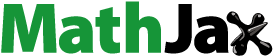
ABSTRACT
This study aimed to synthesize silver nanoparticles (VJ@AgNPs) using Valeriana jatamansi root extract and assess their antibacterial, antioxidant, and antibiofilm properties against Escherichia coli, Staphylococcus aureus, and Streptococcus mutans. Various chemical and physical characterization methods were employed to analyze the synthesized VJ@AgNPs. UV-Visible spectroscopy confirmed the presence of VJ@AgNPs with a peak absorption at 426 nm, while FT-IR results indicated the involvement of phyto-compounds from V. jatamansi in the reduction and stabilization of these nanoparticles. High-resolution transmission electron microscopy revealed well-dispersed spherical nature with an average size of 29.1 ± 2.06 nm, and X-ray diffraction confirmed their crystalline structure. The biosynthesized VJ@AgNPs exhibited significant antibacterial activity against all tested pathogens, with substantial inhibition zones at 50 and 100 μg/mL concentrations. Additionally, VJ@AgNPs displayed potent antibiofilm activity and antioxidant capacity in scavenging assays. Furthermore, these VJ@AgNPs showed promise in photocatalytic degradation, effectively removing 95% of RhB dye within 75 minutes under solar light irradiation, following pseudo-first-order kinetics. This suggests their potential application in wastewater treatment for organic dye removal. The biocompatible and environmentally friendly nature of VJ@AgNPs underscores their potential as therapeutic agents against bacterial infections and oxidative stress-related diseases.
1. Introduction
Worldwide health problems continue to grow worse in the current environment as a result of the proliferation of diseases that are resistant to treatment (Citation1). Research in antimicrobial medications was a turning point in the fight against diseases, but it also led to the emergence of numerous resistant pathogens (Citation2). Staphylococcus aureus and Escherichia coli, two omnipresent bacteria with distinct characteristics, have the ability to cause a variety of illnesses and wreak havoc on human health (Citation3, Citation4). S. aureus, a versatile gram-positive bacterium, colonizes our skin and mucosal surfaces, unleashing a spectrum of infections, from seemingly trivial skin ailments to life-threatening conditions (Citation5). Equipped with a diverse array of virulence factors, S. aureus manipulates host defenses to establish its pathogenic dominance (Citation6–8). S. aureus has become resistant to antibiotics and is increasingly a common source of illnesses in public places. The spread of CA-MRSA among healthy people can happen rapidly and is now an epidemic in the US (Citation9).
Gram-negative bacteria E. coli, occupies diverse environmental niches, including the intestinal tract of warm-blooded humans and other organisms (Citation10). While specific E. coli strains exert positive effects on host health as probiotics, others can manifest as formidable pathogens (Citation11). By employing a range of virulence factors that manipulate cellular processes, this versatile microorganism can cause a broad spectrum of diseases (Citation12). Notably, E. coli O157:H7, a major foodborne pathogen, has garnered global attention due to its ability to induce severe illnesses through the production of potent virulence factors (Citation4, Citation13). Furthermore, urinary tract infections caused by E. coli pose a significant burden, particularly among women, with the rising concern of antibiotic resistance, exacerbating the morbidity associated with these infections (Citation14). This information is based on research conducted by the ‘Global Burden of Disease (Citation15, Citation16). According to the Antimicrobial Resistance Collaborators, 33 bacterial infections were responsible for 77 million fatalities in 2019 (Citation17). S. aureus, E. coli, S. pneumoniae, K. pneumoniae, and P. aeruginosa are the five most prevalent diseases, which together accounted for 54.9% of fatalities (GBD) (Citation17). E. coli resistance to antibiotics is a serious problem that causes death, and increased morbidity for non-hospitalized patients (Citation18). New strategies such as CRISPR-Cas-antimicrobials, phage – therapy, nanobiotics, and measures of probiotics are being used to combat resistant bacteria (Citation19, Citation20).
As mentioned by Petersen et al. (Citation21) and Casamassimo et al. (Citation22), dental caries has a substantial influence on people's economic, social, and functional well-being and affects between 60 and 90 percent of kids and the great majority of adults., S. mutans continues to be a serious health issue (Citation23). A considerable fraction of the population, especially children and teenagers, are affected by dental caries, which is a widespread global problem (Citation24). The GBD Study estimates that in 2017, untreated dental decay affected almost 3.5 billion people globally (Citation25). S. mutans has a greater effect because it may create biofilms on tooth surfaces, which encourage the growth of bacteria that produce acid and cause demineralization of tooth enamel (Citation26). In addition to causing pain and discomfort, dental caries has serious social and economic repercussions (Citation27).
Valeriana jatamansi Jones (Valerianaceae), commonly known as Indian valerian, is a well-known temperate plant used as a raw ingredient in pharmaceutical companies and fragrance sectors (Citation28). It is preferred over current medications for sedation and has been known to enhance the therapeutic benefits of other generic medications. V. jatamansi is a highly valued medicinal plant known for its diverse range of compounds, including valepotriates, flavones, lignans, sesquiterpenoids, phenolic compounds, and terpinoids (Citation29). Due to its antihelmethic, sedative, toxic to neurons, cytotoxic, insecticide activity, antioxidants and antidepressants, and antibacterial properties, it is utilized in both conventional and modern medicine (Citation30–32). Additionally, a specific compound called Jatamanvaltrate (P), an entirely new iridoid ester found in V. jatamansi, has shown promising antitumor properties. It prevents the progression and development of cells derived from humans with breast cancer both in vivo and in vitro, inducing cell cycle slow down, apoptosis, and autophagy (Citation33). Furthermore, extracts from methyl alcohol and aqueous extracts of V. jatamansi rhizome isolates demonstrated inflammatory-reduction action (Citation34). Future research should focus on developing effective compounds for new drugs, addressing neurodegenerative, cardiovascular, and cerebrovascular diseases, and preventing the waste of medicinal resources (Citation35).
One of the rapidly expanding fields in contemporary materials science and technology is nano-biotechnology (Citation36–39). Nanoparticles demonstrated inhibitory effects against bacteria, algae, archaea, fungi, viruses, and larvae (Citation40–42). Nanotechnology encompasses the production and application of materials with sizes extending from 1 to 100 nanometers. Among the nanoparticles, silver-based nanomaterials (AgNPs) have earned substantial attention because of their antimicrobial and disinfectant activities (Citation43). The biogenic reduction of a metal precursor offers an environmentally benign and economical approach, free from chemical contaminants, making it suitable for biological and medical applications (Citation44). Silver nanoparticles, in particular, exhibit broad-spectrum bioactivities, displaying potential as smart nanomedicine with antibacterial, antiviral, antifungal, antiprotozoal (Citation45), larvicidal, Acaricidal (Citation46, Citation47), and anticancer activities (Citation48–50). Furthermore, advancements in nanoscience and technology have revolutionized disease diagnosis, treatment, and prevention (Citation51). In biomedicine, silver nanoparticles take a crucial part, particularly in cancer diagnosis and therapy (Citation49). Precisely, nanobiotechnology, with a focus on silver nanoparticles, holds immense potential for diverse applications (Citation52). They not only possess antimicrobial, antioxidant, and antibiofilm properties but also offer unique properties that can be harnessed for catalytic, optical, electronic, and energy-related advancements. Further research and development in this field are vital for unlocking the full benefits of silver nanoparticles in various sectors (Citation53). In particular, AgNPs are biocompatible, non-toxic, and cost-effective starting materials for generating a significant amount of reactive oxygen species and hot charge carriers that can remove organic pollutants from wastewater (Citation54, Citation55). Therefore, it is important to destroy the organic pollutants/dyes in wastewater completely in the development of a semiconducting photocatalyst-mediated advanced oxidation process (Citation56, Citation57). This photocatalysis system has gained more attraction to the research community to tackle environmental problems in most assured way, prominently eliminating the residual dyes from effluents. Moreover, Green synthesis which used in this study is known for utilizing natural biological systems, and for offering a sustainable approach using naturally sourced materials and low energy processes, which gives a potential solution to traditional methods (Citation58). Biosynthesis of nanoparticles is a cost-effective, environmentally friendly, and easily scaled-up method using microbial enzymes and plant extracts. This method eliminates toxic chemicals and high pressure, energy, and temperature requirements (Citation59).
Nanoparticles composed of metals and their oxide derivatives have been extensively studied for their antioxidative properties, and bio-synthesis approaches using natural compounds have garnered attention for enhancing the antioxidant capabilities of these nanoparticles (Citation60, Citation61). Compared to other metal nanoparticles, silver nanoparticles have shown remarkable antimicrobial activity and antioxidant potential, making them attractive for therapeutic applications in infectious diseases and oxidative stress-related disorders (Citation62, Citation63). Sotiriou and Pratsinis (Citation64) showed a simple, eco-friendly method for synthesizing silver nanoparticles (SNPs) from Plectranthus amboinicus leaf extract. SNPs showed antibacterial and anticancer activity, making them potential biosensors and agents for industrial and biomedical applications. Molecular docking is a computational technique widely used in antibiofilm research to predict how small molecules, such as silver nanoparticles, interact and bind with target proteins involved in biofilm formation (Citation65). Utilizing bioinformatics tools and software like Chem3D Pro, MGL AutoDock Tools, and AutoDock Vina, we conducted molecular docking simulations between V. jatamansi compounds and specific biofilm-associated proteins.
In the present study, several approaches were employed to analyze the biosynthesized VJ@AgNPs. UV-Vis spectrophotometry tracked the synthesis process by observing the surface plasmon resonance peak. Energy – Dispersive X-ray – Spectroscopy (EDX) determined the composition of elements, Fourier – Transform Infrared (FT-IR) spectroscopy identified functional groups, High-Resolution Transmission Electron – Microscopy (HR-TEM), further XRD provided insights into the morphology and size. Zeta size and potential analysis evaluated the ionic charge of the nanoparticles in the solution and their stability. The V. jatamansi root extract mediated biosynthesis of VJ@AgNPs evaluates the antimicrobial, antibiofilm antioxidant, photocatalytic removal of rhodamine blue (RhB) dye, and in silico molecular docking analysis for the first time.
2. Materials and Methods
2.1. Plant collection and preparation of extract
V. jatamansi roots were obtained from Yelagiri Hills, Tirupattur district (12°34’46.5” N 78°38’23.6” E), Tamil Nadu, India. The acquired fresh roots were thoroughly washed with initially tap water, and subsequently distilled water. Afterward, the roots were sliced and shade dried (∼15 days) and finely ground with the help of a stainless-steel blender. 250 mL of milli-Q water was used to soak 100 g of the powdered plant material. Later, the mixture was heated for 30 minutes at 60°C using a water bath. Subsequently, it was cooled down and filtered using Whatman No.1filter paper (pore size 11 μm) (Sigma-Aldrich, Bangalore, India).
2.2. Bio-synthesis of VJ@AgNPs using V. jatamansi root extract
To produce biologically synthesized silver nanoparticles (VJ@AgNPs), different ratios of the V. jatamansi solution and a 1 mM AgNO3 (Sigma-Aldrich, Bangalore, India) solution were combined to determine the optimal conditions for AgNP production. Three ratios were tested: 10 mL of 1 mM AgNO3 with 90 mL – root extract solution, 20 mL of 1 mM AgNO3 with 80 mL – root extract solution, and 50 mL of 1 mM AgNO3 with 50 mL – root extract solution. After combining the solutions, the combinations were left to incubate at room temperature for 30 minutes.
The successful formation of silver nanoparticles was indicated by the development of a brown coloration. Analysis of the solutions was carried out using UV-Visible (UV-Vis) spectrophotometry. The UV-visible spectra of all solutions were systematically monitored, and the solution demonstrating the highest concentration of VJ@AgNPs was chosen for subsequent characterization. For the purification of silver nanoparticles, the resulting filtrate underwent an additional filtration step to eliminate any remaining impurities. Subsequently, the filtered solution was dried using a hot air oven, leading to the formation of a dried extract. This collected dried extract, enriched with the synthesized silver nanoparticles, was meticulously gathered and stored for subsequent utilization and analysis.
2.3. Characterization VJ@AgNPs physical and chemical properties
2.3.1. UV-Vis spectrophotometry analysis
The silver nanoparticles (VJ@AgNPs) were produced using V. jatamansi root aqueous extract and confirmed by UV-visible spectroscopy analysis to primarily define the production of nanoparticles. The UV-visible spectroscopy analysis and characterization of nanoparticles of silver were conducted by a Shimadzu UV-3600 Plus spectrophotometer. The emergence of a dark – brown color indicated the development of VJ@AgNPs, which was supported by surface – plasmon resonance (SPR) and oscillation of free electrons in the nanoparticles (Citation66). Synthesis of VJ@AgNPs was initiated within 10 to 30 minutes and continued vigorously for up to 24 hours. After 24 hours, no further color change occurred, indicating the end of the nanoparticle synthesis.
