and Capabilities of Photo-Formable and Photoreversible Cross-Linked Polymer Networks. Mater. Horiz. 2019, 6 (9), 1762–1773.
- Dong, J.; Liu, B.; Ding, H.; Shi, J.; Liu, N.; Dai, B.; Kim, I. Bio-based Healable non-Isocyanate Polyurethanes Driven by the Cooperation of Disulfide and Hydrogen Bonds. Polym. Chem. 2020, 11 (47), 7524–7532.
- Yang, X.; Wang, S.; Liu, X.; Huang, Z.; Huang, X.; Xu, X.; Liu, H.; Wang, D.; Shang, S. Preparation of non-Isocyanate Polyurethanes from Epoxy Soybean oil: Dual Dynamic Networks to Realize Self-Healing and Reprocessing Under Mild Conditions. Green Chem. 2021, 23 (17), 6349–6355.
- Niedner, L.; Kali, G. Green Engineered Polymers: Solvent Free, Room-Temperature Polymerization of Monomer from a Renewable Resource, Without Utilizing Initiator. Chemistry Select 2019, 4 (12), 3495–3499.
- Kaur, G.; Johnston, P.; Saito, K. Photo-reversible Dimerisation Reactions and Their Applications in Polymeric Systems. Polym. Chem. 2014, 5 (7), 2171–2186.
- Hughes, T.; Simon, G.; Saito, K. Improvement and Tuning of the Performance of Light-Healable Polymers by Variation of the Monomer Content. Polym. Chem. 2018, 9 (47), 5585–5593.
- Roy, P.S.; Mention, M.M.; Turner, M.A.; Brunissen, F.; Stavros, V.G.; Garnier, G.; Allais, F.; Saito, K. Bio-based Photo-Reversible Self-Healing Polymer Designed from Lignin. Green Chem. 2021, 23 (24), 10050–10061.
- Abdallh, M.; He, P.; Hearn, M.T.; Simon, G.P.; Saito, K. Light-Switchable Self-Healing Dynamic Linear Polymers: Reversible Cycloaddition Reactions of Thymine-Containing Units. Chem Plus Chem 2019, 84 (4), 333–337.
- Hughes, T.; Simon, G.; Saito, K. Photocuring of 4-arm Coumarin-Functionalised Monomers to Form Highly Photoreversible Crosslinked Epoxy Coatings. Polym. Chem. 2019, 10 (17), 2134–2142.
- Abdallh, M.; Yoshikawa, C.; Hearn, M.T.; Simon, G.P.; Saito, K. Photoreversible Smart Polymers Based on 2π + 2π Cycloaddition Reactions: Nanofilms to Self-Healing Films. Macromolecules 2019, 52 (6), 2446–2455.
- Abdallh, M.; Hearn, M.T.; Simon, G.P.; Saito, K. Light Triggered Self-Healing of Polyacrylate Polymers Cross-Linked with 7-Methacryloyoxycoumarin Crosslinker. Polym. Chem. 2017, 8 (38), 5875–5883.
- Inada, M.; Tatsuhiro, H.; Fujie, T.; Nakanishi, T.; Asahi, T.; Saito, K. Debonding-on-demand adhesives based on photo-reversible cycloaddition reactions. Mater. Adv. 2023, 4 (5), 1289–1296.
- Lin, H.; Wan, X.; Li, Z.; Jiang, X.; Wang, Q.; Yin, J. Photoreversible Resists for UV Nanoimprint Lithography (UV-NIL). ACS Appl. Mater. Interfaces 2010, 2 (7), 2076–2082.
- Alrayyes, A.U.; Low, Z.-X.; Wang, H.; Saito, K. Multi-cycle Reversible Control of gas Permeability in Thin Film Composite Membranes via Efficient UV-Induced Reactions. Chem. Commun. 2021, 57 (27), 3391–3394.
- Alrayyes, A.U.; Hu, Y.; Tabor, R.F.; Wang, H.; Saito, K. Photo-switchable Membranes Constructed from Graphene Oxide/Star-PDMS Nanocomposites for gas Permeation Control. J. Mater. Chem. A 2021, 9 (37), 21167–21174.
- Tabet, A.; Forster, R.A.; Parkins, C.C.; Wu, G.; Scherman, O.A. Modulating Stiffness with Photo-Switchable Supramolecular Hydrogels. Polym. Chem. 2019, 10 (4), 467–472.
- Boga, K.; Patti, A.F.; Warner, J.C.; Simon, G.P.; Saito, K. Sustainable Light-Stimulated Synthesis of Cross-Linked Polymer Microparticles. Macromol. Chem. Phys. 2022, 223 (13), 2100493.
- Wong, C.S.; Hassan, N.I.; Su’ait, M.S.; Serra, M.A.P.; Gonzalez, J.A.M.; Granda, L.A.; Badri, K.H. Photo-activated Self-Healing bio-Based Polyurethanes. Ind. Crops Prod. 2019, 140, 111613.
- Wang, Y.; Liu, Q.; Li, J.; Ling, L.; Zhang, G.; Sun, R.; Wong, C.-P. UV-triggered Self-Healing Polyurethane with Enhanced Stretchability and Elasticity. Polymer 2019, 172, 187–195.
- Cuevas, J.M.; Seoane-Rivero, R.; Navarro, R. Á. Marcos-Fernández, Coumarins into Polyurethanes for Smart and Functional Materials. Polymers. (Basel) 2020, 12 (3), 630.
- Chen, Y.; Geh, J.-L. Copolymers Derived from 7-Acryloyloxy-4-Methylcoumarin and Acrylates: 2. Reversible Photocrosslinking and Photocleavage. Polymer 1996, 37 (20), 4481–4486.
- Li, P.; Chu, Z.; Chen, Y.; Yuan, T.; Yang, Z. One-pot and Solvent-Free Synthesis of Castor oil-Based Polyurethane Acrylate Oligomers for UV-Curable Coatings Applications. Prog. Org. Coat. 2021, 159, 106398.
- Hughes, T.; Simon, G.P.; Saito, K. Light-healable Epoxy Polymer Networks via Anthracene Dimer Scission of Diamine Crosslinker. ACS Appl. Mater. Interfaces 2019, 11 (21), 19429–19443.