2.3.2. X – ray Diffraction evaluation (XRD)
The X-ray diffraction investigation of VJ@AgNPs was performed, utilizing the BRUKER USA D8 Advance, Davinci X-ray diffractometer with powder and thin film geometry. The X-ray source was CuKα – radiation (λ = 1.5406 Å), with an operating voltage – 40 kV and a current – 40 mA. The 2θ/θ scanning mode was employed within a range between 30 and 80 degrees, with a step size of 0.0202 degree. The VJ@AgNPs sample, prepared according to the appropriate synthesis method, was placed on the sample collector and analyzed. The collected XRD data was then analyzed to obtain the diffraction pattern and identify the characteristic peaks, providing details regarding the structure of the crystal and orientation of the VJ@AgNPs sample.
2.3.3. Fourier – Transform Infrared Spectroscopy evaluation (FT-IR)
Fourier-transform infrared spectroscopy analysis was utilized to characterize the root extract and its silver nanoparticle complex VJ@AgNPs. The FT-IR spectroscopy procedure was performed by Shimadzu IRTracer-100 FT-IR spectrometer. Sample preparation involved the separate preparation of the root extract and the silver nanoparticle complex. The root extract was obtained using V. jatamansi root extract – reducing agent for silver nanoparticle synthesis, while VJ@AgNPs was formed by mixing the root extract with a silver nanoparticle solution. For analysis, a small amount of each sample was placed on an infrared-transparent material, such as a potassium bromide (KBr) pellet or an attenuated total reflection (ATR) crystal. The samples were subsequently exposed to infrared radiation, allowing for interactions with the molecules present. Spectral analysis of the FT-IR spectra involved the finding of specific wavenumbers and corresponding functional groups within the samples. The positions of characteristic peaks were determined, and the organizational functional groups in the root extract and VJ@AgNPs were recognized based on the known infrared absorption bands associated with various functional groups.
2.3.4. High-Resolution-Transmission Electron Microscopy (HR-TEM)
For HR-TEM analysis, pellets of VJ@AgNPs were made by centrifugation at 10,000 rpm for about 25 minutes. The resulting solid residue containing VJ@AgNPs was later washed 3 times with milliQ water, to eliminate any unattached biological contamination. Overnight, the filtered residue was dried – out in an oven at 70°C. To observe VJ@AgNPs under HR-TEM, a small drop of the dried sample from the synthesized V. jatamansi solution, dispersed in 1 ml of milliQ water, was positioned on a 200-mesh copper-grid that had carbon coating and left to evaporate. HR-TEM measurements were conducted using a JEOL Japan, JEM-2100 Plus instrument functioning at a 200 kV accelerating voltage. Energy – Dispersive X-ray Spectroscopy was conducted to analyze the composition of the synthesized silver nanoparticles (VJ@AgNPs) using V. jatamansi root extract.
2.3.5. Zeta potential analysis
The distribution of size along with particle charge measurements of the produced nanoparticles of silver (VJ@AgNPs) were performed by a Malvern/Nano ZS-90 instrument, which is a Zetasizer Nano Series analyzer. To evaluate the size – distribution, the dynamic light scattering (DLS) analysis was employed. DLS analysis allows for the evaluation of the hydrodynamic diameter of nanoparticles in a sample. The measurements were conducted using the Malvern/Nano ZS-90 instrument, which utilizes the principles of DLS to analyze the intensity of light that is dispersed from the fragment. In addition to size distribution evaluation, the zeta potential of silver nanoparticles was assessed using the instrument. The zeta potential result provided information on the nanoparticles’ stability and surface charge. Their measurements were based on the electrophoretic mobility of the particles in a liquid medium.
2.3.6. Gas – chromatography – mass spectrometry (GC-MS) technique
Gas – chromatography-mass spectrometry was employed to evaluate the sample using a GC-MS-QP2010 Plus instrument equipped with a non-polar column. The instrument parameters were set as follows: a column oven temp of 50°C, an injection temp of 280°C, a split injection mode with a ratio of 10, and a pressure flow control mode with specific settings. The oven temperature program involved a gradual increase from 50°C to 270°C, by 10°C/min, followed by a 3-minute hold.
2.4. Antibacterial activity of synthesized VJ@AgNPs
2.4.1. Bacterial strains
The gram-positive and gram-negative strains of S. mutans (MTCC-890), S. aureus (ATCC25923), and E. coli (ATCC25922) were utilized in this study was from the stock culture maintained in the laboratory on brain heart infusion. The culture was obtained from the Green Lab, Saveetha Dental College and Hospitals, Department of Cariology, Chennai, Tamil Nadu, India. The cultures were kept in brain heart infusion (BHI) that contained 20% – glycerol (v/v) and kept at 80 °C. In advance of the investigation, the concentration of the strains in the investigation was calibrated to a long-term concentration of 0.5 McFarland (1.0 × 108 CFU/mL).
2.4.2. Antibacterial activity of VJ@AgNPs
The Antibacterial potential of nanoparticles produced by V. jatamansi root extract was determined using the agar well diffusion technique. Pathogenic cultures were sub-cultured and stored in the lab. In the antibacterial assays, VJ@AgNPs at concentrations of about 50 and 100 μg/mL were loaded to wells made in Mueller – Hinton agar (MHA) plates. Then the plates were incubated at a temp of 37 °C for 24 hours. Chloramphenicol, an antibiotic, was included as a positive control. The Diameter of the growth inhibition domain had been evaluated using a degree of zone inhibition (Hi-Media, India)
2.4.3. Antibiofilm activity of synthesized VJ@AgNPs
A 96-microtitre well plate was used to conduct a quantitative investigation on biofilm development (Citation67). Freshly grown bacteria were added to Brain Heart Infusion (BHI) broth and the combination was then incubated for 72 hours at 37°C. The cell suspensions were diluted at a ratio of 1:100 in freshly made BHI – broth medium after 24 h. Cells of bacteria that weren't subjected to VJ@AgNPs were regarded as the positive control. VJ@AgNPs were also added to the treated bacterial cultures at a concentration from 25, 50, 75, 100 µg/mL. The sterile BHI broth medium remained empty. Then, 200 µL culture suspensions with and without VJ@AgNPs treatment were added to the sterilized 96-well microplates, which were then incubated for a further 24 h at 37°C. Three replicates of each bacterial suspension were stored. The plates were then rotated to eliminate all the treated and untreated cells in the microtiter wells. Free-floating cells and undesirable material were then removed by washing the plates 3 times in phosphate – buffered saline (PBS, pH 7.2). After 24 h biofilm formation and inhibition were measured as optical density (OD) at 600 nm.
2.5. Antioxidant activity of synthesized VJ@AgNPs
2.5.1. The DPPH assay
VJ@AgNPs’ potential to scavenge the DPPH (2, 2-diphenyl-1-picrylhydrazyl) radicals was assessed using an approach stated by Dua et al. (Citation68) with minor changes. In a nutshell, 1 mL of 0.3 mM DPPH solution was combined with 2 mL of VJ@AgNPs and aqueous extract solution in water at various concentrations of 100, 200, 300, 400, and 500 μg/mL. 1 mL of DPPH and 2 mL of ethanol have been combined to produce the control. After 30 minutes, the wavelength of the consequent substance was detected at 517 nm. Distilled water served as the blank. Using the following formula (1), the proportion of DPPH radical scavenging has been determined.
1)Where Ac (Absorbance of control – ethanol) and As (Absorbance of sample – VJ@AgNPs).
2.5.2. The ABTS assay
In the ABTS studies, ABTS – 2, 2'-azino-bis 3-ethylbenzothiazoline-6-sulfonic acid, radical working solution has been prepared prior to the experiment. Before the test, ABTS radical solution was prepared. After 0.1 mL of each of the varied amounts of aqueous extract and VJ@AgNPs (ranging from 100 to 500 g/mL) had been mixed with ABTS radical working solution (0.9), the absorbance at 734 nm was observed. The equation (2) was employed to determine the scavenging activity of ABTS (Citation69).
(2)
(2)
2.5.3. The Ferric – reducing – antioxidant power assay
The antioxidant property of V. jatamansi and phyto-synthesized VJ@AgNPs was tested using Ferric-Reducing – antioxidant Power (FRAP), which involves changing ferric tripyridyltriazine complex [Fe(III)-TPTZ] to ferrous [Fe(II)-TPTZ] using a reductant (VJ@AgNPs) at low pH levels while recording alteration in its absorbance at 593 nm. The material used for the FRAP experiment was produced daily through the addition of 10 mM TPTZ solution in 40 mM of HCl, 20 mM of FeCl3 6H2O, and 300 mM of acetate – buffer [pH 3.6] in a 10:1:1 ratio and at an operating temperature of 37°C. A total of 1.5 mL of FRAP solution was correctly combined with 50μL of the samples being investigated (VJ@AgNPs or V. jatamansi diluted in DI water) at different amounts (100 to 500 μg/mL), after which incubation in a darkroom at 37°C for 10 min. The beginning absorbance of the blank sample (which included just FRAP reagent) was subtracted from the end absorbance of the main sample (which contained FRAP reagent added to VJ@AgNPs and V. jatamansi) to determine the FRAP reading for each sample. The ferrous sulfate (FeSO4) standard curve was developed using a number of known concentrations (0.125–2 mM), and the results were displayed as Fe (II) ion equivalents (mM).
2.6. Photocatalytic assessments
To evaluate the photocatalytic efficiency of the catalysts under solar light irradiation, RhB dye (10 ppm) was photocatalytically decomposed. For this purpose, 100 mL of the RhB dye solution are mixed with 0.2 g of the photocatalyst in a glass beaker. The reaction solution was constantly agitated at room temperature for one hour in the dark before being exposed to radiation in order to reach the adsorption–desorption equilibrium. 4 mL of the solution combination were regularly removed at various intervals, centrifuged, and examined using a UV-visible spectrophotometer. The dye degradation was found to follow the pseudo-first-order kinetic model and its kinetics are expressed by using equation (3):
(3)
(3) where C0 is the starting concentration of RhB solution following the adsorption–desorption process and Ct is the current RhB concentration under solar light, was computed. In order to check the durability, the photocatalyst was retrieved and reused again for seven cycles.
2.7. Molecular docking analysis
2.7.1. Computational tools and web servers
A well-defined in silico computational study of the selected compounds using various bioinformatics software such as Chem3D pro-12.0, MGL AutoDock Tools, AutoDock Vina, Molegro Molecular Viewer, Discovery Studio Visualizer. Online resources such as RCSB-Protein Data Bank (PDB), PubChem database, SwissADME, pkCSM prediction of toxicity of chemicals, Active site prediction tool, etc., were used in this study (Citation70–73).
2.7.2. ADMET study
The compounds intake, transportation, metabolism, and elimination (ADME) characteristics were carried out by ‘SWISSADME online server’. The Drug likeness property was evaluated by Lipinski’s rule of five. According to it, the molecular weights require to be 500 Daltons or less. Hydrogen – bond donor should be lower than 5, Hydrogen – bond acceptor should be lower than 10 and Partition coefficient (LogP) should be lower than 5. Various toxicity studies including AMES toxicity, Hepatotoxicity, hERG I inhibitor, and Hepatotoxicity were predicted using an online server called pkCSM server (Citation74, Citation75).
2.7.3. Protein and ligand preparation
The drug target proteins were retrieved from the RCSB-PDB database with higher resolution. The protein crystal formation of S. mutans Antigen I/II carboxy-terminus (PDB ID 3QE5) was predicted by X-ray diffraction technique, a resolution of 2.50 Å. This protein involved virulence and biofilm-forming capacity. The retrieved proteins were prepared by removal of co-crystallized ligand and molecules of water using Molegro Molecular Viewer and saved as the PDB file format. The PubChem database's SDF file format was used to download the 3D structures of the Ligand molecules. Chem3D pro 12.0 software was used to reduce energy in these 3D structures containing the ligand molecules and saved as the PDB file format. The Physiochemical properties of the molecules were calculated using ‘SWISSADME online server (Citation76–78).
2.7.4. Molecule docking and site of activity prediction
The target protein's active site was identified by the Supercomputing Facility for Bioinformatics and Computational Biology at IIT Delhi. V. jatamansi root extract 13 compounds and standard drugs were docked to the drug target protein. The molecular docking to find the potential adhesion affinity of naturally isolated substances containing the desired protein. The following processes are involved in the molecular docking of the ligands with the intended protein, Protein – Preparation, Ligand – Preparation, Grids Generation and ligand – docking. The molecular docking of the S. mutans Antigen I/II carboxy-terminus was performed using AutoDock Vina with grid coordinates of Center x (87.081), center y (64.936), and center z (150.061), respectively. The grid’s spacing was set to 50 Å with default docking parameters. The binding interactions between the protein and ligand was visualized and analyzed using Biovia Discovery Studio Visualizer 2021 (Citation79, Citation80).
2.8. Statistical analysis
The results have been provided as a mean ± standard deviation (SD) of three independent tests. To assess for significant differences in the free radical scavenging activities and antioxidant status of the treatment groups, a one-way ANOVA, then a Tukey's test was conducted through the Sigma Plot ver.10.1 programme.
3. Result and discussion
3.1. Production of silver nanoparticles
To assess the impact of phytocompound-induced changes in plant cell metabolism on the capacity of reducing Ag + ions, root extracts of the V. jatamansi were implemented. Within a few minutes of incubation, reaction solutions containing silver nitrate and V. jatamansi root extract underwent a color change (A). The synthetic process of silver nanoparticles is frequently indicated through the presence of a dark – brown color in the reaction mixture; this color was not present in control reactions using silver nitrate without extract. Notably, extracts made from highly concentrated V. jatamansi root extract obviously had higher color intensities (A).
Figure 1. UV–vis spectra of bioreduction of Ag+ ions and the intensity of color duringAgNPs production at (A) color changes after 10 minutes and (B) UV–vis spectra of biosynthesized VJ@AgNPs.
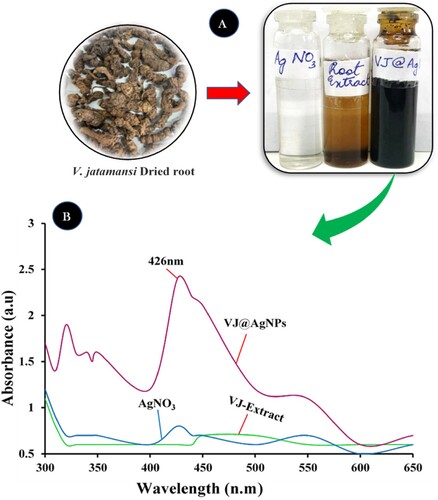
Since extracts made from shoot parts of this medicinal plant have the potential to reduce metal ions, the ability of the root extract to do so was not predicted. According to Iqbal et al. (Citation81), stated that the wavelength of V. jatamansi shoot extract and silver nanoparticle showed the most significant surface plasmon resonance and a peak at 411.0 nm was noted. These present results suggest that the V. jatamansi root extract has the potential to effectively reduce Ag + ions and facilitate the production of spherical nanoparticles of silver with showed strong absorbance peaks at 426 nm (B).
3.2. UV-Vis spectrophotometry analysis of synthesized VJ@AgNPs
Maghsoudy et al. (Citation82) contend that the nanoparticles’ absorbance peak created by Erodium cicutarium is at 230 nm. A spike at 420 nm was visible in the surface plasmon resonance (SPR) of VJ@AgNPs. In the present research, different ratios of root extract and AgNO3 solution were combined, and while stirring continuously, the NP synthesis was observed. Based on the solution's color shift, the first observation of AgNPs synthesis was made. When nanoparticles were produced, a solution's color changed and the resulting densely colored solution (dark brown) revealed that AgNPs had been produced (A). The findings indicating that this color change has occurred are in line with those of Bharathi et al. (Citation83). These researchers observed that the solution used to synthesize AgNPs from Diospyros montana extract was brown in color. The UV-Vis spectrophotometric study was used as a final confirmation of Ag nanoparticle synthesis. Light is absorbed by AgNPs, which have a distinctive absorption peak in the 400–500 nm region. Peak intensity denotes the level of AgNPs. The highest SPR peak at 426.0 nm wavelength across all samples reflected the maximal silver NPs production in a solution with a 5:5 ratio (5 mL AgNO3 and 5 mL root aqueous extract solution) (B). Our UV-Vis spectrophotometric results are consistent with the findings of Sreekanth et al. (Citation84) study, which described the production of AgNPs from Nelumbo nucifera extract and noted its SPR peak at 412.0 nm. In our research, we found that an increase in the extract concentration relative to AgNO3 produces more AgNPs. Umoren et al. (Citation85) made a similar observation and found that a larger extract content increased the likelihood of a stable and well-defined AgNPs synthesis. As a result, the current study showed that the roots of V. jatamansi offer a different method for producing reducing agents involved in the biosynthesis of nanoparticles.
3.3. X-ray diffraction results of synthesized VJ@AgNPs
The XRD evaluation of VJ@AgNPs revealed distinct peaks at the 2θ values of 38°, 44°, 64°, and 77°. These peaks were determined to be silver nanoparticle planes 111, 200, 220, and 311, respectively. Peak intensities were found to be 183, 64, 38, and 43, respectively (). Agreeing to Dharshini et al. (Citation86), using XRD diffraction and a Bragg's angle of 20–80° at 2θ, they assessed the crystalline nature and composition of nanoparticles. The nanoparticles’ peaks, together with their location and relative intensity, were adaptive to the crystalline structure of the silver and showed that nanoparticles have been formed from silver nanoparticles (Citation87).
Similar investigations found that iron and silver nanoparticles produced by green synthesis displayed diffraction peaks at 2θ values about 33° and 38°, respectively (Citation88). The average crystallite size was calculated using the Debye-Scherrer's equation and Scherrer's constant of 0.9, and it was discovered to be 6 nm (Citation89). The obtained XRD pattern confirms the face-centered-cubic crystal formation of the produced VJ@AgNPs. The sharpness of the peaks suggests a well-defined crystal structure.
3.4. Fourier – transform infrared spectroscopy analysis
The FTIR analysis of the aqueous extract of V. jatamansi and its silver nanoparticle complex (VJ@AgNPs) revealed specific functional groups and their corresponding compound classes. In the aqueous extract, observed peak at 3393.4 cm−1 and 3418.8 cm−1 denoted O-H stretching vibrations, characteristic of alcohols. The 2936.0 cm−1 peak stands for C–H stretching vibrations, associated with alkanes assigned to the strong C–H stretching of synthesized silver nanoparticles ( & Table S1). The peak at 1630.7 cm−1 indicated C = O stretching vibrations, characteristic of carbonyl compounds. The occurrence of a peak at 1410.5 cm−1 suggested the appearance of O-H bending vibrations, typically found in carboxylic acids. In addition, according to Awwad et al. (Citation90), functional groups including CC (alkenyl), CN (amide), and N-H (amine) are responsible for the production of nanoparticles. The 1380.8 cm−1 peak in VJ@AgNPs represented C–H stretching vibrations, commonly found in alkyl groups. The appearance of a peak at 1068.8 cm−1 in aqueous extract of V. jatamansi shows the presence of C–N stretching vibrations, associated with amines. Similarly, the appearance of a peak at 1076.8 cm−1 denotes the presence of C–O stretching vibrations, typically found in primary alcohols. The protection from the aggregation of the CO amide band stretching and stabilization of growing protein-amide group encapsulation (Citation91). Similar experiments have been reported by Sharma et al. (Citation92), in which silver nanoparticles displayed functional groups such N-H (amine), – OH (tertiary alcohol), – C–N (amine), and C–H (aromatic). In the VJ@AgNPs complex, the observed peaks were consistent with those in the aqueous extract, indicating the occurrence of similar functional groupings ().
3.5. High-resolution transmission electron microscopy (HR-TEM)
The HRTEM study revealed that VJ@AgNPs were predominantly spherical in its shape. The distribution in size analysis indicated that the nanoparticles exhibited a size below 40 nm. The smallest observed size was measured to be 18.1 ± 0.2 nm, while the largest size observed was 39.0 ± 5 nm (A–C).
Figure 4. (A-C) displays a HRTEM image of a region of the sample, while Figure s (B) and (C) show the size distribution of the nanoparticles. (D) SAED image of VJ@AgNPs.
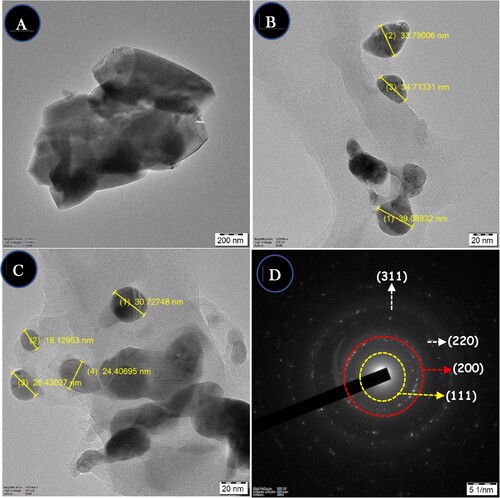
Similar findings were reported by Prodan et al. (Citation93) using TEM images of metal nanoparticles, which displayed a spherical morphology with an average size of 7 ± 0.2 nm. With an average particle size of 50 nm, the silver nanoparticles in the TEM image had a morphology of spherical and round forms. The current results are comparable to those of spherical, biosynthesized silver nanoparticles with diameters between 20 and 40 nm (Citation94). The majority of the AgNPs with sizes up to 200 nm were found to be spherical, with M. balbisiana, A. indica, and O. tenuiflorum extracts being used in their synthesis (Citation95). Additionally, TEM images of green-synthesized silver nanoparticles utilizing Lonicera japonica leaves extract have been distributed, clearly displaying the AgNPs’ natural spherical form (Citation96). The SAED pattern of produced silver nanoparticles is displayed in D. The presence of various crystal planes linked to the production of silver nanoparticles was discovered. The XRD graphs have a strong correlation with the SAED observations. XRD spectra provide assistance for the SAED analysis. The distinct pattern of concentric circular rings indicates that the produced silver nanoparticles are crystalline. These findings demonstrate the successful synthesis of uniform nanospheres with a size distribution that is relatively narrow.
3.6. Energy-dispersive x-ray spectroscopy (EDX) analysis
The EDX analysis of the produced VJ@AgNPs evaluated the occurrence of Ag as the major constituent element. No significant impurities were detected within the nanoparticle sample. The EDX spectrum exhibited a strong peak at 3 keV, which relates to the Ag Kα line. This strong peak denotes the high purity of the bio – synthesized VJ@AgNPs. The elemental composition analysis further revealed that the weight percentage of silver in the nanoparticles was 95.2% ().
Figure 5. EDX analysis showed that the synthesized silver nanoparticles were composed of 100% elemental silver (Ag) (inserted), confirming the purity of the synthesized VJ@AgNPs.
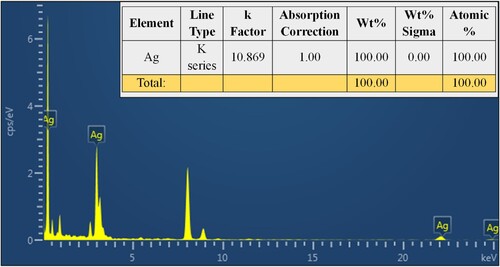
This high percentage indicates a successful synthesis of VJ@AgNPs with a high yield. The k-factor used for silver in the analysis was determined to be 10.869, and the absorption correction factor was set to 1.00. Energy-dispersive spectroscopy study of Ricinus communis-mediated silver nanoparticles revealed their elemental appearance, according to Linima et al. (Citation97). The great purity of the metal nanoparticles was confirmed by the synthesized metal nanoparticles, which revealed that they contained 85% silver and 15% oxygen. Similar to this, Reddy et al. (Citation94) found that the silver content of silver nanoparticles made with Flemingia wightianas extract was 41% (Ag).
3.7. Zeta potential evaluation
The analysis of the formed silver nanoparticles using DLS revealed a mean hydrodynamic diameter of 133.0 nm with a size distribution that is relatively narrow, as denoted by the polydispersity – index (PdI) of 0.247 (A). The zeta potential analysis showed a value of negatively charge (-9.19 mV), indicating a high level of stability due to the strong electrostatic repulsion between particles (B). An increased negative zeta potential value suggests increased stability and prevents the aggregation of nanoparticles. The intensity % of 0.809 indicates a good quality of the result obtained from the measurements. The size istribution report by intensity, as obtained from the DLS analysis, is presented in A,B.
Figure 6. (A) Size distribution reports by intensity and (B) zeta potential of biosynthesized VJ@AgNPs.
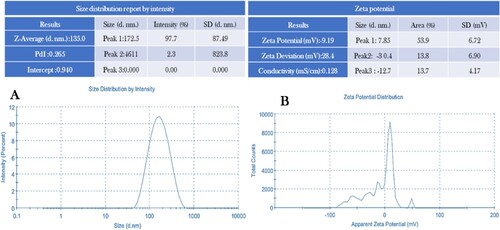
It shows an average diameter of 181.9 nm with a standard deviation of 100.0 nm. This low zeta potential points to the produced Ag-NPs good stability in liquid preparation and is explained by the NPs’ strong electrostatic attraction to one another (Citation98). Additionally, as previously documented, positively charged NPs are less bioactive than negatively charged NPs (Zeta-potential ≤ −30 mV), which are more likely to react with negatively-charged cell membranes (Citation99, Citation100). According to the FTIR results, this negative charge is possibly connected to the numerous negatively charged functional groups (OH, COO, and CH) on the VJ@AgNPs surface. These results further support the effective synthesis of stable silver nanoparticles with a relatively narrow distribution of size.
The disparity between the dynamic light scattering (DLS) results (181 nm) and transmission electron microscopy (TEM) results (40 nm), the observations align with previous studies in the field. The generally smaller but comparable TEM sizes in contrast to the larger DLS sizes are consistent with the notion that TEM provides a number-based size distribution of the physical size, excluding certain factors such as capping agents, while DLS is sensitive to small amounts of larger particles, potentially leading to an overestimation of size due to aggregation (Citation101, Citation102).
3.8. Gas chromatography-mass spectrometry evaluation
The investigation of phyto-compounds derived from V. jatamansi root extract holds significant importance in the encapsulation and production of VJ@AgNPs. To explore the chemical structure of the extract, GC-MS analysis was conducted, resulting in the detection of 106 peaks. Among these peaks, the largest one appeared at a retention time of 4.793 minutes and was identified as styrene, representing 12.13% of the total area. Several other noteworthy compounds were also identified during the analysis. Propanoic acid, 2-oxo-, methyl ester exhibited a peak area of 2.64%, while 3-Buten-2-one had a peak area of 1.33% (Table S2). Additionally, Cyclohexanone was observed with a peak area of 2.99%, and Cyclopentanone, 2-methyl – had a peak area of 1.51%. Other compounds present in smaller quantities included Acetamide, N-(α-methylphenethyl) – (0.19%), Stevioside (0.25%), Heptanal (0.30%), N, N, N’, N’, – Tetramethyl-2-butene-1,4-diamine (0.40%), 3-chloro-N, N-dimethylpropanamide (0.37%), Carbamic acid (0.35%), and Ethanamine, 2-chloro-N, N-dimethyl- (0.20%). V. jatamansi is renowned for the abundance of active ingredients found in both its roots and leaves. This plant species has been shown to contain valepotriates, a group of natural chemicals with medicinal potential (Citation103). Patchoul (24.3%), α-bulnesene (13.8%), isovaleric acid (12.9%), α-guaiene (8.7%), and 3-methylvaleric acid (8.4%) were the main components of V. jatamansi essential oil (Citation104, Citation105). According to Shukla et al. (Citation106), significant components of the leaves of V. jatamansi included luteolin, eugenol, isoeugenol, protocatechuic acid, caffeic acid, quinic acid, protocatechuic acid, valerenic acid, kaempferol-3-O-rutinoside, and protocatechuic acid. These findings are consistent with earlier studies that have identified various bioactive compounds in V. jatamansi.
3.9. Antibacterial activity of synthesized VJ@AgNPs
A maximal inhibition zone was seen in the antibacterial activity of V. jatamansi root aqueous extract and its mediating silver nanoparticles against human pathogenic bacteria. V. jatamansi root aqueous extract 10.8 ± 1.48 mm, 14.7 ± 1.82 mm (E. coli); 8.6 ± 0.84 mm, 10.7 ± 1.42 mm (S. aureus); and 10.2 ± 1.62 mm, 13.4 ± 0.33 mm (S. mutans) at concentration of 50 and 100 μg/mL (A,C & E). The bio-synthesized VJ@AgNPs displayed significantly high inhibitory effects, with inhibition range of 11.2 ± 1.84 mm, 18.3 ± 0.46 mm (E. coli); 13.2 ± 1.68 mm, 15.7 ± 1.32 mm (S. aureus), and 13.6 ± 0.64 mm, 16.2 ± 1.58 mm (S. mutans) at the concentration of 50 and 100 μg/mL ( B, D & F). Positive control (Streptomycin) showed 24.0 ± 0.18, 14.3 ± 1.22 and 19.4 ± 1.02 mm against three strains at μg/mL. Additionally, negatively charged and electrostatically repelling Gram-negative bacteria, the produced AgNPs prevent particle attachment and entry into bacterial cells while simultaneously releasing silver ions (Ag+).
Figure 7. (A–F). Visible clear zone produced by V. jatamansi root aqueous extract and biosynthesized VJ@AgNPs against three species of foodborne pathogen: (A) E. coli, skin infective pathogen (B) S. aureus, and human oral cavity pathogen (C) Streptococcus mutans. Positive control streptomycin was used all the tested pathogens.
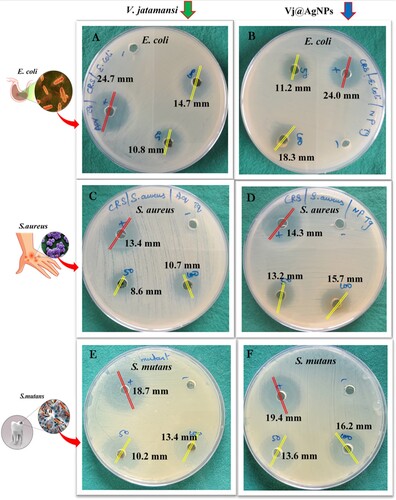
These ions interact with molecules found in the cell wall that include sulfur and phosphorus (Citation107). This contact leads to the upheaval of cell wall and development of small pits on its surface. In a study by Linima et al. (Citation97), R. communis-mediated silver nanoparticles were found to exhibit action against microorganisms that are harmful to humans, with a maximal inhibition zone (mm) for S. typhi (7 ± 0.040), S. aureus (6 ± 0.017), and E. coli (3 ± 0.052). Silver nanoparticles demonstrated strong antibacterial action, and a zone of inhibition was noticed for E. coli (17.00 ± 0.14 mm), Shigella sp. (18.00 ± 0.21 mm), S. typhi (14.00 ± 0.13 mm), and Listeria sp. (13.00 ± 0.29 mm) (Citation92). In comparison to the root extract, the present investigation found that the bio-synthesized AgNPs promised greater antibacterial action against E. coli, S.aureus, and S. mutans. The highly developed surface and small particle size of the nanoparticles enhance their antibacterial action (Citation95). Similarly, a study by (Citation108) exhibited significant antibacterial potential against both Gram-negative (Salmonella typhimurium and Escherichia coli) and Gram-positive (Bacillus cereus and Staphylococcus aureus) microorganisms, from Oxalis corniculate. Nanoparticles interact with the lipids, hydrophobicity, and proteins of the bacterial cell membrane based on their charge and surface chemistry (Citation109). The nanoparticle combined effects of disrupted cell wall formation, increased intracellular pressure, and swelling causes the cell wall to rupture and results in cell lysis (Citation110).
3.10. Antibiofilm activity of synthesized VJ@AgNPs
The current situation necessitates a study on an antibacterial drug that can combat biofilms since these have been identified to be intimately related to a variety of microbial infections (Citation111). In the present study, E. coli, S. aureus, and S. mutans biofilms were used as test subjects for the antibiofilm activity of V. jatamansi root extract and biosynthesized VJ@AgNPs ().
Figure 8. Antibiofilm activity of V. jatamansi root extract and biosynthesized VJ@AgNPs. Biofilm formed by (EC) Escherichia coli, (SA) Staphylococcus aureus, (SM) Streptococcus mutans, (PC) Streptomycin was used as positive control, and (C) control untreated, staining with crystal violet. After 24 h biofilm formation and inhibition were measured as optical density at 600 nm
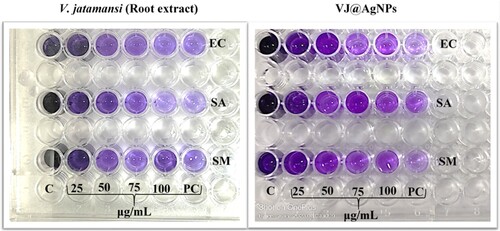
The obtained results showed that the OD values of biofilm formed in the presence of 1/2 MIC of V. jatamansi root extract, VJ@AgNPs, and positive control (Streptomycin) was 0.875, 0.696, and 0.897, respectively, compared to negative control (Without treatment) (3.138) at 600 nm (A-C). One-way ANOVA result exhibited various tested concentration with significant difference between the concentration and tested organisms. F-value: 34.82; P- value: < 0.0001 and R- squared: 0.8970 against (E. coli); F-value: 22.16; P- value: < 0.0001; R- squared: 0.8471 (S. aureus), and F-value: 60.32; P- Value :< 0.0001, R- squared: 0.9378 (S. mutans), respectively. There was no significant difference between the percentages of biofilm formed in the presence of V. jatamansi root extract and biosynthesized VJ@AgNPs all the concentration (P < 0.05).
Figure 9. Inhibitory effects of V. jatamansi and biosynthesized VJ@AgNPs on biofilm formation of (A) Escherichia coli, (B) Staphylococcus aureus, and (C) Streptococcus mutans. The data were presented as the mean ± SD (n = 3) of three independent experiments. * p < 0.05, ** p < 0.0001.

The inhibitory effects were contrasted with an untreated control group and a positive control (streptomycin). After 24 hours, biofilm development and inhibition were assessed using optical density at 600 nm. According to Fatih and Torlak (Citation112), research revealed from the Thymus serpyllum leaves mediated AgNPs had the maximum level of inhibition for the production of S. aureus biofilms at 100 μg/mL, hovering around 73%. The inhibition value at 6.25 μg/mL was discovered to be 44.88 ± 3.47%. According to Akhil et al. (Citation113) and Abishad et al. (Citation114), the increased creation of ROS and suppression of MDR-EAEC exopolysaccharides may be the causes of green synthesized Ag/ZnO NCs antibiofilm activity. Exopolysaccharides are a key component of bacterial biofilms. As a result, the current findings showed that biofilm inhibition is dose-dependent. By interacting with biological elements including nucleic acids and proteins, VJ@AgNPs have the capacity to either directly or indirectly kill off bacteria. VJ@AgNPs precise mechanism of preventing biofilm development is unknown. The VJ@AgNPs highly easy binding and greater penetration into the biofilm structure, which disrupted the lipidome of cell membranes, was the cause of the anti-biofilm activity (Citation115).
3.11. Antioxidant activity of synthesized VJ@AgNPs
The antioxidant property of V. jatamansi root aqueous extract and biosynthesized VJ@AgNPs was assess by ABTS and DPPH scavenging assay. The radical scavenging ability of VJ@AgNPs was concentration reliant and increased as concentrations of VJ@AgNPs from 100 to 500 μg/mL. V. jatamansi root aqueous extract and VJ@AgNPs demonstrated the most scavenging potential of 20 ± 1.5% and 84 ± 2.0% against DPPH radicals and One-way ANOVA result showed F-value: 38.75, P-value: < 0.0001 and R-squared :0.8659 (A).
Figure 10. A. Free radical scavenging activity of different concentrations of aqueous root extract of V. jatamansi and VJ@AgNPs using DPPH scavenging effect. Data represents the results of experiments done in triplicates, n = 3. Values are the mean ± standard deviation of three independent experiments. An asterisk (*, **) represents a significance difference when compared to control values at *p < 0.05. B. ABTS radical scavenging activity. Data represents the results of experiments done in triplicates, n = 3. Values are the mean ± standard deviation of three independent experiments. An asterisk (*, **) represents a significance difference when compared to control values at *p < 0.05. C. Reducing power activity (FRAP). Data represents the results of experiments done in triplicates, n = 3. Values are the mean ± standard deviation of three independent experiments. An asterisk (*, **) represents a significance difference when compared to control values at *p < 0.05.

Similarly, ABTS furthermore demonstrated high radical scavenging abilities with a maximum restriction of 450 ± 10.36 μg/mL for O2 •− and One-way ANOVA result exhibited with F-value: 21.03, P- value: < 0.0001 and R-squared: 0.7781 (B).
VJ@AgNPs expressed good Fe3+ reduction potential in FRAP assay with the least 480 ± 10.5 μg/mL. VJ@AgNPs showed better scavenging properties against DPPH and ABTS + when compared to the aqueous extract and One-way ANOVA results showed at F-value: 25.63, P- value :< 0.0001 and R- squared: 0.8103, respectively (C). Similarly, small significant variation (p > 0.05) was found between VJ@AgNPs and the standard BHT in O2 •− scavenging activities.
Kalim et al. (Citation116) have discovered the roots extracts of V. jatamansi exhibited significant antioxidant property using various assays such as the DPPH, ABTS, hydroxyl radical scavenging, superoxide radical scavenging, and peroxynitrite scavenging. Other research has also mentioned the antioxidant action of V. jatamansi. Thusoo et al. (Citation117) have evaluated the antioxidant action of the essential oil and extracts from methanol, while Pandian et al. (Citation118) have assessed the antioxidant property of the essential oil and the supercritical CO2 fluid extracts. Jugran et al. (Citation119) investigated the antioxidant action of the extracts of the roots and aerial parts of V. jatamansi. Jugran et al. (Citation120) studied the essential oil composition, exhibited the antioxidant capacities. Similar experiments on the DPPH assay were reported by Shejawal et al. (Citation88), in which biologically produced iron and silver nanoparticles showed inhibitions of 18.06 ± 0.60% and 20.83 ± 0.33%, respectively. Additionally, the outcomes were comparable to the biologically produced Morinda lucida leaves extract-mediated silver nanoparticles’ antioxidant properties (Citation121). According to Ulewicz and Wesolowski (Citation122), the higher antioxidant activity of nanoparticles in comparison to the raw extract was noteworthy because antioxidants chemoprotect humans against diseases brought on by stress.
3.12. Photocatalytic degradation of RhB dye
The strong brilliant red hue and sturdy aromatic structure of rhodamine B make it a widely sought-after cationic dye. It is frequently used in the food and textile sectors to provide finished products constant color intensity even when exposed to light. However, conventional and biological techniques of degradation face difficulties due to its strong chemical stability and complicated aromatic structure. In order to solve this problem, we investigated the photocatalytic degradation of Rhodamine B utilizing the promising semiconductor photocatalyst VJ@AgNPs. RhB degrades very little in the absence of a semiconductor, decomposing only 5% following exposure to solar radiation for 75 min. VJ@AgNPs, on the other hand, dramatically increase the degradation efficiency when utilized as a photocatalyst. The photocatalytic performance of VJ@AgNPs was assessed by monitoring the intensity of RhB's maximum absorption peak at 554 nm over time. The photocatalytic performance of VJ@AgNPs is illustrated in . Moreover, C/C0 plots to evaluate the photodegradation process, where C0 represents the initial concentration of RhB, and C represents its concentration at a given time during the degradation reaction. Without any catalyst, the absorbance of RhB decreased by 8%, while in the dark, it decreased by 16% as shown in (a). Remarkably, in the presence of VJ@AgNPs, the absorbance decreased by an impressive 95% after 75 minutes of solar light irradiation.
Figure 11. UV–vis absorption spectra of photodegraded RhB solutions during photo-catalytic experiment at given time intervals.

Figure 12. (a). Effect of solar light irradiation time on the degradation performance, (b) Kinetic trends for photodegradation by the synthesized photocatalysts of RhB.
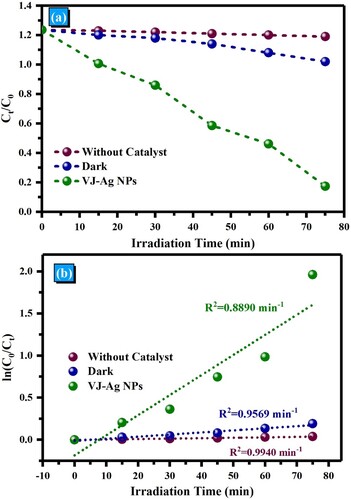
The kinetics of the photocatalytic degradation and found that it followed a pseudo-first-order kinetics model, represented by −ln(C/C0) = kt, where k is the pseudo-first-order rate constant, and t is time. The kinetic curves of all the samples for photocatalytic RhB degradation are demonstrated in (b). The calculated value of k for VJ@AgNPs, and the kinetics plots had a high correlation coefficient (R2) of 0.889. These results demonstrate that VJ@AgNPs exhibit excellent photocatalytic activity, following the pseudo-first-order kinetics model for RhB dye degradation under solar light irradiation. In summary, VJ@AgNPs prove to be efficient in the photocatalytic degradation of Rhodamine B, offering a promising solution for wastewater remediation in industries that utilize RhB as a stable colorant.
In practical applications, one crucial performance criterion for photocatalysts is their ability to maintain efficiency over multiple uses. To assess the recyclability of our VJ@AgNPs, we conducted seven cycles of RhB decomposition (). Between each cycle, the VJ@AgNPs were thoroughly washed with deionized water. Encouragingly, we observed a consistent RhB decomposition rate across all seven cycles, indicating that our VJ@AgNPs can be reused multiple times without significant loss of efficiency.
Therefore, in comparing the photocatalytic degradation proficiency of VJ@AgNPs synthesized using different methods for different pollutants, it is important to specify the experimental conditions used along with the value of the efficiency to ensure a fair comparison () of the reported values.
Table 1. Degradation of RhB by AgNPs obtained from previous studies.
3.13. Molecular docking on synthesized VJ@AgNPs
In the current study, the scores of dockings of the derived compounds ranged from −2.7 to −7.6 kcal/mol. A less docking score generally means a stronger binding-affinity between the ligand and the target protein. Therefore, compounds with more negative docking scores, such as 3-Buten-2-one, Carbamic acid-(2 chloroethylidene) bis diethyl ester, Stevioside, and Styrene, are likely to have stronger interactions with the S. mutans Antigen I/II carboxy-terminus protein. Table S3 represent the molecular docking result of V. jatamansi root extract compounds and standard drugs with S. mutans Antigen I/II carboxy-terminus (PDB ID 3QE5) protein.
3.14. ADME and toxicity study
Based on the results of SWISS ADME, most of the phytochemicals had no infractions against of Lipinski rule of five. Stevioside had three violation of Lipinski rule of five presented in Table S4. According to the outcomes of the pkCSM server the compounds 3-chloro N, N dimethylpropanamide, Carbamic acid-(2 chloroethylidene) bis diethyl ester and Chloramphenicol had AMES toxicity. Table S5 represent the toxicity result of V. jatamansi root extract compounds and the standard drugs. Acetamide, N-(α-methylphenethyl) had the Hepatotoxicity. None of the compounds had hERG I inhibitors.
3.15. Molecular docking study
The thirteen compounds and standard drugs Chloramphenicol and Streptomycin were docked with S. mutans Antigen I/II carboxy-terminus (A–C). We performed molecular docking to determine possible binding affinity of naturally isolated compounds with target protein. The ligand molecules had a high propensity for binding (−2.7 to −7.6 kcal/mol) with the S. mutans Antigen I/II carboxy-terminus protein. Among the thirteen compounds, 3-Buten-2-one, Carbamic acid-(2 chloroethylidene) bis diethyl ester, Stevioside and Styrene had good binding affinity with the target protein (A).
Figure 14. A 3D and 2D interaction of Stevioside with Streptococcus mutans Antigen I/II carboxy-terminus protein. B. 3D and 2D interaction of Styrene with Streptococcus mutans Antigen I/II carboxy-terminus protein. C. 3D and 2D interaction of Acetamide, N-(α-methylphenethyl) with Streptococcus mutans Antigen I/II carboxy-terminus protein.
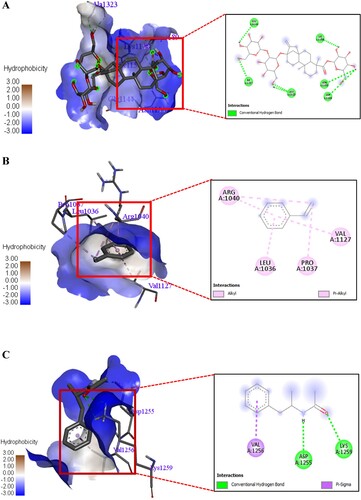
The standard drug Chloramphenicol and Streptomycin had more binding affinity toward the desired protein with docking score of −5.3 and −5.9 kcal/mol. The stevioside had more hydrogen bond interaction with GLY A: 1144, LYS A: 1158, ILE A: 1157, ALA A: 1323, ASN A: 1155 and ASP A: 1189 amino acids of S. mutans Antigen I/II carboxy-terminus protein. The Styrene had Pi-Alkyl interaction with ARG A: 1040, LEU A: 1036, PRO A: 1037 and VAL A: 1127 amino acids of S. mutans Antigen I/II carboxy-terminus protein (B).
The Acetamide, N-(α-methylphenethyl) had hydrogen bond interaction with Alkyl-Pi contact with ASP A: 1255, LYS A: 1259 and Alkyl-Pi contact with VAL A: 1256 1127 amino acids of S. mutans Antigen I/II carboxy-terminus protein (C). The molecular docking study shows that the few phytochemicals had strong interaction with Streptococcus mutans Antigen I/II carboxy-terminus protein.
Additionally, valepotriates, stevioside, α-guaiene, α-bulnesene, luteolin, eugenol, isoeugenol, valerenic acid, and isovaleric acid are found in V. jatamansi and responsible for various bioactivity (Citation103–106). Dereplicating various substances, such as polyphenols, alkaloids, anthraquinones, flavonoids, terpenoids, glycosides, steroids, and reducing sugars, is made easier by using a molecular networking technique. Dereplication of various substances, including stevioside, 3-buten-2-one, styrene, acetamide, N-(α-methylphenethyl), would be the right manner to verify the validity of the molecular networking analysis's findings. In addition, the relationship between the biological activity of extracts and their constituent parts promotes up the discovery of new drugs when biological assays of crude extracts are combined with metabolomics (Citation127–129). The presence of certain polyphenols may be the cause of the root aqueous extract and VJ@AgNPs’ strong antibacterial activity. The comparatively high interaction between stevioside and styrene is thought to be responsible for the root extract's anti-biofilm formation, anti-adhesion, and bactericidal activity. In general, poly hydroxylated compounds were shown to have high interaction values with all investigated proteins, which may be a result of the potent H-bond interaction between ligands and proteins. Additionally, all ligands had considerably different docking score values, proving that affinity is structure-dependent.
The introduced compounds in V. jatamansi extracts could be utilized in upcoming research plans, such as docking analyzes of the components with virulence factors from bacteria, fungi, viruses, and protozoa, as well as proteins that mediate drug resistance. Furthermore, docking studies can be performed on molecules linked to human diseases like cancer or dementia. The application of potential ligands in in-vivo investigations to create efficient medications is the second stage after docking analysis. The dereplicated compounds’ consistency with the chemical structures of the V. jatamansi extracts described in the literature demonstrated the effectiveness of the molecular networking method as a tool for identifying recognized metabolites in plant extracts.
3.16. The study aligns with multiple sustainable development goals (SDGs)
Goal 3: Promoting health by exploring VJ@AgNPs’ antibacterial properties.
Goal 6: Supporting clean water and sanitation through potential wastewater treatment applications.
Goal 9: Encouraging innovation by synthesizing silver nanoparticles from natural extracts.
Goal 12: Advancing sustainable production and consumption via eco-friendly organic dye degradation.
Goal 13: Contributing to climate action through efficient solar-driven dye removal.
Goal 15: Upholding biodiversity and ecosystems through environmentally friendly VJ@AgNPs.
Goal 17: Fostering partnerships for interdisciplinary SDG-focused research and innovation.
4. Conclusion
In conclusion, a comprehensive characterization analysis of synthesized VJ@AgNPs (V. jatamansi root extract capped silver nanoparticles) has provided valuable insights into their structural, chemical, and biological properties. Layout for the making of biogenic VJ@AgNPs, their characterization, and capping evaluation employing different methods together with aqueous root extract from V. jatamansi (Scheme 1). The analysis validated the synthesis's performance of VJ@AgNPs with a well-defined face-centered cubic crystal structure, uniform distribution of size, and high purity of nanoparticles of silver. The appearance of specific functional groups in both the V. jatamansi extract and VJ@AgNPs complex was identified through Fourier-transform – infrared spectroscopy. The High-resolution transmission electron microscopy provided visual evidence of nanoparticles that are spherical with a restricted size distribution. Furthermore, the characterization analysis demonstrated the stability of VJ@AgNPs, as indicated by a strong negative zeta potential value and low polydispersity-index (PdI). The synthesized VJ@AgNPs exhibited notable antibacterial impact on pathogens, including S. aureus, E. coli and S. mutans, with stronger inhibitory effects compared to V. jatamansi alone. The potential binding affinity of certain compounds present in VJ@AgNPs to the target protein of S. mutans suggests their antimicrobial efficacy. The synthesized VJ@AgNPs also demonstrated significant antioxidant activity, as demonstrated by their scavenging potential against DPPH and ABTS radicals, as well as their ability to reduce Fe3+ in the FRAP assay. Antioxidant function of VJ@AgNPs surpassed that of the aqueous extract, indicating the enhanced antioxidant capacity of the synthesized nanoparticles. Overall, these findings highlight the promising potential of VJ@AgNPs as multifunctional agents with antimicrobial and antioxidant properties. Moreover, the as-synthesized VJ@AgNPs exhibited higher efficiency in achieving 95% photocatalytic degradation of RhB within 75 minutes under solar light irradiation. The synthesized nanoparticles hold promise for various applications in domain such as medicine, nanotechnology, preservation of food, and oral healthcare. However, additional study is required to investigate the driving forces behind action, safety profiles, possible clinical outcomes of VJ@AgNPs. In summary, the characterization analysis and biological evaluations of VJ@AgNPs have shed light on their structural, chemical, and biological properties, indicating their potential as antimicrobial and antioxidant agents. These discoveries contribute to the expanding understanding of V. jatamansi and its nanoparticles, laying the foundation for additional research and potential applications across various fields.
Ethical statement
There are no human subjects in this article and informed consent is not applicable.
Supplemental Material
Download MS Word (82.8 KB)Acknowledgements
Pradisha Prem: Conceptualization, methodology, formal analysis, investigation, data curation, writing-original draft preparation, Selvam Naveenkumar: Formal analysis, Software, data collection and draft preparation, Chinnaperumal Kamaraj: Investigation, Conceptualization, Resources, & Supervision, Chinnasamy Ragavendran: Validation, Data curation &Writing – review & editing, Arumugam Priyadharsan: Validation, Data curation &Writing – review & editing, Kumar Manimaran: Formal analysis, Software, Naify S Alharbi: Validation, Software & Resources, Nilesh Rarokar: Formal analysis, Software, Tijo Cherian: Validation, Data curation, Writing – review & editing, Vimal Sugumar: Validation, Software & Resources, Muthu Thiruvengadam: Formal analysis, Software, Vinoth Kumarasamy: Validation, Data curation, Writing – review & editing, Vetriselvan Subramaniyan: Validation, Software & Resources. All authors have read and agreed to the published version of the manuscript. The facilities for characterization studies, including XRD, FT-IR, EDX, and HR-TEM, were generously provided by the SRM Institute of Science and Technology, Kattankulathur, Chennai-603 203, Tamil Nadu, India.
Disclosure statement
No potential conflict of interest was reported by the author(s).
Data availability statement
Data available on request from the corresponding author.
Additional information
Funding
References
- Morris, S.; Cerceo, E. Trends, Epidemiology, and Management of Multi-Drug Resistant Gram-Negative Bacterial Infections in the Hospitalized Setting. Antibiotics 2020, 9, 196.
- Bezza, F.A.; Tichapondwa, S.M.; Chirwa, E.M. Synthesis of Biosurfactant Stabilized Silver Nanoparticles, Characterization and Their Potential Application for Bactericidal Purposes. J. Haz. Mater. 2020, 393, 122319.
- Otto, M. Staphylococcus aureus Toxins. Curr. Opin. Microbiol. 2014, 17, 32–37.
- Kaper, J.B.; Nataro, J.P.; Mobley, H.L. Pathogenic Escherichia coli. Nat. Rev. Microbiol. 2004, 2, 123–140.
- Taylor, T.A.; Unakal, C.G. Staphylococcus aureus Infection; Stat Pearls, 2022, 28722898.
- Cheung, G.Y.C.; Bae, J.; Otto, M. Pathogenicity and Virulence of Staphylococcus aureus. Virulence. 2021, 12, 547–569.
- Zecconi, A.; Scali, F. Staphylococcus aureus Virulence Factors in Evasion from Innate Immune Defences in Human and Animal Diseases. Immunol. Lett. 2013, 150, 12–22.
- Lowy, F.D. Staphylococcus aureus Infections. N. Engl. J. Med. 1998, 339, 520–532.
- Chambers, H.F.; Deleo, F.R. Waves of Resistance: Staphylococcus aureus in the Antibiotic era. Nat. Rev. Microbiol. 2009, 7, 629–641.
- Alm, E. W.; Walk, S. T.; Gordon, D. M., The Niche of Escherichia coli. In S. T. Walk & P. C. H. Feng (Eds.), the Niche of Escherichia coli (Chapter 6). ASM Press 2011, 67–69.
- Fijan, S. Microorganisms With Claimed Probiotic Properties: An Overview of Recent Literature. Int. J. Environ. Res. Public Health 2014, 11, 4745–4767.
- Pakbin, B.; Brück, W.M.; Rossen, J.W.A. Virulence Factors of Enteric Pathogenic Escherichia coli: A Review. Int. J. Mol. Sci. 2021, 22, 9922.
- Lim, J.Y.; Yoon, J.; Hovde, C.J.A. A Brief Overview of Escherichia coli O157:H7 and its Plasmid O157. J. Microbiol. Biotechnol. 2015, 20, 5–14.
- Lee, D.S.; Lee, S.J.; Choe, H.S. Community-Acquired Urinary Tract Infection by Escherichia coli in the Era of Antibiotic Resistance. Biomed Res. Int. 2018, 7656752.
- GBD. Diseases and Injuries Collaborators Global Burden of 369 Diseases and Injuries in 204 Countries and Territories, 1990–2019: A Systematic Analysis for the Global Burden of Disease Study 2019. Lancet 2019, 396, 1204–1222.
- Ikuta, K.S.; Swetschinski, L.R.; Aguilar, G.R.; Sharara, F.; Mestrovic, T.; Gray, A.P.; Weaver, N.D.; Wool, E.E.; Han, C.; Hayoon, A.G.; Aali, A. Global Mortality Associated with 33 Bacterial Pathogens in 2019: A Systematic Analysis for the Global Burden of Disease Study. Lancet 2019, 400, 2221–2248.
- Murray, C.J.L. The Global Burden of Disease Study at 30 Years. Nat. Med. 2022, 28, 2019–2026.
- Peralta, G.; Sanchez, M.B.; Garrido, J.C.; De Benito, I.; Cano, ME;; Martínez-Martínez, L.; Roiz, M.P. Impact of Antibiotic Resistance and of Adequate Empirical Antibiotic Treatment in the Prognosis of Patients with Escherichia coli Bacteraemia. J. Antimicrob. Chemo. 2007, 60, 855–863.
- Elshimy, R. Escherichia coli (E. coli) Resistance Against Last Resort Antibiotics and Novel Approaches to Combat Antibiotic Resistance. Intech Open 2023.
- Theuretzbacher, U.; Outterson, K.; Engel, A.; Karlén, A. The Global Preclinical Antibacterial Pipeline. Nat. Rev. Microbiol. 2020, 18, 275–285.
- Petersen, P.E.; Bourgeois, D.; Ogawa, H.; Estupinan-Day, S.; Ndiaye, C. The Global Burden of Oral Diseases and Risks to Oral Health. Bull. World Health Organiz. 2005, 83, 661–669.
- Casamassimo, P.S.; Thikkurissy, S.; Edelstein, B.L.; Maiorini, E. Beyond the Dmft: The Human and Economic Cost of Early Childhood Caries. J. Amer. Dent. Assoc. 2009, 140, 650–657.
- Kumarasamy, B.; Manipal, S.; Duraisamy, P.; Ahmed, A.; Mohanaganesh, S.; Jeevika, C. Role of Aqueous Extract of Morinda Citrifolia (Indian Noni) Ripe Fruits in Inhibiting Dental Caries-Causing Streptococcus Mutans and Streptococcus Miti. J. Dent. 2014, 11, 703–710.
- Bagramian, R.A.; Garcia-Godoy, F.; Volpe, A.R. The Global Increase in Dental Caries. A Pending Public Health Crisis. Am. J. Dent. 2009, 22, 3–8.
- Bernabe, E.; Marcenes, W. Global, Regional, and National Levels and Trends in Burden of Oral Conditions from 1990 to 2017: A Systematic Analysis for the Global Burden of Disease 2017 Study. J. Dent. Res. 2020, 99, 362–373.
- Marsh, P.D. Dental Plaque as a Microbial Biofilm. Caries Res. 2004, 38, 204–11.
- Peres, M.A.; Macpherson, L.M.; Weyant, R.J.; Daly, B.; Venturelli, R.; Mathur, M.R.; Watt, R.G. Oral Diseases: A Global Public Health Challenge. Lancet 2019, 394, 249–260.
- Dhiman, B.; Sharma, P.; Pal, P.K. Biology, Chemical Diversity, Agronomy, Conservation and Industrial Importance of Valeriana jatamansi: A Natural Sedative. J. Appl. Res. Med. Aromat. Plants 2020, 16, 100243.
- Jugran, A.K.; Rawat, S.; Bhatt, I.D.; Rawal, R.S. Valeriana jatamansi: An Herbaceous Plant with Multiple Medicinal Uses. Phytother. Res. 2019, 33, 482–503.
- Lin, S.; Shen, Y.H.; Li, H.L.; Yang, X.W.; Chen, T.; Lu, L.H.; Huang, Z.Z. Acylated Iridoids with Cytotoxicity from Valeriana jatamansi. J. Nat. Prod. 2009, 72, 650–655.
- Raina, A.P.; Negi, K.S. Essential oil Composition of Valeriana jatamansi Jones from Himalayan Regions of India. Indian J. Pharm. Sci. 2015, 77, 218.
- Mathela, C.C.; Chanotiya, C.C.; Sammal, S.S.; Pant, A.K.; Pandey, S. Compositional Diversity of Terpenoids in the Himalayan Valeriana Genera. Chem. Biodivers. 2005, 2, 1174–1182.
- Yang, B.; Zhu, R.; Tian, S.; Wang, Y.; Lou, S.; Zhao, H. Jatamanvaltrate P Induces Cell Cycle Arrest, Apoptosis, and Autophagy in Human Breast Cancer Cells in Vitro and in Vivo. Biomed. Pharmacothe.r 2017, 89, 1027–1036.
- Subhan, F.; Karim, N.; Ibrar, M. Anti-inflammatory Activity of Methanolic and Aqueous Extracts of Valeriana Wallichii DC Rhizome. Pak. J. Pl. Sci. 2007, 13, 103–108.
- Li, J.; Li, X.; Wang, C.; Zhang, M.; Ye, M.; Wang, Q. The Potential of Valeriana as a Traditional Chinese Medicine: Traditional Clinical Applications, Bioactivities, and Phytochemistry. Front. Pharmacol. 2022, 13, 973138.
- Kamaraj, C.; Ragavendran, C.; Manimaran, K.; Sarvesh, S.; Islam, A.R.; Malafaia, G. Green Synthesis of Silver Nanoparticles from Cassia Auriculata: Targeting Antibacterial, Antioxidant Activity, and Evaluation of Their Possible Effects on Saltwater Microcrustacean, Artemia Nauplii (non-Target Organism). Sci. Tot. Environ. 2023, 861, 160575.
- Srivastava, S.; Bhargava, A.; Srivastava, S.; Bhargava, A. Nanobiotechnology: Present Status and Future Prospects. In Green Nanoparticles. Fut. Nanobiotechnol. 2022, 345–352.
- Kingsley, J.D.; Ranjan, S.; Dasgupta, N.; Saha, P. Nanotechnology for Tissue Engineering: Need, Techniques and Applications. J. Pharm. Res. 2013, 7, 200–204.
- Thakkar, K.N.; Mhatre, S.S.; Parikh, R.Y. Biological Synthesis of Metallic Nanoparticles. Nanomedicine 2010, 6, 257–262.
- Jeevanandam, J.; Krishnan, S.; Hii, Y.S.; Pan, S.; Chan, Y.S.; Acquah, C.; Danquah, M.K.; Rodrigues, J. Synthesis Approach-Dependent Antiviral Properties of Silver Nanoparticles and Nanocomposites. J. Nanostruct. Chem. 2022, 1–23.
- Naveenkumar, S.; Kamaraj, C.; Ragavendran, C.; Vaithiyalingam, M.; Vimal, S.; Marimuthu, K. Gracilaria Corticata Red Seaweed Mediate Biosynthesis of Silver Nanoparticles: Larvicidal, Neurotoxicity, Molecular Docking Analysis and eco-Friendly Approach. Biomass Convers. Biorefinery 2023, 1–23.
- Ragavendran, C.; Kamaraj, C.; Jothimani, K.; Priyadharsan, A.; Kumar, D.A.; Natarajan, D.; Malafaia, G. Eco-friendly Approach for ZnO Nanoparticles Synthesis and Evaluation of its Possible Antimicrobial, Larvicidal and Photocatalytic Applications. Sustain. Mater. Technol. 2023, 36, 00597.
- Das, C.; Paul, S.S.; Saha, A.; Singh, T.; Saha, A.; Im, J.; Biswas, G. Silver-Based Nanomaterials as Therapeutic Agents Against Coronaviruses: A Review. Int. J. Nanomed. 2020, 15, 9301–9315.
- Hussain, F.S.; Abro, N.Q.; Ahmed, N.; Memon, S.Q.; Memon, N. Nano-antivirals: A Comprehensive Review. Front. Nanotechnol. 2020, 4, 1064615.
- Kamaraj, C.; Ragavendran, C.; Satish Kumar, R.C.; Sarvesh, S.; Vetrivel, C.; Vaithiyalingam, M.; Malafaia, G. Synthesize Palladium Nanoparticles from the Macroalgae Sargassum Fusiforme: An eco-Friendly Tool in the Fight Against Plasmodium Falciparum. Sci. Tot. Environ. 2023, 857, 159517.
- Kamaraj, C.; Gandhi, P.R.; Satish Kumar, R.; Balasubramani, G.; Malafaia, G. Biosynthesis and Extrinsic Toxicity of Copper Oxide Nanoparticles Against Cattle Parasites: An Eco-Friendly Approach. Envi. Res 2022, 214, 114009.
- Kamaraj, C.; Karthi, S.; Reegan, A.D.; Balasubramani, G.; Ramkumar, G.; Kalaivani, K.; Zahir, A.A.; Deepak, P.; Senthil-Nathan, S.; Rahman, M.M.; Islam, A.R.M.T. Green Synthesis of Gold Nanoparticles Using Gracilaria Crassa Leaf Extract and Their Ecotoxicological Potential: Issues to be Considered. Env. Res. 2022, 213, 113711.
- Rai, M.; Kon, K.; Ingle, A.; Duran, N.; Galdiero, S.; Galdiero, M. Broad-spectrum Bioactivities of Silver Nanoparticles: The Emerging Trends and Future Prospects. Appl. Microbiol. Biotechnol. 2014, 98, 1951–1961.
- Zhang, X.F.; Liu, Z.G.; Shen, W.; Gurunathan, S. Silver Nanoparticles: Synthesis, Characterization, Properties, Applications, and Therapeutic Approaches. Int. J. Mol. Sci. 2016, 17, 1534.
- Wong, K.K.; Liu, X. Silver Nanoparticles—the Real “Silver Bullet” in Clinical Medicine. Med. Chem. Commun. 2010, 1, 125–131.
- Sahoo, S.K.; Parveen, S.; Panda, J.J. The Present and Future of Nanotechnology in Human Health Care. Nanomedicine 2007, 3, 20–31.
- Prabhu, S.; Poulose, E.K. Silver Nanoparticles: Mechanism of Antimicrobial Action, Synthesis, Medical Applications, and Toxicity Effects. Int. Nano Lett. 2010, 2, 1–10.
- Jasni, A.H.; Ali, A.A.; Sagadevan, S.; Wahid, Z. Silver Nanoparticles in Various New Applications. In Nanoparticles Technology. Intech Open 2021, 1–18.
- Rani, P.; Kumar, V.; Singh, P.P.; Matharu, A.S.; Zhang, W.; Kim, K.H.; Singh, J.; Rawat, M. Highly Stable AgNPs Prepared via a Novel Green Approach for Catalytic and Photocatalytic Removal of Biological and non-Biological Pollutants. Environ. Intern. 2020, 143, 105924.
- Kareem, M.A.; Bello, I.T.; Shittu, H.A.; Awodele, M.K.; Adedokun, O.; Sanusi, Y.K. Green Synthesis of Silver Nanoparticles (AgNPs) for Optical and Photocatalytic Applications: A Review. In IOP Conference Series. Mater. Sci. Eng. 2020, 80, 012020.
- Dua, T.K.; Giri, S.; Nandi, G.; Sahu, R.; Shaw, T.K.; Paul, P. Green Synthesis of Silver Nanoparticles Using Eupatorium Adenophorum Leaf Extract: Characterizations, Antioxidant, Antibacterial and Photocatalytic Activities. Chem. Pap. 2023, 1–10.
- Ghatage, M.M.; Mane, P.A.; Gambhir, R.P.; Parkhe, V.S.; Kamble, P.A.; Lokhande, C.D.; Tiwari, A.P. Green Synthesis of Silver Nanoparticles via Aloe Barbadensis Miller Leaves: Anticancer, Antioxidative, Antimicrobial and Photocatalytic Properties. Appl. Surf. Sci. Adv. 2023, 16, 100426.
- Huston, M.; DeBella, M.; DiBella, M.; Gupta, A. Green Synthesis of Nanomaterials. Nanomaterials 2021, 11, 2130.
- Ramya, M.; Subapriya, M.S. Green Synthesis of Silver Nanoparticles. Int. J. Pharm. Med. Biol. Sci. 2012, 1, 54–61.
- Lopez-Chaves, C.; Soto-Alvaredo, J.; Montes-Bayon, M.; Bettmer, J.; Llopis, J.; Sanchez-Gonzalez, C. Gold Nanoparticles: Distribution, Bioaccumulation and Toxicity. In Vitro and in Vivo Studies. Nanomed. Nanotechnol. Biol. Med. 2018, 14, 1–12.
- Vilas, V.; Philip, D.; Mathew, J. Catalytically and Biologically Active Silver Nanoparticles Synthesized Using Essential oil, Spectrochim. Acta A Mol. Biomol. Spectrosc. 2014, 132, 743–750.
- Sondi, I.; Salopek-Sondi, B. Silver Nanoparticles as Antimicrobial Agent: A Case Study on E. coli as a Model for Gram-Negative Bacteria. J. Colloid Interface Sci. 2004, 275, 177–182.
- Sotiriou, G.A.; Pratsinis, S.E. Antibacterial Activity of Nanosilver Ions and Particles. Environ. Sci. Technol. 2010, 14, 5649–5654.
- Prabakaran, L.; Sathyaraj, W.V.; Yesudhason, B.V.; Subbaraj, G.K.; Atchudan, R. Green Synthesis of Multifunctional Silver Nanoparticles Using Plectranthus Amboinicus for Sensitive Detection of Triethylamine, with Potential In Vitro Antibacterial and Anticancer Activities. Chemosensors 2023, 11, 373.
- Karnjana, K.; Jewboonchu, J.; Niyomtham, N.; Tangngamsakul, P.; Bunluepuech, K.; Goodla, L.; Mordmuang, A. The Potency of Herbal Extracts and its Green Synthesized Nanoparticle Formulation as Antibacterial Agents Against Streptococcus Mutans Associated Biofilms. Biotechnol. Rep. 2023, 37.
- Mulvaney, P. Surface Plasmon Spectroscopy of Nanosized Metal Particles. Langmuir 1996, 12, 788–800.
- Basumatari, M.; Devi, R.R.; Gupta, M.K.; Gupta, S.K.; Raul, P.K.; Chatterjee, S.; Dwivedi, S.K.; Musa balbisianai. Colla Pseudostem Biowaste Mediated Zinc Oxide Nanoparticles: Their Antibiofilm and Antibacterial Potentiality. Curr. Res. Green Sustain. Chem 2021, 4, 100048.
- Dua, T.K.; Giri, S.; Nandi, G. Green Synthesis of Silver Nanoparticles Using Eupatorium Adenophorum Leaf Extract: Characterizations, Antioxidant, Antibacterial and Photocatalytic Activities. Chem. Pap. 2023, 77, 2947–2956.
- Reddy, N.V.; Li, H.; Hou, T.; Bethu, M.S.; Ren, Z.; Zhang, Z. Phytosynthesis of Silver Nanoparticles Using Perilla Frutescens Leaf Extract: Characterization and Evaluation of Antibacterial, Antioxidant, and Anticancer Activities. Int. J. Nanomed. 2021, 16, 15.
- Padmavathy, K.; Sivakumari, K.; Rajesh, S. Exploring Squalene and Rhodoxanthin from Hylocereus Undatus as a Therapeutic Agent for the Treatment of Human Liver Cancer Using Docking Analysis. Chettinad Health City Med. J. 2022, 11, 24–32.
- Kelutur, F.J.; Saptarini, N.M.; Mustarichie, R.; Kurnia, D. Molecular Docking of the Terpenes in Gorgonian Corals to COX-2 and iNOS Enzymes as Anti-Inflammatory. Lett. Drug Des. Discov. 2022, 19, 706–721.
- Shahab, S.; Kaviani, S.; Sheikhi, M.; Alhosseini Almodarresiyeh, H.; Al Saud, S. Dft Calculations and in Silico Study of Chlorogenic, Ellagic and Quisqualic Acids as Potential Inhibitors of SARS-CoV-2 Main Protease. Mpro 2022.
- Moulishankar, A.; Lakshmanan, K. Data on Molecular Docking of Naturally Occurring Flavonoids with Biologically Important Targets. Data. Brief. 2020, 29, 105243.
- Garg, A.; Tadesse, A.; Eswaramoorthy, R. A Four-Component Domino Reaction: An eco-Compatible and Highly Efficient Construction of 1, 8-Naphthyridine Derivatives, There in Silico Molecular Docking, Drug Likeness, ADME, and Toxicity Studies. J. Chem. 2021, 1–16.
- Chen, X.; Li, H.; Tian, L.; Li, Q.; Luo, J.; Zhang, Y. Analysis of the Physicochemical Properties of Acaricides Based on Lipinski's Rule of Five. J. Comput. Biol. 2020, 27, 1397–1406.
- Taghizadeh, M.S.; Niazi, A.; Moghadam, A.; Afsharifar, A. Experimental, Molecular Docking and Molecular Dynamic Studies of Natural Products Targeting Overexpressed Receptors in Breast Cancer. PLoS One 2022, 17.
- Rivera-Quiroga, R.E.; Cardona, N.; Padilla, L.; Rivera, W.; Rocha-Roa, C.; Diaz De Rienzo, M.A.; Martinez, M.C. In Silico Selection and in Vitro Evaluation of new Molecules That Inhibit the Adhesion of Streptococcus Mutans Through Antigen I/II. Int. J. Mol. Sci. 2020, 22, 377.
- Babaeekhou, L.; Ghane, M. Antimicrobial Activity of Ginger on Cariogenic Bacteria: Molecular Networking and Molecular Docking Analyses. J. Biomol. Struct. Dyn 2021, 39, 2164–2175.
- Bhat, S.K.; Purushothaman, K.; Kini, K.R.; Appu Rao, G.R. Design of Mutants of GH11 Xylanase from Bacillus Pumilus for Enhanced Stability by Amino Acid Substitutions in the N-Terminal Region: An in-Silico Analysis. J. Biomol. Struct. Dyn. 2020, 40, 7666–7679.
- El-Hachem, N.; Haibe-Kains, B.; Khalil, A.; Kobeissy, F.H.; Nemer, G. AutoDock and AutoDockTools for Protein-Ligand Docking: Beta-Site Amyloid Precursor Protein Cleaving Enzyme 1 (BACE1) as a Case Study. Neuroproteom. Meth. Protoc. 2017, 391–403.
- Iqbal, M.; Bawazeer, S.; Bakht, J.; Rauf, A.; Shah, M.; Khalil, A.; El-Esawi, M. Green Synthesis of Silver Nanoparticles from Valeriana jatamansi Shoots Extract and its Antimicrobial Activity. Green Process. Synth. 2017, 9, 715–721.
- Maghsoudy, N.; Azar, P.A.; Tehrani, M.S.; Husain, S.W.; Larijani, K. Biosynthesis of Ag and Fe Nanoparticles Using Erodium Cicutarium; Study, Optimization, and Modeling of the Antibacterial Properties Using Response Surface Methodology. Journal of Nanostructure in Chemistry 2019, 9, 203–216.
- Bharathi, D.; Josebin, M.D.; Vasantharaj, S.; Bhuvaneshwari, V. Biosynthesis of Silver Nanoparticles Using Stem Bark Extracts of Diospyros Montana and Their Antioxidant and Antibacterial Activities. J Nanostruct Chem. 2018, 8, 83–92.
- Sreekanth, T.V.M.; Ravikumar, S.; Eom, I.Y. Green Synthesized Silver Nanoparticles Using Nelumbo Nucifera Root Extract for Efficient Protein Binding, Antioxidant and Cytotoxicity Activities. J. Photochem. Photobiol. B Biol. 2014, 141, 100–105.
- Umoren, S.A.; Obot, I.B.; Gasem, Z.M. Green Synthesis and Characterization of Silver Nanoparticles Using red Apple (Malus Domestica) Fruit Extract at Room Temperature. J. Mater. Environ. Sci. 2014, 5, 907–914.
- Dharshini, R.S.; Poonkothai, M.; Srinivasan, P.; Mythili, R.; Syed, A.; Elgorban, A.M.; Selvankumar, T.; Kim, W. Nano-decolorization of Methylene Blue by Phyllanthus Reticulatus Iron Nanoparticles: An eco-Friendly Synthesis and its Antimicrobial, Phytotoxicity Study. Appl. Nanosci. 2021, 1–11.
- Rajeshkumar, S. Citrus Lemon Juice Mediated Preparation of AgNPs/Chitosan-Based Bionanocomposites and its Antimicrobial and Antioxidant Activity. J. Nanomater. 2021, 1–10.
- Shejawal, K.P.; Randive, D.S.; Bhinge, S.D.; Bhutkar, M.A.; Wadkar, G.H.; Jadhav, N.R. Green Synthesis of Silver and Iron Nanoparticles of Isolated Proanthocyanidin: Its Characterization, Antioxidant, Antimicrobial, and Cytotoxic Activities Against COLO320DM and HT29. J. Genetic Eng. Biotechnol. 2020, 18, 1–11.
- Razack, S.A.; Suresh, A.; Sriram, S.; Ramakrishnan, G.; Sadanandham, S.; Veerasamy, M.; Nagalamadaka, R.B.; Sahadevan, R. Green Synthesis of Iron Oxide Nanoparticles Using Hibiscus Rosa-Sinensis for Fortifying Wheat Biscuits. SN Appl. Sci. 2020, 2, 1–9.
- Awwad, A.M.; Salem, N.M.; Abdeen, A.O. Green Synthesis of Silver Nanoparticles Using Carob Leaf Extract and its Antibacterial Activity. Intern. J. Industr. Chem. 2013, 4, 1–6.
- Khan, M.; Ware, P.; Shimpi, N. Synthesis of ZnO Nanoparticles Using Peels of Passiflora Foetida and Study of its Activity as an Efficient Catalyst for the Degradation of Hazardous Organic dye. SN App. Sci. 2021, 3, 1–17.
- Sharma, A.; Sagar, A.; Rana, J.; Rani, R. Green Synthesis of Silver Nanoparticles and its Antibacterial Activity Using Fungus Talaromyces Purpureogenus Isolated from Taxus Baccata Linn. Micro Nano Syst. Lett. 2022, 10, 2.
- Prodan, A.M.; Iconaru, S.L.; Chifiriuc, C.M.; Bleotu, C.; Ciobanu, C.S.; Motelica-Heino, M.; Sizaret, S.; Predoi, D. Magnetic Properties and Biological Activity Evaluation of Iron Oxide Nanoparticles. J. Nanomater. 2013.
- Reddy, N.V.; Satyanarayana, B.M.; Sivasankar, S.; Pragathi, D.; Subbaiah, K.V.; Vijaya, T. Eco-friendly Synthesis of Silver Nanoparticles Using Leaf Extract of Flemingia Wightiana: Spectral Characterization, Antioxidant and Anticancer Activity Studies. SN Appl. Sci. 2020, 2, 1–10.
- Arumugam, N.; Thulasinathan, B.; Pasubathi, R.; Thangavel, K.; Muthuramalingam, J.B.; Arunachalam, A. Biogenesis of Silver Nanoparticles Using Selected Plant Leaf Extract; Characterization and Comparative Analysis of Their Antimicrobial Activity. Nanomed. J. 2017, 4.
- Rajivgandhi, G.N.; Chackaravarthi, G.; Ramachandran, G.; Manoharan, N.; Ragunathan, R.; Siddiqi, M.Z.; Alharbi, N.S.; Khaled, J.M.; Li, W.J. Synthesis of Silver Nanoparticle (Ag NPs) Using Phytochemical Rich Medicinal Plant Lonicera Japonica for Improve the Cytotoxicity Effect in Cancer Cells. J. King Saud Univ. Sci. 2022, 34, 101798.
- Linima, V.K.; Ragunathan, R.; Johney, J. Biogenic Synthesis of Ricinus Communis Mediated Iron and Silver Nanoparticles and its Antibacterial and Antifungal Activity. Heliyon 2023, 9.
- Mat Khalir, W.; Shameli, W.K.A.; Jazayeri, K.; Othman, S.D.; Che Jusoh, N.A.; Hassan, N.W.; M, N. Biosynthesized Silver Nanoparticles by Aqueous Stem Extract of Entada Spiralis and Screening of Their Biomedical Activity. Front. Chem. 2020, 8, 620.
- Wypij, M.; Jędrzejewski, T.; Trzcińska-Wencel, J.; Ostrowski, M.; Rai, M.; Golińska, P. Green Synthesized Silver Nanoparticles: Antibacterial and Anticancer Activities, Biocompatibility, and Analyses of Surface-Attached Proteins. Front. Microbiol. 2021, 12, 632505.
- Auría-Soro, C.; Nesma, T.; Juanes-Velasco, P.; Landeira-Viñuela, A.; Fidalgo-Gomez, H.; Acebes-Fernandez, V.; Gongora, R.; Almendral Parra, M.J.; Manzano-Roman, R.; Fuentes, M. Interactions of Nanoparticles and Biosystems: Microenvironment of Nanoparticles and Biomolecules in Nanomedicine. Nanomaterials 2019, 9, 1365.
- Baalousha, M.; Lead, J. R., Characterization of Natural Aquatic Colloids (<5 nm) by Flow-Field Flow Fractionation and Atomic Force Microscopy. Environ. Sci. Technol. 2007, 41, 1111.
- Domingos, R.F.; Baalousha, M.A.; Ju-Nam, Y.; Reid, M.; Tufenkji, N.; Lead, J.R.; Leppard, G.G.; Wilkinson, K.J. Characterizing Manufactured Nanoparticles in the Environment: Multimethod Determination of Particle Sizes. Environ. Sci. Technol. 2009, 43, 7277–7284.
- Thakur, P.; Sharma, Y.P.; Bhardwaj, C. Phyto-chemical Variation in Gynodioecious Valeriana jatamansi Jones. J. Pharm. Phytochem. 2019, 8, 1576–1582.
- Liu, X.C.; Zhou, L.; Liu, Z.L. Identification of Insecticidal Constituents from the Essential oil of Valeriana jatamansi Jones Against Liposcelis Bostrychophila Badonnel. J. Chem. 2013, 853912.
- Bhatt, I.D.; Dauthal, P.; Rawat, S.; Gaira, K.S.; Jugran, A.; Rawal, R.S.; Dhar, U. Characterization of Essential oil Composition, Phenolic Content, and Antioxidant Properties in Wild and Planted Individuals of Valeriana jatamansi Jones. Sci. Horticul. 2012, 136, 61–68.
- Shukla, V.; Singh, P.; Konwar, R.; Singh, B.; Kumar, B. Phytochemical Analysis of High Value Medicinal Plant Valeriana jatamansi Using LC-MS and It's in-Vitro Anti-Proliferative Screening. Phytomed. Plus 2021, 1, 100025.
- Urnukhsaikhan, E.; Bold, B.E.; Gunbileg, A.; Sukhbaatar, N.; Mishig-Ochir, T. Antibacterial Activity and Characteristics of Silver Nanoparticles Biosynthesized from Carduus Crispus. Sci. Rep. 2021, 11, 21047.
- Perumal, S.; Atchudan, R.; Ramalingam, S.; Edison, T.N.J.I.; Lee, H.M.; Cheong, I.W.; Lee, Y.R. Comparative Investigation on Antibacterial Studies of Oxalis Corniculata and Silver Nanoparticle Stabilized Graphene Surface. J. Mater. Sci. 2022, 57, 11630–11648.
- Linklater, D.P.; Baulin, V.A.; Le Guével, X.; Fleury, J.B.; Hanssen, E.; Nguyen, T.H.P.; Juodkazis, S.; Bryant, G.; Crawford, R.J.; Stoodley, P.; Ivanova, E.P. Antibacterial Action of Nanoparticles by Lethal Stretching of Bacterial Cell Membranes. Adv. Mater. 2020, 32, 2005679.
- Ahmed, R.H.; Mustafa, D.E. Green Synthesis of Silver Nanoparticles Mediated by Traditionally Used Medicinal Plants in Sudan. Int. Nano Lett. 2020, 10, 1–14.
- Khan, M.I.; Behera, S.K.; Paul, P.; Das, B.; Suar, M.; Jayabalan, R.; Fawcett, D.; Poinern, G.E.J.; Tripathy, S.K.; Mishra, A. Biogenic Au@ ZnO Core–Shell Nanocomposites Kill Staphylococcus aureus Without Provoking Nuclear Damage and Cytotoxicity in Mouse Fibroblasts Cells Under Hyperglycemic Condition with Enhanced Wound Healing Proficiency. Med. Microbiol. Immunol. 2019, 208, 609–629.
- Fatih, E.R.C.I.; Torlak, E. Antimicrobial and Antibiofilm Activity of Green Synthesized Silver Nanoparticles by Using Aqueous Leaf Extract of Thymus Serpyllum. Sakarya Univ. J. Sci. 2019, 23, 333–339.
- Akhil, K.; Jayakumar, J.; Gayathri, G.; Khan, S.S. Effect of Various Capping Agents on Photocatalytic, Antibacterial and Antibiofilm Activities of ZnO Nanoparticles. J. Photochem. Photobiol B Biol. 2016, 160, 32–42.
- Abishad, P.; Vergis, J.; Unni, V.; Ram, V.P.; Niveditha, P.; Yasur, J.; Juliet, S.; John, L.; Byrappa, K.; Nambiar, P.; Kurkure, N.V. Green Synthesized Silver Nanoparticles Using Lactobacillus Acidophilus as an Antioxidant, Antimicrobial, and Antibiofilm Agent Against Multi-Drug Resistant Enter Aggregative Escherichia coli. Probio. Antimicro. Prot 2022, 14, 904–914.
- Vanlalveni, C.; Lallianrawna, S.; Biswas, A.; Selvaraj, M.; Changmai, B.; Rokhum, S.L. Green Synthesis of Silver Nanoparticles Using Plant Extracts and Their Antimicrobial Activities: A Review of Recent Literature. RSC Adv. 2021, 11, 2804–2837.
- Kalim, M.D.; Bhattacharya, D.; Banerjee, A.; Chattopadhyay, S. Oxidative DNA Damage Preventive Activity and Antioxidant Potential of Plants Used in Unani System of Medicine. BMC Compl. Altern. Med. 2010, 10, 1–11.
- Thusoo, S.; Gupta, S.; Sudan, R.; Kour, J.; Bhagat, S.; Hussain, R.; Bhagat, M., Antioxidant Activity of Essential oil and Extracts of Valeriana jatamansi Roots. Biomed. Res. Int. 2014, 614187.
- Pandian, D.S.; Nagarajan, N.S. Comparison of Chemical Composition and Antioxidant Potential of Hydrodistilled oil and Supercritical Fluid CO2 Extract of Valeriana Wallichi DC. J. Nat. Prod. Res. 2015, 1, 25–30.
- Jugran, A.K.; Bahukhandi, A.; Dhyani, P.; Bhatt, I.D.; Rawal, R.S.; Nandi, S.K. Impact of Altitudes and Habitats on Valerenic Acid, Total Phenolics, Flavonoids, Tannins, and Antioxidant Activity of Valeriana jatamansi. J. Appl. Biochem. Biotechnol. 2016, 179, 911–926.
- Jugran, A.K.; Rawat, S.; Bhatt, I.D.; Rawal, R.S. Essential oil Composition, Phenolics and Antioxidant Activities of Valeriana jatamansi at Different Phenological Stages. Plant Biosyst. 2021, 155, 891–898.
- Labulo, A.H.; David, O.A.; Terna, A.D. Green Synthesis and Characterization of Silver Nanoparticles Using Morinda Lucida Leaf Extract and Evaluation of its Antioxidant and Antimicrobial Activity. Chem. Pap. 2022, 76, 7313–7325.
- Ulewicz-Magulska, B.; Wesolowski, M. Total Phenolic Contents and Antioxidant Potential of Herbs Used for Medical and Culinary Purposes. Plant Foods Hum. Nutrit. 2019, 74, 61–67.
- Panchal, P.; Meena, P.; Nehra, S.P. A Rapid Green Synthesis of Ag/AgCl- NC Photocatalyst for Environmental Applications. Environ Sci Pollut Res 2021, 28, 3972–3982.
- Wali, L.A.; Alwan, A.M.; Dheyab, A.B.; Hashim, D.A. Excellent Fabrication of Pd-Ag NPs/PSi Photocatalyst Based on Bimetallic Nanoparticles for Improving Methylene Blue Photocatalytic Degradation. Optik. (Stuttg) 2019, 179, 708–717.
- Rani, P.; Kumar, V.; Singh, P.P.; Matharu, A.S.; Zhang, W.; Kim, K.H.; Rawat, M. Highly Stable AgNPs Prepared via a Novel Green Approach for Catalytic and Photocatalytic Removal of Biological and non-Biological Pollutants. Environ. Intern. 2020, 143, 105924.
- Nguyen, T.H.; Hoang, N.H.; Van Tran, C.; Nguyen, P.T.M.; Dang, T.D.; Chung, W.J.; La, D.D. Green Synthesis of a Photocatalyst Ag/TiO2 Nanocomposite Using Cleistocalyx Operculatus Leaf Extract for Degradation of Organic Dyes. Chemosphere 2020, 306, 135474.
- Tawfike, A.F.; Tate, R.; Abbott, G.; Young, L.; Viegelmann, C.; Schumacher, M.; Diederich, M.; Edrada-Ebel, R. Metabolomic Tools to Assess the Chemistry and Bioactivity of Endophytic Aspergillus Strain. Chem. Biodivers. 2017, 14, 1700040.
- Kamal, N.; Viegelmann, C.V.; Clements, C.J.; Edrada-Ebel, R. Metabolomics-guided Isolation of Anti-Trypanosomal Metabolites from the Endophytic Fungus Lasiodiplodia Theobromae. Planta Med. 2017, 234, 565–573.
- Abbas-Mohammadi, M.; Farimani, M.M.; Salehi, P.; Ebrahimi, S.N.; Sonboli, A.; Kelso, C.; Skropeta, D. Acetylcholinesterase-inhibitory Activity of Iranian Plants: Combined HPLC/Bioassay-Guided Fractionation, Molecular Networking and Docking Strategies for the Dereplication of Active Compounds. J. Pharma Biomed. Anal. 2018, 158, 471–479.